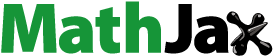
Abstract
CLÆSS is a general-purpose absorption spectroscopy beamline at the ALBA CELLS Spanish synchrotron. Its optical layout is presented here along with its powerful capabilities for collecting absorption spectra with high signal to noise ratio in an unusually wide energy range (2.4–63.2 keV). Continuous energy scanning for quick scans is available, allowing to collect X-ray absorption near edge structure and extended X-ray absorption fine structure spectra in 3–5 and 8–10 min, respectively. The full automatization of the beamline allows performing successive measurements at different conditions without attending to the beamline. The different experimental setups available to users are reported. Examples of XAS measurements are presented, showing the performances of the beamline at different standard conditions.
Public Interest Statement
X-ray absorption spectroscopy is an element-selective local probe to access the structure and electronic properties of matter in all its forms: crystals, glasses or amorphous, liquids, and gases. This is predominantly a synchrotron technique, usually not accessible in research laboratories, due to its intrinsic necessity to tune constantly the incoming photon energy and because it requires a high photon flux. Due to its wide versatility, this technique attracts a large and growing community of users, comprising physicists, material and catalysis scientists, environmentalists, electrochemists, biologists, etc.
CLÆSS is the new general-purpose absorption spectroscopy beamline at the ALBA CELLS Spanish synchrotron which was opened to users from 1 March 2013.
In this paper, we report about its optical layout and available experimental setups, showing its powerful capabilities for collecting quick absorption spectra with high signal-to-noise ratio in an unusually wide energy range (2.4–63.2 keV).
1. Introduction
X-ray absorption spectroscopy (XAS) is an element-selective local probe to access the structure and electronic properties of matter in all its forms: crystals, glasses or amorphous, liquids, and gases (Koningsberger & Prins, Citation1987; Stern & Heald, Citation1983). This is predominantly a synchrotron technique, usually not accessible in research laboratories, due to its intrinsic necessity to tune constantly the incoming photon energy and because it requires a high photon flux. Due to its wide versatility, this technique attracts a large and growing community of users, comprising environmentalists, electrochemists, biologists, material and catalysis scientists, etc. CLÆSS stands for core-level absorption and emission spectroscopies. At ALBA synchrotron, CLÆSS is then a beamline dedicated to XAS. It is installed on a wiggler insertion device in order to access a very wide energy range: from 2.4 to 63.2 keV, with high photon flux (10-10
ph/s) and beam stability (around
15
m) to meet the needs of a large number of researchers. Various fields of research are exploiting this instrument since its opening to users (1st of March 2013): environmental science (Doménech, Ziegler, et al., Citation2016; Gomez-Gonzaleza et al., Citation2016), energy storage (Balachandran et al., Citation2015; Doménech, Romero et al., Citation2016; Domínguez et al., Citation2016; Eslava, Iglesias-Juez, Agostini, dez-Garcá, & Guerrero-Rui, Citation2016; Kylie et al., Citation2016; Masa et al., Citation2014; Olszewski et al., Citation2016), cultural heritage, and catalysis (Bansode et al., Citation2014; Blanco et al., Citation2016; Domínguez et al., Citation2015; Grünert et al., Citation2014a,Citation2014b; Santos et al., Citation2015; Schacht et al., Citation2015; Wezendonk et al., Citation2016), as well as solid state physics (Caramazza, Marini, Simonelli, Dore, & Postorino, Citation2016; Cuartero et al., Citation2015; García-Prieto et al., Citation2015; Lafuerza, García, Subías, Blasco, & Cuartero, Citation2014; Lafuerza et al., Citation2015; Padilla-Pantoja, Herrero-Martín, & Gargiani, Citation2014; Paris et al., Citation2016), chemistry (Stekrovaa et al., Citation2014), and bio-chemistry (Escriche-Tur et al., Citation2015). In this paper, we report the description of the CLÆSS beamline and its potentiality. First of all, we present the beamline layout (from the optical to the experimental hutches) and the available and under-development sample setups. We then report about the available acquisition modes, giving information as well on the already ongoing upgrades. Finally, we show examples of the spectra quality obtainable at CLÆSS.
