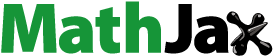
Abstract
First principles calculations based on density functional theory with generalized gradient approximation are performed to investigate the structural, elastic, electronic, and optical properties of new bismuth oxides, ABi2O6 (A = Mg, Zn) with the trirutile-type structure. Initially, the geometry structures obtained by geometry optimization are consistent with the experimental values. The calculated structural parameters show a good agreement with the experimental results. The optimized lattice parameters, six independent elastic constants (C11, C12, C13, C33, C44 and C66), bulk modulus (B), shear modulus (G), Young’s modulus (Y), Pugh’s ratio (G/B), Poisson’s ratio (ν), and elastic anisotropy (A) are calculated and discussed. This is the first quantitative theoretical prediction of the electronic, elastic, and optical properties of these compounds. The investigation of the electronic band structures reveals that these compounds are electrical conductors, with contribution predominantly from the Bi 6p states. The analysis of the elastic constants and other moduli shows large anisotropy on elasticity and brittle behavior. The origins of features that appear in different optical properties of these two compounds have been discussed using band structures. The large reflectivity of the predicted compounds in the low energy region might be helpful in high-quality candidate materials for coating to avoid solar heating.
Public Interest Statement
In this research work, we have studied the structural, elastic, electronic, and optical properties of ABi2O6 (A = Mg, Zn) with trirutile-type structure using the plane-wave ultrasoft pseudo-potential technique, which is based on the first principle density functional theory with generalized gradient approximation. To the best of our knowledge, this is the first quantitative theoretical prediction of the electronic, elastic, and optical properties of these compounds. We hope that this work will help investigate the different properties of other materials of this group.
1. Introduction
Nanoparticles are having different properties compared with bulk materials. Most of the researchers are working with metal oxide nanoparticles because of their unique characteristics such as hydrophobic, photo catalytic, and stability. Hence, they are used in many applications such as coatings, catalysts, anti-bacterial, medical sciences, sensors, semiconductors, capacitors, and batteries (Piriyawong, Thongpool, Asanithi, & Limsuwan, Citation2012). These oxide materials can be prepared by different synthesis methods such as solution combustion (Bai et al., Citation2011), co-precipitation (Vatsha et al., Citation2013), sol–gel (Tamilselvi, Yelilarasi, Hema, & Anbarasan, Citation2013), hydrothermal (Hayashi & Hakuta, Citation2010), solvothermal (Hou, Yu, & Gao, Citation2003), microwave-assisted sol–gel (Mirzaei & Davoodnia, Citation2012), and green synthesis (Patil & Bhanage, Citation2013). MgO is an important material used in many applications like catalysis, toxic waste remediation, paint, superconducting products, and anti-bacterial activities against food-borne pathogens (Jin & He, Citation2011; Mageshwari, Mali, Sathyamoorthy, & Patil, Citation2013; Mastuli, Rusdi, Mahat, Saat, & Kamarulzaman, Citation2012). As an alternative to the good standard TiO2 photocatalyst, the use of zinc oxide (ZnO) as a robust candidate for waste water treatment is widespread due to its similarity in charge carrier dynamics upon band gap excitation and the generation of reactive oxygen species in aqueous suspensions with TiO2. However, the large band gap of ZnO, the massive charge carrier recombination, and the photo-induced corrosion–dissolution at extreme pH conditions, together with the formation of inert Zn (OH)2 during photocatalytic reactions, act as barriers for its extensive applicability. Zinc oxide is widely used as a white pigment in paints and as a catalyst in the manufacture of rubber to disburse heat. It is also used to protect rubber polymers and plastics from ultraviolet radiation (UV) (Emsley, Citation2001). Semiconductor-based photocatalytic oxidation has been established to be one of the most promising technologies for environment remediation and has been employed in the treatment of all kinds of organic contaminants (Chen, Ma, & Zhao, Citation2010; Fujishima, Rao, & Tryk, Citation2000; Hoffmann, Martin, Choi, & Bahnemann, Citation1995). The effective application of photocatalys in environmental remediation requires that the photocatalysts should have high photocatalytic efficiency. Therefore, during the past decade, a tremendous effort has been devoted to the development of new photocatalysts (Ai, Zhang, & Lee, Citation2010; Bi, Ouyang, Cao, & Ye, Citation2011; Chen