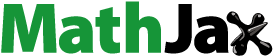
Abstract
We investigated neutron-irradiation-induced point defects in spinel single crystals using a synchrotron VUV-UV source and positron lifetime spectroscopy. Photoexcitation (PE) spectra near 230 nm and their corresponding photoluminescence (PL) spectra at 475 nm were attributed to F-centers. With increasing irradiation temperature and fluence, PE efficiency and PL intensity decreased dramatically. Positron lifetimes (PLT) of neutron-irradiated and non-irradiated samples were measured to identify the cation vacancies. A PLT measurement of 250 ps was obtained in a neutron-irradiated (20 K) sample which is tentatively attributed to an aluminum monovacancy. Decreasing PLT with higher irradiation indicates the formation of oxygen-vacancy complex centers.
Public Interest Statement
We study the effects of radiation on magnesium aluminate spinel, an insulator with potential applications in fusion reactors, by exposing spinel crystals to high-intensity neutron and electron beams at multiple temperatures. Radiation alters the crystals by removing oxygen anions, yielding F-centers, or by removing aluminum cations, yielding V-centers. We then irradiated the crystals with ultraviolet light. Excitation and emission peaks near 230 nm and 475 nm, respectively, were observed, which are characteristic of F-centers. We measured the V-center by exposing the crystals to positrons. Positrons can be absorbed into the cation holes, where their lifetime is greater. We found that moderate radiation yields high F- and V-center densities. However, higher radiation doses, especially at high temperatures, cause these to quench, perhaps due to the formation of more complex centers.
1. Introduction
Magnesium aluminate spinel (MgOnAl
O
) is a radiation hard insulating material. It is a candidate material for various key components in advanced fusion reactor technology such as the inert matrix, window materials for radio frequency heating, insulating materials, and the liquid metal cooling system (Ibarra, Bravo, Lopez, & Garner, Citation2005; Turos, Matzke, Drigo, Sambo, & Falcone, Citation1996). The crystal structure of MgO
nAl
O
consists of a cubic cell with Fd3m symmetry, containing a close-packed array of 32 oxygen atoms with cations in tetrahedral and octahedral interstices (Gritsyna, Afanasyev-Charkin, Kobyakov, & Sickafus, Citation1999). The relatively complex structure, compared to that of its constituent oxides MgO and
-Al
O
, makes the analysis of defects more difficult. Neutron-irradiation can potentially create both anions (F-type centers) and cations (V-type centers) in spinel single crystals.
Studies of irradiation-induced point defects such as F-type centers in MgOnAl
O
were reported for both stoichiometric and non-stoichiometric spinel crystals (Bandyopadhyay & Summers, Citation1985; Gritsyna et al., Citation1999; Gritsyna, Afanasyev-Charkin, Kazarinov, & Sickafus, Citation2004; Summers, White, Lee, & Crawford, Citation1980). The F-center absorption band shifts toward higher energy in non-stoichiometric spinel crystal because of lattice constant compression. This shift in absorption band is consistent with the Mollvo–Ivey relation (Gritsyna et al., Citation2004). Vibronic photoexcitation spectra in fast neutron-irradiated single crystals related to F-type centers at 13 K were reported elsewhere (Rahman et al., Citation2013). The F-center excitation band in neutron-irradiated non-stoichiometric spinel has a high energy tail which falls beyond 6.2 eV or 200 nm and includes the vacuum ultraviolet range. We have used a synchrotron radiation light source to measure the photoexcitation (PE) and photoluminescence (PL) band.
Studies of positron annihilation spectroscopy to evaluate vacancy-type defects in the neutron-irradiated single crystal spinels are very few to our knowledge. However, defect studies in polycrystalline spinel using positron lifetimes have been reported elsewhere (He, Lin, Lu, & Wang, Citation2002; Klym, Ingram, Shpotyuk, Filipecki, & Hadzaman, Citation2007). Positron lifetime measurement is a non-destructive, sensitive and very useful tool to detect vacancy type defects in a wide range of materials. It provides information on the vacancy size and concentrations. As the positron is normally trapped in cation vacancies, it can be used to investigate the V-type centers in spinel single crystals.
In this report, we used synchrotron VUV-UV and positron lifetime spectroscopy to investigate the anion and cation vacancies, respectively, in electron- and fast neutron-irradiated MgOnAl
O
single crystals. The concentration of F-centers decreases significantly due to higher neutron fluence and temperature. Although the mechanisms responsible for the evolution of simple point defects is not yet fully understood, we would like to present our experimental results. We hope that our results may be useful for an eventual understanding of these mechanisms.