Figure 1. CLÆSS optical layout. Notes: The inset on the left reports the monochromator energy resolution calculated for the Si(111) and Si(311) reflections. The relative intensity of the Si(111) and Si(311) reflections are reported. On the right are shown the X-ray absorption edges accessible within the CLÆSS energy range (2.4 E
63.2 keV).
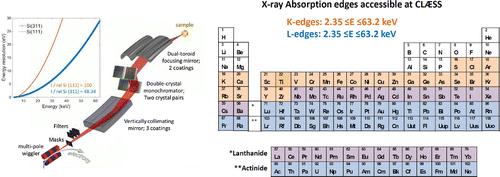
2. Beamline optical layout
Figure shows the optical layout of the CLÆSS beamline, located on a hybrid NdFeB 25 poles 1-m-long wiggler, with maximum on-axis field of 1.74 T and minimum gap of 12.9 mm, to achieve a theoretical maximum energy of 70 keV. Fixed and movable masks made of Glidcop AL-15, having thickness 124 and 200 mm, are placed at 9.6 m and 12.8 m respectively, downstream the wiggler source, to define the correct shape of the X-ray beam and to reduce the thermal heat load. Downstream the wiggler (the fixed masks) the beam divergence is estimated to be 2.21 mrad and
0.34 mrad (
0.94 mrad and
0.27 mrad) in the horizontal and vertical directions, respectively. A set of diamond and carbon filters is located at around 17.6 m from the source to control the thermal bumps on the two first optical elements. The first optical element is a collimating mirror placed at 18.8 m from the source. It has an optically active length of 940, 940, and 1,100 mm and width of 32 mm for the Si, Rh, and Pt coatings, respectively. It is positioned at a variable angle with respect to the direct beam (with a working angular range of 1.3–4.7 mrad). The water-cooled mirror is kept in a liquid Ga bath and is operating under ultra-high vacuum conditions. Its radius of curvature is chosen in order to optimize the energy resolution, beam intensity, and focal properties. The white beam, coming from the collimated mirror, is then monochromatized by means of a liquid nitrogen-cooled double-crystal FMB-Oxford monochromator placed at 20.9 m from the source. The energy range 2.4–63.2 keV can be covered using interchangeable, under ultra-high vacuum, pairs of Si(111) (in general for E
12 keV) and Si(311) (in general for E
12 keV) crystals. The active areas of the first and second crystal (upstream and downstream, respectively) are 60 x 45 and 180 x 40 mm
, respectively. The flux intensity decreases approximately by a factor 2 while the energy resolution improves substantially when moving from the Si(111) to the Si(311) reflection (inset in Figure ). The monochromator angular range is between
3 and 58 degrees, with a resolution of 0.5
rad. To keep the beam exit height fixed during the monochromator rotation, the distance between the two monochromator crystals varies together with the Bragg angle during the energy change or scan. The second crystal angle can be tuned independently to optimize the parallelism of the two crystals and hence the beam intensity. At 24 m from the source the monochromatized beam is focused from 1
10 mm
(standard front end movable masks aperture) down to 100
200
m
(vertical
horizontal) beam size at the sample position (36 m from the source) by means of a toroidal focusing mirror. Two toroidal mirrors with U-bender and different coatings, Rh and Pt for low and high energy, respectively, are exchangeable under ultra-high vacuum. They have an optically active length of 1000 and 1300 mm and width of 32 and 35 mm for the Rh and Pt coating, respectively. The focusing mirror is positioned at a variable angle with respect to the incoming beam, depending on the chosen angle of the collimating mirror and the reqired beam focal properties at the sample position (with a working angular range of around 0.4–4 mrad). Higher harmonic contributions to the selected energies are rejected by the incidence angles and coatings of the collimating and focusing mirrors. All the optical elements are located in the optical hutch. There are no windows in the beam path, and the vacuum presents a gradient from 10
bar, close to the front end, to 10
bar, at the photon shutter isolating the optical from the experimental hutch. We have defined five standard optical configurations to cover the full energy range. To switch between them requires less than one hour (5–60 min), since the procedure has been strongly automatized. Anyway few hours (2–4) should be considered for optics thermalization in order to avoid temperature drifts.