et al., Citation2011; Dong et al., Citation2009; Ouyang & Ye, Citation2011; Shi, Li, Kou, Ye, & Zou, Citation2011; Yu, Dai, & Huang, Citation2009; Zhu, Xu, Fu, Zhao, & Zhu, Citation2007). The exploration and search for active semiconductor photocatalysts, especially for solar energy applications, is one of the challenging tasks. Bismuth (V)-containing photocatalysts have gained great significance due to their unique electronic band structures. Recently, the trirutile-type structure compounds with general formula ABi2O6 (where A = Mg and Zn,) were found to be promising candidates for application in microwave devices due to the fact that evaluations of the microwave dielectric properties of these compounds reveal a high-quality factor (Q × f) and a high dielectric constant (εr). Many experimental works have been carried out for preparing the trirutile-type structure of ABi2O6 (A = Mg, Zn). Microwave hydrothermal processing has been confirmed to be of greater advantage in kinetics than conventional hydrothermal processing for preparation of various oxides (Komarneni, Li, & Roy, Citation1994; Komarneni, Li, Stefansson, & Roy, Citation1993; Komarneni, Menon, Li, Roy, & Ainger, Citation1996; Komarneni, Pidugu, Li, & Roy, Citation1995; Komarneni, Roy, & Li, Citation1992). Hydrothermal processing can yield novel phases that cannot be prepared by high-temperature solid-state reaction. For example, several new bismuth oxides, Bi2O4 (Kumada, Kinomura, Woodward, & Sleight, Citation1995), LiBiO3 (Kumada, Takahashi, Kinomura, & Sleight, Citation1996), and ABi2O6 (A = Mg, Zn) (Kumada, Takahashi, Kinomura, & Sleight, Citation1997) with unusual oxidation state (Bi5+) were prepared only under the hydrothermal condition below 140°C, using NaBiO3znH2O as a starting material. Unfortunately, no theoretical works have been performed on these two new bismuth oxides, i.e. MgBi2O6 and ZnBi2O6. For the first time, we have performed theoretical investigation on these two compounds by employing CASTEP code (Clark et al., Citation2005) which utilizes the plane-wave pseudo-potential based on the framework of density functional theory (DFT) (Kohn & Sham, Citation1965). In our work, we have studied structural, elastic, electronic, and optical properties of MgBi2O6 and ZnBi2O6. We have focused on optical properties such as dielectric function, refractive index, absorption coefficient, reflectivity, energy loss function, and photoconductivity of both compounds. Today, knowledge of refractive indices and absorption coefficients of solids is especially important for the design and analysis of new optoelectronic devices (Charifi, Reshak, & Baaziz, Citation2009). High dielectric materials can be used in the next generation of microelectronic devices in which the reduced dimension requires gate insulators with high dielectric constants (Xu et al., Citation2006). Our present work is organized in three sections. In Section 2, we briefly discuss the computational techniques used in this study. The results and discussion are presented in Section 3. Finally, a summary of our results is shown in Section 4.
2. Computational method
The zero temperature energy calculations predicted in this research work were carried out by applying the CASTEP code (Clark et al., Citation2005) which utilizes the plane-wave pseudo-potential based on the DFT (Kohn & Sham, Citation1965) framework. The electronic exchange–correlation energy is treated under the generalized gradient approximation using the scheme of Perdew–Burke–Ernzerhof (Perdew, Burke, & Ernzerhof, Citation1996). Using ultrasoft Vanderbilt-type pseudo-potentials, we represented the interactions between ion and electron of atoms Mg, Bi, Zn, and O (Vanderbilt, Citation1990). The pseudo-atomic calculations were performed for Mg—2p63s2, Zn—3d104s2, Bi—6s26p3, and O—2s22p4. The k-point sampling of the Brillouin zone was constructed using Monkhorst–Pack scheme (Monkhorst & Pack, Citation1976) with 10 × 10 × 12 grids in primitive cells of MgBi2O6 and ZnBi2O6. Also the k-points 8 × 8 × 10 were used to calculate the elastic constants of MgBi2O6. The value of the energy cut-off was set to 350 eV and the electromagnetic wave functions were expanded in a plane wave basis set with this energy. The equilibrium crystal structures are relaxed by Broyden–Fletcher–Goldfarb–Shanno (BFGS) minimization scheme (Almlöf, Fischer, Gassman, Ghosh, & Häser, Citation1993). Geometry optimization was performed using with the total energy of 1 × 10−5 eV/atom, maximum force of 0.002 eV/Å, maximum stress of 0.05 GPa, and the maximum atomic displacement of 1 × 10−3 Å. Mulliken bond population, electronic density of states, elastic constants Cij, and optical properties were directly calculated using the CASTEP code.