2. Experiments
Single crystals of undoped non-stoichiometric magnesium aluminate spinel (MgOnAl
O
) (n = 2) grown using the Czochralski method were obtained from the Furuuchi Chemical Corporation, Japan. The typical sample size was 7
5
1 mm
. The samples were irradiated with reactor neutrons under different conditions. Neutron bombardment was performed using the low-temperature loop (LTL), hydraulic exposure tube (HET) facilities at the Kyoto University Reactor Research Institute (KURRI), and the Japan Materials Testing Reactor (JMTR) facilitiy of the Japan Atomic Energy Agency. Total irradiation doses ranged from 1.3
10
to 1.2
10
n/cm
, which corresponds to 6.9
10
to 6.4
10
displacements per atom (dpa) estimated using an average displacement threshold energy of 52 eV (He & Jung, Citation2000). Table shows the irradiation conditions of the samples. Electron-irradiation was performed at liquid nitrogen temperature (LNT) using the electron linear accelerator (LINAC) in KURRI. The energy of the electron beam was 30 MeV with a fluence of 5.8
10
/cm
at a flux of 1.6
10
/cm
sec .
Photoluminescence (PL) measurements were performed using the vacuum ultra violet (VUV) beamline 4B8 of the Beijing Synchrotron Radiation Facility at the Institute of High Energy Physics, Chinese Academy of Sciences (CAS). The electron energy of the storage ring was 2.5 GeV and the beam current was approximately 160 to 240 mA during the measurement. Photoexcitation and photoluminescence spectra were measured from 13 to 290 K. Photoexcitation spectra were measured from 125 to 350 nm and PL emission spectra were measured from 400 to 700 nm. A Seya-Namioka-type grating monochromator (1200 L/mm) was used for measuring photoexcitation spectra and an ACTON SP308 monochromator (1200 L/mm) was used to measure emission spectra. The spectral resolution of the system is 0.2 nm. The signal was detected by a Hamamatsu H8259-01 photon counting head. The PL spectra were calibrated using the sodium salicylate signal. Atomic force microscopy (AFM) was used to determine the surface morphology of the samples.
Positron lifetime measurements were performed for neutron irradiated and non-irradiated samples at room temperature using a conventional fast-slow spectrometer with a time resolution of 177 ps (full width at half maximum). Two BaF scintillator detectors coupled to photomultiplier tubes were used to record the start and stop signals. A
Na positron source was used. Each spectrum was accumulated to a total of 2
10
counts. Obtained lifetime spectra were decomposed into three lifetime components using the computer program LT: (Kansy, Citation1996).
(1)
(1)
where are the lifetimes and
are the intensities.
Table 1. Irradiation conditions of the single crystal MgOnAl
O
(n = 2)
3. Results
3.1. AFM
Figure shows an AFM image of the surface morphology in unirradiated and neutron-irradiated spinel. Despite the surface roughness due to unevenness of crystal surface, the difference caused by surface damage to the unirradiated and neutron-irradiated samples is clearly visible.
3.2. VUV-UV spectra
Figure 2. VUV-UV photoexcitation (left) and photoluminescence (right) spectra of irradiated and unirradiated (MgOnAl
O
) (n = 2). LINAC (a), LTL(b), HET-20hrs (c), JMTR (d) and unirradiated (e).
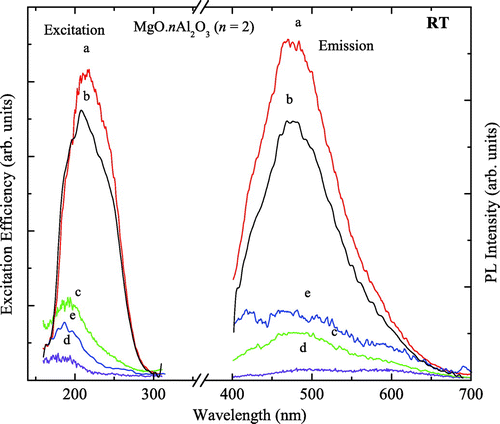
Figure 3. Gaussian curve fitting the experimental photoexcitation spectra in the fast neutron irradiated (LTL) sample.