Figure 2. CLÆSS experimental hutch layout (top). ,
, and
are the three ionization chambers allowing the measurements of the photon flux before and after the sample and after the reference. CLÆSS reflectivity curves for different vertical collimating mirror (VCM) angles respect to the white beam (bottom). The photon flux intensity have been measured for the five standard optical configurations with a ring current of 130 mA by means of a calibrating diode located after I
, taking into account as well the absorption of I
.
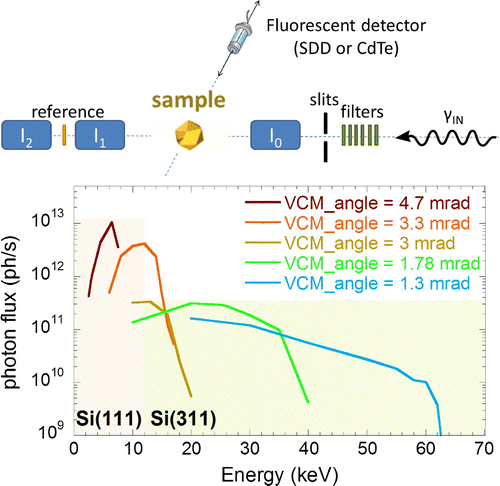
3. Experimental setup
In Figure , a sketch of the experimental hutch downstream the focusing mirror is reported. Here, a very wide motorized table allows following the beam movements in the vertical direction due to the change of configurations necessary to cover the entire energy range (around 50 mm). On this table are located a set of slits and an array of filters to control the beam intensity at the sample position. A series of three ionization chambers, placed after the slits on the motorized table, permits to measure the photon flux before (I ) and after (I
) the sample and a reference (I
). At the entrance of I
is located the first window of the beamline since the ring, a kapton foil of 13
m, to access the lowest energies. The ionization chambers, isolated by one entrance and exit 13
m kapton foil windows, are filled with optimal He, N
, Ar, Kr, and Xe gas mixtures at a standard pressure of 1 bar, and are operating at a field of 3.76 kV per 15 mm of gap between the electrodes at 20 cm of length. Two independent signals are coming from the two isolated parts of the collector electrode at 0 potential, that allow for controlling the vertical position or the intensity of the beam by means of a feedback on a piezo which independently controls the angle of the second monochromator crystal (MOCO feedback control, developed at ESRF). Typically, the beam stability is
15
m (
5
m) in the vertical (horizontal) direction during an energy scan of 1.2 keV. The current signals from the ionization chambers are collected, amplified and converted to output voltage by the ALBA Electrometer (Lidon-Simon et al., Citation2011). The signal in finally digitized and read by an ADLink-2006 card. The typical incoming photon flux as a function of energy is shown in Figure . It was determined by means of a calibrated photon diode, placed just after the I
chamber. Values were extracted for a standard beam size (1
10 mm
movable masks) and ring current of around 130 mA. The absorption of I
was taken into account.
For absorption measurements in fluorescence mode a solid-state detector (Amptek silicon drift (XR-100SDD) or CdTe (XR-100T-CdTe) single channel detector, for low (E 20 keV) or high (E
20 keV) energy, respectively) placed at 90 degrees with respect to the incoming beam is used. A PX5 digital pulse processor is used to collect the detector signal. The fluorescence detector is mounted on a motorized stage allowing the variation of the sample–detector distance from around 30 up to 110 mm. At the moment, from 1 to 6 hours are required to set the fluorescence detector in order to reach the best possible data quality, depending on the sample under investigation.
Although not the objective of this particular paper, it is worthwhile to mention the presence of a unique X-ray emission spectrometer (CLEAR) between I or I
and the sample (urlhttps://www.cells.es/en/media/news/x-ray-emission-spectroscopy-available). The CLEAR spectrometer is still under commissioning but it is expected to be operational from the beginning of 2017, in the energy range 6.4–12.5 keV. XAS and X-ray emission spectroscopy (XES) are the two faces of the same coin. In XAS the samples under investigation are illuminated with photons of selected energies resulting in electronic transitions from occupied to empty levels or to the continuum, and the evolution of the absorption coefficient as a function of energy gives access to the local electronic and structural properties around the absorber. On the other hand, in XES the samples under investigation are illuminated with photons of energy well above a selected absorption edge, and the decay, i.e. the fluorescence signal following the creation of the core-hole, is studied as a function of the emitted energy to access complementary information on the local electronic, magnetic, and structural properties. The CLEAR spectrometer allows to energy-analyze the emitted fluorescence and to resolve with good energy resolution the signals from the different de-excitation channels. Last but not least, thanks to the combined use of a Mythen unidimensional detector and a silicon diced analyzer, it is possible to acquire a spectrum on a single-shot basis. In practice, this enormously boosts the scientific possibilities of the CLÆSS beamline since it will give access to all the complementary information obtainable by investigating the atomic emission lines and, the rather featureless XAS spectra, obtained with the conventional energy integrated mode, become spectra with fine structure containing key information on electronic levels and magnetic properties. Details on the instrument will be published soon.