3. Results and discussion
3.1. Structural properties
New bismuth oxides, ABi2O6 (A = Mg, Zn), with the trirutile-type structure possess tetragonal crystal structure with space group P42/mnm (No. 136). The atomic positions in the unit cell of this compound are A (0 0 0), Bi (0 0 0.332), and O (0.305 0.305 0). The equilibrium tetragonal lattice parameters are a = 4.8260 Å and c = 9.7190 Å (Eicher, Greedan, & Lushington, Citation1986; Reimers, Greedan, Stager, & Kremer, Citation1989) A for A = Mg and a = 4.8386 Å and c = 9.7422 Å (Eicher et al., Citation1986; Kumada, Takahashi, & Kinomura, Citation1997; Reimers et al., Citation1989) A for A = Zn. The geometrical optimized lattice constants are as follows: a = 10.021 Å c = 7.108 Å for MgBi2O6 and a = 10.021 Å c = 7.108 Å for compound ZnBi2O6. We optimized the lattice constants and atomic positions as a function of normal stress by minimizing the total energy. The optimized structures of MgBi2O6 and ZnBi2O6 are shown in Figures and , respectively. The calculated lattice parameters are listed in Table . We can see that the lattice constant a is slightly smaller and c is slightly larger than that from Kumada, Takahashi, and Kinomura (Citation1997).
Figure 1. Crystal structures of MgBi2O6 (a) the conventional tetragonal cell and (b) the primitive cell.
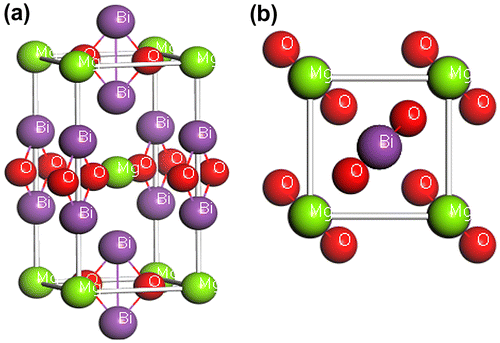
Figure 2. Crystal structures of ZnBi2O6 (a) the conventional tetragonal cell and (b) the primitive cell.
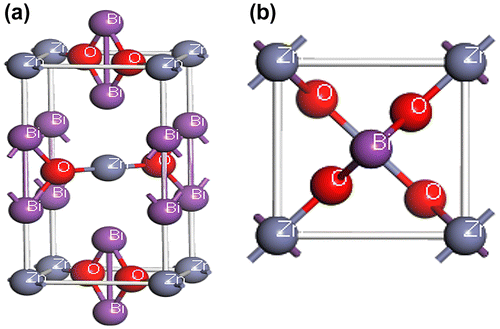
Table 1. The calculated equilibrium lattice parameters “a” and c, unit cell volume “V0”, and bulk modulus “B0” of MgBi2O6 and ZnBi2O6
The existing deviation of lattice parameters from the experimental values can be attributed to the fact that our calculated data are simulated at 0 K, while the experimental data are measured at room temperature. This indicates the reliability of our present DFT-based calculations.
3.2. Elastic properties
The elastic properties of materials give useful information about the bonding character between neighboring atomic planes, the anisotropic nature of the bonding, the structural stability, and the rigidity of materials. These properties also provide information about the interatomic potentials, interatomic bonding, thermal expansion, Debye temperature, phonon spectra, and specific heat capacity (Bouhemadou, Khenata, Chegaar, & Maabed, Citation2007; Pan, Xia, Ye, & Ding, Citation2012; Ponce, Casali, & Caravaca, Citation2008). Elastic constants are defined by means of a Taylor expansion of the total energy, namely: the derivative of the energy as a function of a lattice strain (Clark et al., Citation2005; Perdew & Zunger, Citation1981). In order to study the elastic properties of MgBi2O6 and ZnBi2O6, the elastic constants Cij, bulk modulus B, shear modulus G, Young’s modulus Y, Poisson’s ratio ν, and the anisotropic factor A have been calculated for the first time. There are six independent elastic coefficients Cij, i.e. C11, C12, C13, C33, C44, and C66, for tetragonal crystal-like MgBi2O6 and ZnBi2O6. At present, no theoretical data are available because of first time study. For a stable tetragonal structure, the six independent elastic constants Cij should satisfy the following Born–Huang criteria (Roknuzzaman & Islam, Citation2013):(1)
(1)
(2)
(2)
(3)
(3)
The calculated elastic constants are presented in Table . All the coefficients are positive and fulfill the above stability conditions, i.e. Equations (1)–(3). This suggests that both materials are mechanically stable compounds. The elastic anisotropy of a crystal depends on the orientation of the elastic moduli. A suitable explanation of such an anisotropic manner has an important implication in manufacturing science as well as in solid-state (crystal) physics. The elastic anisotropy of the shear of hexagonal crystals, defined by A = 2C44/(C11–C12), may be responsible for the development of microcracks in the material (Medvedeva, Enyashin, & Ivanovskii, Citation2011). For a completely isotropic crystal, the value of A = 1. Any value of A either smaller or greater than unity indicates elastic anisotropy. The deviated magnitude of the anisotropic factor A from unity is used for measuring the degree of elastic anisotropy possessed by the crystal. To measure the elastic anisotropy of the trirutile crystal structure of ABi2O6 (A = Mg and Zn), we have evaluated the Zener anisotropy factor A. The calculated Zener anisotropy factor for MgBi2O6 and ZnBi2O6 is presented in Table . As shown in Table , the value of A indicates that both the materials can be regarded as elastically anisotropic materials. Another anisotropy parameter that can be defined by the ratio between linear compressibility coefficients along the c- and a-axes for tetragonal crystal is kc/ka = (C11 + C12 – 2C13)/(C33 – C13). The unit value of this ratio means that the compressibility along both directions is same, i.e. the crystal is isotropic but any value less (or greater) than unity indicates that the compressibility along the c-axis is either smaller or larger than that along the a-axis. Our result reveals that the compressibility along the c-axis is slightly larger than that along the a-axis for MgBi2O6, but for ZnBi2O6, the compressibility is reversed, as c-axis is stiffer than that along the a-axis. This parameter also indicates that the materials MgBi2O6 and ZnBi2O6 are anisotropic.