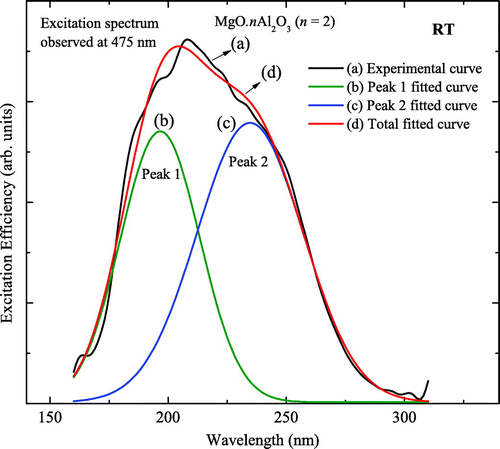
Figure shows the VUV-UV PE (left) and PL (right) spectra of the fast neutron-irradiated and unirradiated spinel samples measured at room temperature. The PE and PL spectra of LINAC-, LTL-, HET-20hrs-, JMTR-irradiated, and unirradiated samples are represented by a, b, c, d, and e, respectively, in Figure . PL spectra were measured under PE into 219 nm. PE spectra were obtained by monitoring luminescence at 476 nm. The peak position of the PE band was found to be near 210 nm in the LINAC- and LTL-irradiated samples whereas the peak positions for the HET-20hrs-, JMTR-irradiated and unirradiated are near 190 nm. The emission peak was found near 475 nm in the LINAC-, LTL-, and HET-20hrs-irradiated samples with a shoulder near at 425 nm. The unirradiated sample shows no obvious emission peak near the 475 nm band except for a shoulder near 425 nm.
Figure shows a Gaussian curve fitting the experimental PE spectra of the LTL-irradiated sample. The black curve represents the experimental curve which is well fitted by the Gaussian curve of total fit (red curve). The PE spectrum of the unirradiated sample (blue curve) is presented for comparison. Peak 1 (green) and peak 2 (blue) were found using the fit multi-peaks method with the OriginPro-8 program. These peak positions were estimated to be 195 and 235 nm, respectively.
Low-temperature PE spectra of spinel irradiated under various conditions are shown in Figure . Electron- and LTL-irradiated samples show vibronic structure whereas HET-20hrs- and JMTR-irradiated do not. Low-temperature PL spectra were also measured (Figure ). Vibronic structure was not observed. However, there was a slight shift to higher energy. A PL band was observed for the LTL- and electron-irradiated samples at 465 nm. This band almost disappears with higher neutron fluence.
3.3. Positron lifetime measurements
Table shows the lifetimes and intensities of long lifetimes in reactor neutron-irradiated MgOnAl
O
(n = 2). Defect-related lifetimes
changed little between the HET-5hrs- and HET-10hrs- irradiated samples.
of the JMTR-irradiated sample is 237 ps with an intensity (I
) of 40.7
. PLT in the LTL-irradiated sample was found to be 250 ps. Table also presents the positron lifetime parameters and trapping modes. The average positron lifetime
, defect-free bulk lifetime
, and positron trapping rate in defects
were calculated using the following equations (Klym et al., Citation2007):
(2)
(2)
The difference will be identified with the defect size (Ghosh, Nambissan, & Bhattacharya, Citation2004) whereas ratio of
describes the nature of the defect (He et al., Citation2002). The size of the vacancy increased in the JMTR-irradiated sample. The ratio
was the same in both the HET-5hrs- and HET-10hrs-irradiated samples whereas there was a small increase in the JMTR-irradiated sample.