Figure 3. A picture of the standard sample setup in the CLÆSS experimental hutch (left). The path of the X-ray beam is indicated together with the sample insertion apertures for the different standard setups. Pictures and characteristics of the different standard and no-standard available setups with the sketch of the ones currently under developments (right).
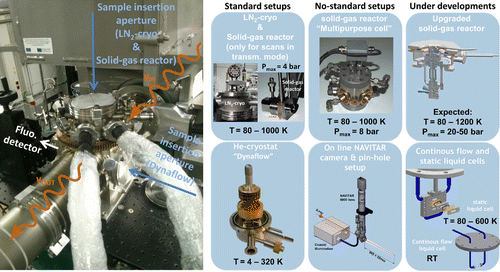
4. Sample environments
Figure shows the setups currently in use at CLÆSS. All the standard sample environments are working in vacuum (10bar), can be subjected to motorized rotation with respect to the incoming beam by means of a home-designed and built rotary feedthrough, and are quickly interchangeable in the experimental hutch at 36 m from the source. A motorized stage allows for sample alignment in the vertical and horizontal directions, perpendicular to the X-ray beam in a range of about
1.5 and
1 cm, respectively.
The most exploited standard setup is the liquid nitrogen cryostat (LN-cryo), that allows fully automatized X-ray absorption measurements in both transmission and fluorescence modes between 80 and 1000 K. Below 600 K spectra are measurable as well in total electron yield mode to access to higher surface sensitivity. The recently installed standard liquid helium cryostat (van der Linden et al., Citationxxxx) extends the temperature range towards lower temperatures. It operates automatically between 5 and 325 K in both transmission and fluorescence modes.
A standard solid–gas reactor allows in situ measurements, i.e. in gas environments, up to a gas pressure of 4 bar, only in transmission mode, and in a temperature range from 80 to 1000 K. Also, in this case the temperature is controlled remotely. A second special cell (multipurpose cell) for the in situ study of catalytic materials has been developed in collaboration with the Instituto de Tecnologia Quimica (ITQ-UPV). This gas–solid reactor is not integrated in the standard beamline setup and it is not operating in vacuum. It allows for measurements both in transmission and fluorescence modes in a temperature range from room temperature up to 1,000 K and up to a maximum gas pressure of 8 bar.
An online camera allows visualization of the beam with respect to the sample. It is not integrated in the standard setup and it works in air. It is possible to mount a pinhole on its motorized support to further reduce the beam size (optimal focus 100 200
m
). For example, the beam can be reduced to approximately 50
50
m
with 70
m diameter pinhole placed at 5 cm upstream the sample, with the focus optimized between the pinhole and the sample. In this case, it is necessary as well to work in air and the intensity of the beam before the sample is monitored by the combination of a scatter and an overhead diode located between the pinhole and the sample.
A new standard gas–solid reactor is under development and is expected to be operational in 2017. The accessible temperature range will be extended between the liquid nitrogen temperature (80 K) up to 1,200 K, and the gas pressure range up to 20–50 bar. Not only the temperature will be controlled remotely but also it will be possible to switch automatically between transmission and fluorescence modes, something that is not possible at present with the multipurpose cell. There will be the possibility to simultaneously mount two samples in the same reaction conditions and to switch between them automatically.
Additionally, two standard liquid setups are under development and are expected to be operational as well in 2017. The first one will work in continuous flow at room temperature, while the second one will be a static liquid cell of variable thickness, compatible with the LN-cryo.