Table 2. The calculated elastic constants Cij (in GPa), the shear anisotropic factors A, and kc/ka of MgBi2O6 and ZnBi2O6
We have estimated the bulk modulus (B) and shear modulus (G) of polycrystalline aggregates from individual elastic constants, Cij, by the well-known Voigt (Monkhorst & Pack, Citation1976) and the Reuss (Reuss & Angew, Citation1929) approximations that are recurrently used in averaging the single crystal elastic constants for polycrystalline manners. Voigt assumes a uniform strain throughout a polycrystalline aggregate and Reuss assumes uniform stress. The bulk modulus BV and shear modulus GV in the Voigt approximation for the tetragonal lattice system are expressed as:(4)
(4)
(5)
(5)
In the Reuss approximation, the bulk modulus BR and shear modulus GR are expressed as:(6)
(6)
(7)
(7)
It is evident that the Voigt and Reuss assumptions are true only for isotropic crystals, but for an anisotropic crystal, their assumptions become immediately invalid. Hill (Citation1952) showed that for an anisotropic crystal, the Voigt and Reuss assumptions result in theoretical maximum and minimum values of the isotropic elastic moduli of the polycrystalline crystals, respectively, and suggested that the actual effective moduli of anisotropic polycrystalline crystals could be approximated by the arithmetic mean of the two values. According to Hill approximation, the bulk modulus B and shear modulus G are given by:(8)
(8)
Again, from the calculated bulk modulus B and shear modulus G, we can estimate the Young’s modulus Y and Poisson’s ratio ν by the following relations:(9)
(9)
Using Equations (4)–(9), the calculated bulk modulus BR, BV, B, shear modulus GR, GV, G, Young’s modulus Y, compressibility K, and Poisson’s ratio ν are listed in Table . It is seen that the difference between BV and BR as well as GV and GR is comparatively small. According to Hill, the difference between these limiting values may be proportional to the degree of elastic anisotropy of crystal. According to Pugh’s criteria (Pugh, Citation1954), the ductility of a material can be roughly calculated by the ability of performing shear deformation, such as the value of shear to bulk modulus ratios (G/B). For a ductile material, the ratio of G/B is low (<0.5); otherwise, the material shows brittle nature. From our calculations, we see that G/B ratios for MgBi2O6 and ZnBi2O6 are ~0.5 and ~0.43, indicating that the two compounds are near border line of ductility. The same can be inferred from an additional argument that the difference in the ductile/brittle nature follows from the calculated Poission’s ratio (ν). The value of ν is small enough for brittle material, whereas the value is typically 0.33 (Haines, Léger, & Bocquillon, Citation2001) for ductile metallic materials. As evident from Table , the calculated Poission’s ratio for MgBi2O6 and ZnBi2O6 indicates that both phases are on the more ductile/brittle border line.
Table 3. The calculated bulk moduli (BR, BV, B in GPa), shear moduli (GR, GV, G in GPa), Young’s modulus (Y in GPa), compressibility (K in GPa−1), G/B, and Poisson’s ratio (ν) of MgBi2O6 and ZnBi2O6
3.3 Electronic properties
The energy bands of new bismuth oxides, ABi2O6 (A = Mg and Zn), with trirutile-type structures along the high symmetry directions (Г-X-M-Г-R-X) of the Brillouin zone in the energy range from −50 eV to +10 eV for MgBi2O6 and from −25 eV to +10 eV for ZnBi2O6 are depicted in Figure . The energy band structures of the two compounds are to some extent alike. We see from the band structure diagrams that there is no band gap at the Fermi level for both phases due to the overlapping of the valence bands and conduction bands, thus indicating the metallic nature of the materials under study.
Figure 3. Electronic band structures of (a) MgBi2O6 and (b) ZnBi2O6 along high symmetry direction in the Brillouin zones.