Table 2. Positron lifetime parameters and trapping modes
4. Discussion
Figure shows the PE and PL spectra of the samples irradiated under different conditions. Luminescence efficiency greatly decreased in the JMTR- and HET-20hrs-irradiated samples as compared with the LTL- and electron-irradiated samples. PE spectra of the JMTR- and HET-20hrs-irradiated samples shifted toward the short wavelength region and the intensity of the band decreased as well comparing with the LTL- and LINAC-irradiated samples. The emission band near 475 nm (2.6 eV) tentatively corresponds to an F-center (two electrons trapped in an oxygen vacancy) as reported elsewhere (Bandyopadhyay & Summers, Citation1985). This band shifted to 465 nm at low temperature. LTL facility was used to induce simple defects such as F-type centers in the materials (Okada et al., Citation2001). Therefore, LTL-irradiated samples show high luminescence efficiency of the F-centers whereas in higher neutron doses the concentration of F-centers significantly decreased as seen in the JMTR-and HET-20hrs-irradiated samples. A shoulder band near 425 nm was also observed in all the samples which may be due to the presence of a trace ion impurity (Pott & McNicol, Citation1970). Peak separation of the broad PE spectrum was performed using Gaussian multi peak curve fitting (Figure ). The position of peak 1 was estimated to be 195 nm which was also found in the unirradiated sample. Thus, this peak may be due to the presence of a
ion impurity (Gritsyna et al., Citation2004; Summers et al., Citation1980). Peak 2 at 235 nm, which was not observed in the unirradiated sample, is due to irradiation-induced defect and has been attributed to an F-center excitation elsewhere (Summers et al., Citation1980). The intensity of the F-center excitation band increased significantly and shifted toward higher energy at low temperature. The intensity of the F-center excitation band in the JMTR- and HET-20hrs-irradiated samples decreases remarkably in fig. 2 which also indicates lowering the concentrations of the F-centers with higher irradiation doses. Due to low concentrations of F-centers in the JMTR- and HET-20hrs- irradiated samples, transitions of the
ion became the main feature of the excitation spectra near the 195-nm band which match well with that of the unirradiated sample.
To eliminate the effect of impurity in the PE and PL bands, a low-temperature experiment at 13 K was conducted. At low temperature, usually the intensities of defects related to PE and PL spectra significantly increase due to the suppression of thermal phonons. The PE and PL bands were found at 240 and 465 nm, respectively, at 13 K. Even at low temperature, the PE efficiency and PL intensity of the JMTR- and HY-20hrs-irradiated samples did not improve at all. This indicates F-center quenching at higher neutron fluences and temperature. Possibly the evolution of F-centers into F-aggregates or metallic colloid formations occurs after exposure to high neutron-irradiation at high temperature. As a result, the F?center-related PE and PL bands disappear.
From the data of the positron lifetime parameters presented in Table , the as-received crystal can be considered to be defect-free as and
are the same. However, charge-compensating cation vacancies (Gritsyna et al., Citation1999) may be present in the crystal which will not be considered further in this discussion. The positron trapping rate in defects
increased with irradiation doses. The defect-related positron lifetime
increased slightly in the HET-10hrs-irradiated sample compared to the HET-5hrs-irradiated sample. The concentrations of the defects increased significantly. The size of the defects created in HET-5hrs- and HET-10hrs-irradiated samples was same. The nature of the defects was also the same for these samples. In the JMTR-irradiated sample,
increased, however the concentration of the defects
decreased comparing to the HET-10hrs-irradiated sample. In the JMTR-irradiated sample, the defect size (
) increased and the nature of the defects was different from that of the HET-5hrs- and HET-10hrs-irradiated samples. The PLT in the LTL-irradiated sample was found to be 250 ps which corresponds to the lifetime of the Al monovacancy (Seeger & Banhart, Citation1987). The PLT decrease with increasing irradiation fluence and temperature may be associated with the formation of oxygen-vacancy complex centers (Rahman et al., Citation2014).
5. Summary
In this study, evidence of anion and cation vacancies in reactor neutron-irradiated was found in VUV-UV and positron lifetime spectroscopic measurements, respectively. For samples subject to higher irradiation doses, the concentrations of the F- and V-centers decreased significantly which may indicate the formation of more complex centers.
Dedication
This work is dedicated to the memory of Prof. Dr. Masuo Nakagawa (1936–2015).
Acknowledgements
We would like to thank the staffs of KURRI and JMTR for their technical support during irradiation of the samples. We also thank Prof. Dr. Ye Tao and Shuaishuai Sun of the VUV group, IHEP, CAS for their support during the VUV-UV spectroscopic measurement. We would like to express our gratitude to Prof. Dr. Long Wei for his kind support.
Additional information
Funding
Notes on contributors
Abu Zayed Mohammad Saliqur Rahman
A Z M S Rahman and his coauthors have a wide range of research interests in material physics including irradiation-induced defect in materials, electronic & luminescent materials, nuclear reactor materials, positron spectroscopy, optical properties of solid, mechanical properties of materials, energy conversion, and high energy physics. Experimental findings of this article may be useful for an eventual understanding of the mechanism related to evolution of point defects into more complex defect center or colloid formation.
References
- Bandyopadhyay, P. K., & Summers, G. P. (1985). Luminescence and photoconductivity in magnesium aluminum spinel. Physical Review B, 31, 2422–2426.