Figure 4. XANES (a and b) and weighted k EXAFS (c and d) spectra corresponding to the S (2.5 keV), Fe (7.1 keV), Mo (20.0 keV), Te (31.8 keV), La (38.9 keV), and Yb (61.4 keV) K-edges collected at CLÆSS on ZnS powder, Li
(NH
)
Fe
Se
, MoSe
, and MoTe
single crystals, and La
WO
and Yb
O
pellet samples, respectively. The S, La, and Yb K-edge spectra are acquired in transmission mode at room temperature. Instead the Fe, Mo, and Te K-edge spectra, measured in fluorescence (beam polarization // c-axis) and transmission mode, respectively, have been acquired at 80K in the LN
-cryo standard setup. Finally, the reported Fe K-edge spectra collected in transmission mode (beam polarization // a,b-plane) have been measured at 10 K in the Dynaflow cryostat. The noise level of the EXAFS spectra, calculated using jefs program, is in 10
for all the reported spectra.
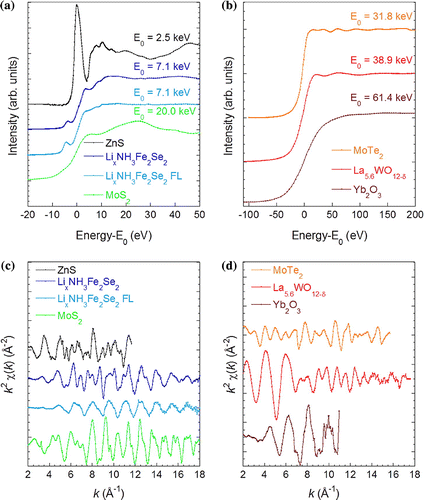
5. Acquisition modes and results
At CLÆSS, spectra can be acquired in either step or continuous mode. In step mode, the acquisition is performed in static mode, as soon as all the motors have reached the desired position. In continuous mode, we are measuring while the monochromator is moving at a constant speed, defined by the two extreme points of the scan and the full acquisition time of the spectra. Since the dead time due to motor movements in step mode is around 2 seconds per point, in general the continuous mode is used both for experiments in transmission and fluorescence mode, with a difference in the number of repeats necessary to reach a good signal-to-noise ratio. We are developing an optimized continuous scan that will allow to change the monochromator speed during the measurements in order to differently weight the different parts of a spectra. Typically, a full EXAFS (XANES) spectra takes around 8–15 min (3–6 min). While in transmission mode at least three repeats should be foreseen to check for reproducibility, in fluorescence mode at least 12–40 (3–15) repeats are necessary to access a good signal-to-noise ratio in the EXAFS (XANES) region, depending on the sample. It results that working in fluorescence mode is, at the moment, very time consuming. Moreover, in fluorescence mode we are often measuring the emission line of highly diluted elements, and the signal coming from the single channel detector appears not enough to reasonably access less than few tens of ppm of concentration. To increase the efficiency and the sensitivity of the CLÆSS fluorescence experiments a multi-channel SDD has been procured and we expect to have it available in 2017. In Figure , few representative XANES and EXAFS spectra are reported for the S (2.5 keV), Fe (7.1 keV), Mo (20.0 keV), Te (31.8 keV), La (38.9 keV), and Yb (61.4 keV) K-edges, collected at CLÆSS on ZnS powder, Li(NH
)
Fe
Se
and MoSe
, and MoTe
single crystals, and La
WO
and Yb
O
pellet samples, respectively. While the S, La, and Yb K-edge spectra were acquired in transmission mode at RT, the Fe, Te, and Mo K-edge spectra measured in fluorescence (beam polarization // c-axis) and transmission mode, respectively, were acquired at 80 K, all of them in the LN
-cryo standard setup. Instead the reported Fe K-edge spectra collected in transmission mode (beam polarization // a,b-plane) have been measured at 10 K in the Dynaflow cryostat. The noise level has been estimated in 10
for all the absorption spectra reported using jefs program, an automatic subroutine for EXAFS evaluation inside GNXAS package (Filipponi & Di Cicco, Filipponi), taking into account the different spectra integration time. It confirms the potentiality of this facility in the full energy range independent of the exploited sample setup. Further examples of results collected at CLÆSS are reported in the literature (Balachandran et al., Citation2015; Bansode et al., Citation2014; Blanco et al., Citation2016; Caramazza et al., Citation2016; Cuartero et al., Citation2015; Doménech, Ziegler et al., Citation2016; Doménech, Romero et al., Citation2016; Domínguez et al., Citation2016; Domínguez et al., Citation2015; Escriche-Tur et al., Citation2015; Eslava et al., Citation2016; García-Prieto et al., Citation2015; Grünert et al., Citation2014a,Citation2014b; Gomez-Gonzaleza et al., Citation2016; Kylie et al., Citation2016; Lafuerza et al., Citation2014; Lafuerza et al., Citation2015; Masa et al., Citation2014; Olszewski et al., Citation2016; Padilla-Pantoja et al., Citation2014; Paris et al., Citation2016; Santos et al., Citation2015; Schacht et al., Citation2015; Stekrovaa et al., Citation2014; Wezendonk et al., Citation2016).