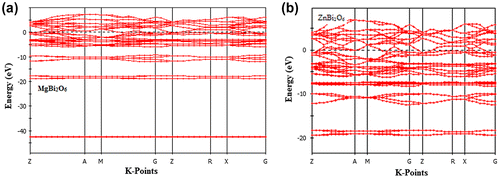
Figure (a) and (b) presents the calculated total and partial densities of states for the materials of MgBi2O6 and ZnBi2O6. At the Fermi level, the values of the density of states are 3.14 and 2.92 states per unit cell per eV which mainly have contributions from Bi 6p states of 3.55 and 2.00 states per eV of the two phases MgBi2O6 and ZnBi2O6, respectively. Mg and Zn have no significant role in the conduction properties due to their poor contributions at the Fermi level. The valence bands of MgBi2O6 and ZnBi2O6 have several energy bands (Figure ). For MgBi2O6, an intense peak of total partial density of state in the lower valence bands from −43 eV to −42 eV below the Fermi level is formed by the main contributions of Mg 2p states. After a large band gap (−42 eV to −19 eV), the next two bands in the energy range from −19 eV to −17 eV and from −12 to −9 eV and originate from the dominating contributions of O 2s and Bi 6s states for MgBi2O6. On the other hand, in case of ZnBi2O6, the dominating contribution on the formation of the lower valence bands from −19 to −17 eV originally comes from the O 2s states. No bands are observed below the Fermi level within the energy range −17 to −12 eV. The next energy band from −12 eV to −9 eV is found due to the Bi 6s states with a little mixture of O 2p states. The more intense peak is formed in the energy range from −8 to −6 eV due to the dominating involvement of Zn 3d states with poor contributions of Bi 6s-p and O 2p orbitals. The top of the valence bands (from −6 eV to Fermi level) is mainly consistent with the strong hybridization of Bi 6p and O 2p states and above the Fermi level, Bi 6p states dominate with less contribution from O 2p and Bi 6s states for both compounds.
3.4 Optical properties
The study of the optical functions of solids helps give a better understanding of the electronic structure of different materials. The frequency-dependent dielectric function defined by ε (ω) = ε1(ω) + iε2(ω) is closely related to the electronic band structure. It fully describes the optical properties of any homogeneous medium at all photon energies. The imaginary part ε2(ω) of the complex dielectric function is obtained from the momentum matrix elements between the occupied and the unoccupied electronic states. This is calculated directly using (Materials Studio CASTEP manual_Accelrys, Citation2010):(10)
(10)
where ω is the frequency of light, e is the electronic charge, is the vector defining the polarization of the incident electric field, and
and
are the conduction and valence band wave functions at k, respectively. The real part of the dielectric function ε1(ω) is derived from the imaginary part of the dielectric function ε2(ω) through the Kramers–Kronig relations. Other optical properties, such as refractive index, absorption spectrum, loss function, reflectivity, and conductivity (real part), are derived from Equations (49)–(54) developed in Ref. (Materials Studio CASTEP manual_Accelrys, Citation2010).
Figures and display the optical functions of MgBi2O6 and ZnBi2O6 calculated for photon energies up to 30 eV and 55 eV for polarization vector [100]. We have used a 0.5 eV Gaussain smearing for all calculations for the reason that this smears out the Fermi level, so that k-points will be more effective on the Fermi surface. Reflectivity is the ratio of energy of a wave reflected from a surface to the energy possessed by the wave striking by the surface. The reflectivity spectra of materials MgBi2O6 and ZnBi2O6 are displayed in Figures (a) and (a) as function of photon energy. It is observed that the reflectivity of both materials shows the same features. The reflectivity is much higher in the infrared region. It starts with a higher value of ~0.55, at first decreases up to a value of ~0.45, and then rises again to reach second maximum value of ~0.49 in the energy range of 0–3 eV. In the moderate infrared region, it drops drastically from a value of ~0.49 to ~0.31. With an increase in photon energy, it makes some several peaks before falling rapidly to a lower value. For both compounds, the large reflectivity for E < 1 eV indicates the characteristics of high conductance in the low energy region. The absorption coefficient gives important information about solar energy conversion efficiency and it indicates how far light of specific frequency can penetrate into the material before being absorbed. The absorption spectra for MgBi2O6 and ZnBi2O6 are presented in Figures (b) and (b) as a function of photon energy. It is seen that the absorption coefficient for both compound starts at zero photon energy due their metallic nature and it rises sharply in the infrared region to reach a peak value at 9 eV for polarization direction [100] with some transitions. After that, the absorption coefficient drops radically up to 17 eV. There is no absorption of photon above 17 eV for ZnBi2O6. But in case of MgBi2O6, another absorption peak is found within the region 41 eV to 50 eV. The refractive index of an optical medium is a dimensionless number that determines how light is bent, or refracted, when it propagates through that medium. Figures (c) and (c) display both the real and imaginary parts of the refractive indices for MgBi2O6 and ZnBi2O6. The static indices of refraction are 7.5 for MgBi2O6 and 6.5 for ZnBi2O6. These values of refractive indices drop sharply up to 4 eV from its peak value and then remain constant in the ultraviolet