- Ghosh, S., Nambissan, P. M. G., & Bhattacharya, R. (2004). Positron annihilation and M{\"o}ssbauer spectroscopic studies of In\textsuperscript{3+} substitution effects in bulk and nanocrystalline MgMn\textsubscript{0.1}Fe\textsubscript{1.9}--xInxO\textsubscript{0.1}. Physics Letters A, 325, 301–308.
- Gritsyna, V. T., Afanasyev-Charkin, I. V., Kazarinov, Yu G, & Sickafus, K. E. (2004). Optical transitions in magnesium aluminate spinel crystals of different compositions exposed to irradiation. Nuclear Instruments and Methods in Physics Research Section B, 218, 264–270.
- Gritsyna, V. T., Afanasyev-Charkin, I. V., Kobyakov, V. A., & Sickafus, K. E. (1999). Structure and electronic states of defects in spinel of different compositions MgO‧nAl_{2}O_{3}:Me. Journal of the American Ceramic Society, 82, 3365–3373.
- He, J., Lin, L. B., Lu, T. C., & Wang, P. (2002). Effects of electron- and/or gamma-irradiation upon the optical behavior of transparent MgAl\textsubscript{2}O\textsubscript{4} ceramics: Different color centers induced by electron-beam and γ-ray. Nuclear Instruments and Methods in Physics Research Section B, 191, 596–599.
- He, Z., & Jung, P. (2000). Dimensional changes of Al\textsubscript{2}O\textsubscript{3}, MgO, MgAl\textsubscript{2}O\textsubscript{4}, AlN and Si\textsubscript{3}N\textsubscript{4} by helium implantation. Nuclear Instruments and Methods in Physics Research Section B, 166--167, 165–170.
- Ibarra, A., Bravo, D., Lopez, F. J., & Garner, F. A. (2005). High-dose neutron irradiation of MgAl\textsubscript{2}O\textsubscript{4} spinel: Effects of post-irradiation thermal annealing on EPR and optical absorption. Journal of Nuclear Materials, 336, 156–162.
- Kansy, J. (1996). Microcomputer program for analysis of positron annihilation lifetime spectra. Nuclear Instruments and Methods in Physics Research Section B, 374, 235–244.
- Klym, H., Ingram, A., Shpotyuk, O., Filipecki, J., & Hadzaman, I. (2007). Extended positron-trapping defects in insulating MgAl\textsubscript{2}O\textsubscript{4} spinel-type ceramics. Physica Status Solidi (c), 4, 715–718.
- Okada, M., Kanazawa, S., Nozaki, T., Nakagawa, M., Atobe, K., Kuramoto, E., ... Sano, T. (2001). Improvement of low-temperature irradiation facility at Kyoto University Reactor (KUR). Nuclear Instruments and Methods in Physics Research Section A, 463, 213–219.
- Pott, G. T., & McNicol, B. D. (1970). The phosphorescence of Fe3+ ions in γ-alumina. Chemical Physics Letters, 6, 623–625.
- Rahman, A. Z. M. S., Cao, X., Wei, L., Wang, B., Tao, Y., Xu, Q., & Atobe, K. (2013). Vibronic photoexcitation spectra of irradiated spinel MgO.nAl\textsubscript{2}O\textsubscript{3} (n = 2) at low temperatures. Nuclear Instruments and Methods in Physics Research Section B, 305, 33–36.
- Rahman, A. Z. M. S., Li, Z., Cao, X., Wang, B., Wei, L., Xu, Q., & Atobe, K. (2014). Positron annihilation study of vacancy-type defects in fast-neutron-irradiated MgO‧nAl\textsubscript{2}O\textsubscript{3}. Nuclear Instruments and Methods in Physics Research Section B, 335, 70–73.
- Seeger, A., & Banhart, F. (1987). On the systematics of positron lifetimes in metals. Physica Status Solidi (a), 102, 171–179.
- Summers, G. P., White, G. S., Lee, K. H., & Crawford, J. H. (1980). Radiation damage in MgAl\textsubscript{2}O\textsubscript{4}. Physical Review B, 21, 2578–2584.
- Turos, A., Matzke, Hj., Drigo, A., Sambo, A., & Falcone, R. (1996). Radiation damage in spinel single crystals. Nuclear Instruments and Methods in Physics Research Section B, 113, 261–265.