6. Conclusions
In summary, the optical layout and experimental setups available at the CLÆSS beamline at ALBA are described in this paper along with a few examples of X-ray absorption spectra collected in the different standard conditions at different energies. We report about the powerful CLÆSS capabilities for collecting quick absorption spectra with high signal-to-noise ratio in an unusually wide energy range (2.4–63.2 keV). The CLÆSS efficiency is enhanced by the full automatization of the beamline and of the experimental setups. The current ongoing upgrades are discussed as well.
Acknowledgements
The design, building, and commissioning of CLÆSS beamline have been the result of the collaborative efforts of many people including technicians and engineers, without whom these results would not have been achievable. In particular, the authors acknowledge S. Ferrer Fábregas, J. Nicolás Román, C. Colldelram Peroliu, L. Ribó Mor, R. Javier Homs Puron, J. Avila Abellan, J. Prieto Burgos, I. Sics, C. Ruget, and Z. Reszela, for their fundamental help and support. Moreover, the authors wish to acknowledge A. Crisol Ariño and A. Carballedo Costa, from the ALBA engineering section, for the design of the sample setups under development. Finally, the two in situ cells for catalysis have been built in collaboration with Instituto de Tecnologia Quimica de Valencia (ITQ-UPV) within the framework of ICTS granted by the Ministerio de Ciencia e Innovacin of Spain.
Additional information
Funding
Notes on contributors
L. Simonelli
Our group research activities are focused to investigate different functional materials with a particular interest to the study of the interplay between lattice and electronic properties in highly correlated systems and other technologically relevant materials, such as high-Tc superconductors, thermoelectric chalcogenides, battery materials, shape-memory alloys, and environments or health correlated materials.
Our experimental approach is to exploit XAS because of its powerfulness and versatility. XAS is a site-specific probe of the distribution of valence electrons, local structure, and chemistry around a selected absorber atom. Moreover, it does not require crystalline order and is therefore an ideal technique to investigate matter in all its forms: crystals, liquids, glasses, and gases.
XAS is predominantly a synchrotron technique due to its intrinsic necessity to tune constantly the incoming photon energy and because it requires a high photon flux. At ALBA synchrotron, CLASS is a beamline dedicated to XAS.
References
- Balachandran, G., Dixon, D., Bramnik, N., Bhaskar, A., Yavuz, M., Pfaffmann, L., ... Ehrenberg, H. (2015). Elucidation of the electrochemical reaction mechanism in MFe2O4 (M=Ni, Co) conversion-type negative electrode systems by using In situ x-ray sbsorption spectroscopy. ChemElectroChem, 2, 1510–1518.
- Bansode, A., Guilera, G., Cuartero, V., Simonelli, L., Avila, M., & Urakawa, A. (2014). Performance and characteristics of a high pressure, high temperature capillary cell with facile construction for operando x-ray absorption spectroscopy. Review of Scientific Instruments, 85, 084105.
- Blanco, M., Alvarez, P., Blanco, C., Jimenez, M. V., Fernandez-Tornos, J., Perez-Torrente, J. J., & Men{\’e}ndez, R. (2016). Effect of structural differences of carbon nanotubes and graphene based iridium-NHC materials on the hydrogen transfer catalytic activity. Carbon, 96, 66–74.