region. On the other hand, the imaginary part of refractive index indicates the amount of absorption loss when electromagnetic wave passes through the materials. The extinction coefficients, i.e. the imaginary parts of refractive indices, show the same qualitative features for both compounds. These values are high in the low energy region where the photon absorption is high and reduce to zero in the high energy region which indicates there is no absorption of photon. But for MgBi2O6, non-zero value of the extinction coefficient is also found in the energy range 41–50 eV resulting in the absorption of photon in that energy range, which is also evident from Figures (b) and (b). The dielectric function describes the polarization and absorption properties of a material. The real part of dielectric function (ε1) represents how much a material becomes polarized when an electric field is applied and the imaginary part of the dielectric function (ε2) represents the absorption of photon in that material. The real and imaginary parts of the dielectric functions for MgBi2O6 and ZnBi2O6 are illustrated in Figures (d) and (d) as function of photon energy. It is observed that the value of ε2 is zero at 18 eV for both materials and hence these materials become transparent above 17 eV. The value of static dielectric constant is high, i.e. 55 for MgBi2O6 and 45 for ZnBi2O6. These high values of static dielectric constants indicate that both materials can be used in the manufacture of high value capacitors. We also observe that the real part of dielectric functions (ε1) becomes zero at about 10 to 20 eV and 47 eV for MgBi2O6 and at about ~15 eV for ZnBi2O6. These correspond to the energy at which the reflectivity exhibits a sharp drop and the energy loss function shows a prominent peak. The peak of the imaginary part of the dielectric function is related to the electron excitation. For the imaginary part of ε2, the peak for <1 eV is due to intraband transitions. Photoconductivity is an optical and electrical phenomenon in which a material becomes more electrically conductive due to the absorption of electromagnetic radiation. Figures (e) and 6(e) show the photoconductivity of materials MgBi2O6 and ZnBi2O6. It is seen that the photoconductivity of both materials starts with zero photon energy due to the overlapping of the valence and conduction bands at the Fermi level. Moreover, it is observed from our band structure calculation (Figure ) that both materials have no band gap. Both compounds show high electrical conductivity in the low energy region. The prominent peak is found within the energy range 0–3 eV; after that, all the spectra gradually decrease to zero with some several intermediate peaks at ~17 eV. There is no photoconductivity when the photon energy is higher than ~17 eV for ZnBi2O6. But for MgBi2O6, photoconductivity becomes zero when the photon energy is higher than ~17 eV except within the energy range 41–50 eV. The energy loss function of a material is a parameter that describes the energy loss of a fast electron traversing that material. The frequency associated with the highest peak of energy loss spectrum is defined as the bulk plasma frequency ωp of the material, which appears at and ε1 = 0 (de Almeida & Ahuja, Citation2006; Saniz, Citation2006). The energy loss spectra of MgBi2O6 and ZnBi2O6 are depicted in Figures (f) and (f) as a function of photon energy. For MgBi2O6, there are two major peaks found at 11.50 eV and 15 eV, which indicate the rapid reduction in the reflectance. On the other hand, the maximum peak is found at 15 eV for ZnBi2O6. Hence, it is found that the plasma frequency for both materials is 15 eV. When the incident photon energy is higher than 15 eV, both materials will become transparent.
4. Conclusion
We have carried out first principles calculations based on DFT to study the structural, elastic, electronic, and optical properties of MgBi2O6 and ZnBi2O6. The calculated elastic constants and different elastic anisotropies of MgBi2O6 and ZnBi2O6 are discussed in detail in our study. The calculated elastic parameters allow us to conclude that both compounds are mechanically stable. In addition, MgBi2O6 and ZnBi2O6 are near the boundary line of ductility and elastically anisotropic. From our band structure calculations, it is clear that both compounds exhibit metallic-like behavior. At the Fermi level, the total densities of states originated from the dominating contributions of Bi 6p states, and hence Bi 6p orbitals play a vital role in the conduction properties of both MgBi2O6 and ZnBi2O6. Mg and Zn do not have contributions to the densities of states at the Fermi level and therefore are not involved in conduction properties. The major features in the spectra of the optical parameters have also been discussed. The high values of static dielectric constants of both materials indicate that these compounds can be used in the manufacture of high value capacitors. From the reflectivity spectra, it is seen that the large reflectivity of MgBi2O6 and ZnBi2O6 for E < 1 eV indicate the characteristics of high conductance in the low energy region and suitability of the materials for utilize in solar cell to remove solar heating. To the best of our knowledge, the physical properties of MgBi2O6 and ZnBi2O6 have not been reported. We expect that our study would help researchers pursue their investigation on these two hypothetical compounds, both theoretically and experimentally, in the future.
Additional information
Funding
Notes on contributors
Md. Atikur Rahman
Our research groups have studied to examine special properties such as structural, elastic, electronic, optical, and thermodynamic properties of different materials at ambient conditions and under pressure. All the calculations have been performed using the plane-wave pseudo-potential technique based on the density functional theory implemented in the CASTEP code with generalized gradient approximation. Very recently, our groups have also worked to prepare crystalline powder by solid-state reaction method by carbolite furnace. The composition and crystalline phase will be confirmed by X-ray powder diffraction. We have also synthesized single crystals of oxide materials by Bridgman method using modified horizontal Bridgman furnace.
References
- Ai, Z. H., Zhang, L. Z., & Lee, S. C. (2010). Efficient visible light photocatalytic oxidation of NO on aerosol flow-synthesized nanocrystalline InVO4 hollow microspheres. The Journal of Physical Chemistry C, 114, 18594–18600.10.1021/jp106906s
- Almlöf, J., Fischer, T. H., Gassman, P. G., Ghosh, A., & Häser, M. (1993). Electron correlation in tetrapyrroles. Ab Initio calculations on porphyrin and the tautomers of chlorin. Journal of Physical Chemistry, 97, 10964–10970.
- Bai, J., Meng, F., Wei, C., Zhao, Y., Tan, H., & Liu, J.. (2011). Solution combustion synthesis and characteristics of nanoscale MgO powders. Ceramics–Silikáty, 55, 20–25.
- Bi, Y., Ouyang, S., Cao, J., & Ye, J. (2011). Facile synthesis of rhombic dodecahedral AgX/Ag3PO4 (X=Cl, Br, I) heterocrystals with enhanced photocatalytic properties and stabilities. Physical Chemistry Chemical Physics, 13, 10071.10.1039/c1cp20488b
- Bouhemadou, A., Khenata, R., Chegaar, M., & Maabed, S. (2007). First-principles calculations of structural, elastic, electronic and optical properties of the antiperovskite AsNMg3. Physics Letters A, 371, 337–343.10.1016/j.physleta.2007.06.030
- Charifi, Z., Reshak, A. H., & Baaziz, H. (2009). Electronic band structures of AV(2) (A = Ta, Ti, Hf and Nb) Laves phase compounds. Journal of Physics: Condensed Matter, 21, 025502. doi:10.1088/0953-8984/21/2/025502
- Chen, C. C., Ma, W. H., & Zhao, J. C. (2010). Semiconductor-mediated photodegradation of pollutants under visible-light irradiation. Chemical Society Reviews, 39, 4206–4219.10.1039/b921692h
- Chen, Y., Hu, S., Liu, W., Chen, X., Wu, L., Wang, X., … Li, Z. (2011). Controlled syntheses of cubic and hexagonal ZnIn2S4 nanostructures with different visible-light photocatalytic performance. Dalton Transactions, 40, 2607–2613.10.1039/c0dt01435d
- Clark, S. J., Segall, M. D., Pickard, C. J., Hasnip, P. J., Probert, M. J., Refson, K., & Payne, M. C. (2005). First principles methods using CASTEP. Zeitschrift fuer Kristallographie, 220, 567–570. doi:10.1524/zkri.220.5.567.65075
- de Almeida, J. S., & Ahuja, R. (2006). Electronic and optical properties of RuO2 and IrO2. Physical Review B, 73, 165102–165106.10.1103/PhysRevB.73.165102
- Dong, H., Li, Z., Xu, X., Ding, Z., Wu, L., Wang, X., & Fu, X. (2009). Visible light-induced photocatalytic activity of delafossite AgMO2 (M=Al, Ga, In) prepared via a hydrothermal method. Applied Catalysis B: Environmental, 89, 551–556.10.1016/j.apcatb.2009.01.018
- Eicher, S. M., Greedan, J. E., & Lushington, K. J. (1986). The magnetic properties of FeTa2O6. Magnetic structure and low-dimensional behavior. Journal of Solid State Chemistry, 62, 220–230.
- Emsley, J.. (2001). Nature’s building blocks: An A-Z guide to the elements (pp. 499–505). Oxford: Oxford University Press.
- Fujishima, A., Rao, T. N., & Tryk, D. A. (2000). Titanium dioxide photocatalysis. Journal of Photochemistry and Photobiology C: Photochemistry Reviews, 1, 1–21.10.1016/S1389-5567(00)00002-2
- Haines, J., Léger, J. M., & Bocquillon, G. (2001). Synthesis and design of superhard materials. Annual Review of Materials Research, 31, 1.10.1146/annurev.matsci.31.1.1
- Hayashi, H., & Hakuta, Y. (2010). Hydrothermal synthesis of metal oxide nanoparticles in supercritical water. Materials, 3, 3794–3817.