- Cuartero, V., Blasco, J., Subías, G., García, J., Meneghini, C., & Aquilanti, G. (2015). Stability of Jahn-Teller distortion ordering in LaMn1-xScxO3. Physical Review B, 92, 125118.
- Dom\’{e}nech, B., Romero, V., V\’{a}zquez, M. I., Avila, M., Benavente, J., Mu{\~n}ozd, M., & Macan\’{a}s, J. (2016). Chemical and electrochemical characterization of Nafion containing silver nanoparticles in a stripe-like distribution. RSC Advances, 6, 9923–9931.
- Dom\’{e}nech, B., Ziegler, K., Vigu\’{e}s, N., Olszewski, W., Marini, C., Mas, J., ... Macan\’{a}s, J. (2016). Polyurethane foams doped with stable silver nanoparticles as bactericidal and catalytic materials for the effective treatment of water. New Journal of Chemistry, 40, 3716–3725.
- Domínguez, C., Pérez-Alonso, F.J., Salam, M. A., Al-Thabaiti, S. A., Peña, M. A., Barrio, L., & Rojas, S.(2015). Effect of the N content of Fe/N/graphene catalysts for the oxygen reduction reaction in alkaline media. Journal of Materials Chemistry A, 3, 2448–24494.
- Dom{\’{\i}}nguez, C., P\’{e}rez-Alonso, F., Salam, M. A., Al-Thabaiti, S. A., Pe{\~n}a, M. A., Garc{\’{\i}}a-Garc{\’{\i}}a, F. J., & Barrio, L. (2016). Repercussion of the carbon matrix for the activity and stability of Fe/N/C electrocatalysts for the oxygen reduction reaction. Applied Catalysis B: Environmental, 183, 185–196.
- Escriche-Tur, L., Corbella, M., Font-Bardia, M., Castro, I., Bonneviot, L., & Albela, B. (2015). Biomimetic Mn-catalases based on dimeric manganese complexes in mesoporous silica for potential antioxidant agent. Inorganic Chemistry, 54, 10111–10125.
- Eslava, J. L., Iglesias-Juez, A., Agostini, G., dez-Garcá, M. F., & Guerrero-Rui, A., & Rodr{\’g}uez-Ramos, I. (2016). Time-resolved XAS investigationof the local environment and evolution of oxidation states of a FischerTropsch RuCs/C catalyst. ACS Catalysis, 6, 1437–1445,
- Filipponi, A., & Di Cicco, A. (2000). GNXAS: A software package for advanced EXAFS multiple-scattering calculations and data-analysis. Task Quarterly, 4, 575–669.
- García-Prieto, A., Arteche, A., Aguilera-Granja, F., Torres, M. B., Orue, I., Alonso, J., & Fern{\’a}ndez-Gubieda, M. L. (2015). Breakdown of magnetism in sub-nanometric Ni clusters embedded in Ag. Nanotechnology, 26, 455703.
- Gomez-Gonzaleza, M. A., Voegelinb, A., Garcia-Guineaa, J., Boleac, E., Labordac, F., & Garrido, F. (2016). Colloidal mobilization of arsenic from mining-affected soils by surface runof. Chemosphere, 144, 1123–1131.
- Gr{\"u}nert, W., Gro{\ss}mann, D., Noei, H., Pohl, M.-M., Sinev, I., ... Muhler, M. (2014a). Low-temperature oxidation of carbon monoxide with gold(III) ions supported on titanium oxide. Angewandte Chemie International Edition, 53, 3245–3249.
- Gr{\"u}nert, W., Gro{\ss}mann, D., Noei, H., Pohl, M.-M., Sinev, I., ... Muhler, M. (2014b). How different characterization techniques elucidate the nature of the gold species in a polycrystalline Au/TiO2 Catalyst. Chemie Ingenieur Technik, 86, 1883–1889.
- Koningsberger, D. C., & Prins, R. (1987). X-ray absorption: Principles, applications, techniques of EXAFS, SEXAFS, and XANES, in Chemical Analysis. In S. G. Bunker (Ed.), Introduction to XAF (pp. 92). New York, NY: John Wiley & Sons.
- Kylie, L., Bordet, A., Tricard, S., Sinev, I., Gr{\"u}nert, W., Chaudret, B., & Leitner, W. (2016). Enhancing the Catalytic Properties of RutheniumNanoparticle-SILP Catalysts by Dilution with Iron. ACS Catalysis, 6, 3719–3726.
- Lafuerza, S., García, J., Subías, G., Blasco, J., & Cuartero, V. (2014). Strong local lattice instability in hexagonal ferrites RFe2O4 (R = Lu, Y, Yb) revealed by x-ray absorption spectroscopy. Physical Review B, 89, 045129.
- Lafuerza, S., García, J., Subías, G., Blasco, J., Cuartero, V., & Herrero-Martín, J. (2015). Hard and soft x-rays XAS characterization of charge ordered LuFe2O4. Journal of Physics: Conference Series, 592, 012121.
- Lidon-Simon, J., Fernandez-Carreiras, D., Gigante, J. V., Jamroz, J. J., Klora, J. & Matilla, O. (2011). Low current measurements at Alba. Contributions to the Proceedings of ICALEPCS, Grenoble.
- Masa, J., Xia, W., Sinev, I., Zhao, A., Sun, Z., Gr{\"u}tzke, S., ... Schuhmann, W. (2014). MnxOy/NC and CoxOy/NC nanoparticles embedded in a nitrogen-doped carbon matrix for high-performance bifunctional oxygen electrodes. Angewandte Chemie International Edition, 53, 8508–8512.
- Olszewski, W., Ávila, M., P\’{e}rez, C., Marini, E., Paris, X., Wang, T., & Simonelli, L. (2016). Temperature dependent local structure of NaxCoO2 cathode material for rechargeable sodium-ion batteries. The Journal of Physical Chemistry C, 120, 4227–4232.
- Padilla-Pantoja, J., Herrero-Martín, J., Gargiani, P., Manuel Valvidares, S., Cuartero, V., Kummer, K., ... Garc{\’{\i}}a-Mu{\~n}oz, J. L. (2014). Stability of the cationic oxidation states in Pr0.50Sr0.50CoO3 across the magnetostructural transition by X-ray absorption Spectroscopy. Inorganic Chemistry, 53, 8854–8858.
- Paris, E., Simonelli, L., Wakita, T., Marini, C., Olszewski, W., Terashima, K., & Saini, N. (2016). Temperature dependent local atomic displacements in ammonia intercalated iron selenide superconductor. Scientific Reports, 6, 27646–2764.
- Schacht, E., Zhong, L., Kondratieva, E., Hein, J., Guti\’{e}rre, O. Y., Jentys, A., & Lercher, J. A. (2015). Understanding Ni Promotion of MoS2/-Al2O3 and its Implications for the Hydrogenation of Phenanthrene. ChemCatChem. doi:10.1002/cctc.201500706
- Stekrovaa, M., Kumara, N., Ahoa, A., Sinevd, I., Grünertd, W., Dahle, J., ... Murzina, D. Y. (2014). Isomerization of α-pinene oxide using Fe-supported catalysts: Selective synthesis of campholenic aldehyde. Applied Catalysis A: General, 470, 162–176.
- Stern, E. A., & Heald, S. M. (1983). Basic principles and applications of EXAFS. In E. E. Koch(Ed.), Chapter 10 in Handbook of Synchrotron Radiation (pp. 995–1014). Amsterdam: North-Holland
- Wezendonk, T. A., Santos, V. P., Nasalevich, M. A., Warringa, Q. S. E., Dugulan, A., Chojecki, A., ... Gascon, J. (2016). Elucidating the nature of Fe species during pyrolysis of the Fe-BTC MOF into highly active and stable FischerTropsch catalysts. ACS Catalysis, 6, 3236–3247.
- Caramazza, S., Marini, C., Simonelli, L., Dore, P., & Postorino, P. (2016). Temperature dependent EXAFS study on transition metal dichalcogenides MoX2 (X= S, Se, Te). Journal of Physics: Condensed Matter, 28, 325401.
- van der Linden, P. J. E. M., Sala, M. M., Henriquet, C., Rossi, M., Ohgushi, K., Fauth, F., ... Krisch, M. (xxxx). A dynamic flow cryostat for synchrotron x-ray beamlines. Under review, Review of Scientific Instruments,