- Hill, R. (1952). The elastic behaviour of a crystalline aggregate. Proceedings of the Physical Society. Section A, 65, 349.10.1088/0370-1298/65/5/307
- Hoffmann, M. R., Martin, S. T., Choi, W., & Bahnemann, D. W. (1995). Environmental applications of semiconductor photocatalysis. Chemical Reviews, 95, 69–96.10.1021/cr00033a004
- Hou, Y., Yu, J., & Gao, S. (2003). Solvothermal reduction synthesis and characterization of superparamagnetic magnetite nanoparticles, The royal society of chemistry. Journal of Materials Chemistry, 13, 1983–1987.10.1039/b305526d
- Jin, T., & He, Y. (2011). Antibacterial activities of magnesium oxide (MgO) nanoparticles against foodborne pathogens. Journal of Nanoparticle Research, 13, 6877–6885.10.1007/s11051-011-0595-5
- Kohn, W., & Sham, L. J. (1965). Self-consistent equations including exchange and correlation effects. Physical Review, 140, A1133.
- Komarneni, S., Li, Q. H., & Roy, R. (1994). Microwave–hydrothermal processing for synthesis of layered and network phosphates. Journal of Materials Chemistry, 4, 1903.10.1039/JM9940401903
- Komarneni, S., Li, Q.H., Stefansson, K. M., & Roy, R. (1993). Microwave-hydrothermal processing for synthesis of electroceramic powders. Journal of Materials Research, 8, 3176.10.1557/JMR.1993.3176
- Komarneni, S., Menon, V. C., Li, Q. H., Roy, R., & Ainger, F. (1996). Microwave-hydrothermal processing of BiFeO3 and CsAl2PO6. Journal of the American Ceramic Society, 79, 1409.10.1111/jace.1996.79.issue-5
- Komarneni, S., Pidugu, R., Li, Q. H., & Roy, R. (1995). Microwave-hydrothermal processing of metal powders. Journal of Materials Research, 10, 1687.10.1557/JMR.1995.1687
- Komarneni, S., Roy, R., & Li, Q. H. (1992). Microwave-hydrothermal synthesis of ceramic powders. Materials Research Bulletin, 27, 1393.10.1016/0025-5408(92)90004-J
- Kumada, N., Kinomura, N., Woodward, P. W., & Sleight, A. W. (1995). Crystal structure of Bi2O4 with β-Sb2O4-type structure. Journal of Solid State Chemistry, 116, 28110.1006/jssc.1995.1214
- Kumada, N., Takahashi, N., & Kinomura, N. (1997). Preparation of ABi2O6 (A = Mg, Zn) with the trirutile-type structure. Material Research Bulletin, 32, 1003–1008.
- Kumada, N., Takahashi, N., Kinomura, N., & Sleight, A. W. (1996). Preparation and crystal structure of a new lithium bismuth oxide: LiBiO3. Journal of Solid State Chemistry, 126, 121.10.1006/jssc.1996.0319
- Kumada, N., Takahashi, N., Kinomura, N., & Sleight, A. W. (1997). Preparation of ABi2O6 (A = Mg, Zn) with the trirutile-type structure. Materials Research Bulletin, 32, 1003.10.1016/S0025-5408(97)00071-8
- Mageshwari, K., & Mali, S. S., Sathyamoorthy, R., & Patil, P. S. (2013). Template-free synthesis of MgO nanoparticles for effective photocatalytic applications. Powder Technology, 249, 456–462.10.1016/j.powtec.2013.09.016
- Mastuli, M. S., Rusdi, R., Mahat, A. M., Saat, N., & Kamarulzaman, N. (2012). Sol-gel synthesis of highly stable nano sized MgO from magnesium oxalate dihydrate. Advanced Materials Research, 545, 137–142.10.4028/www.scientific.net/AMR.545
- Materials Studio CASTEP manual_Accelrys. (2010). Retrieved from http://www.tcm.phy.cam.ac.uk/castep/documentation/WebHelp/CASTEP.html
- Medvedeva, N. I., Enyashin, A. N., & Ivanovskii, A. L. (2011). Modeling of the electronic structure, chemical bonding, and properties of ternary silicon carbide Ti3SiC2. Journal of Structural Chemistry, 52, 785–802.10.1134/S0022476611040226
- Mirzaei, H., & Davoodnia, A. (2012). Microwave assisted sol-gel synthesis of MgO nanoparticles and their catalytic activity in the synthesis of hantzsch 1,4-dihydropyridines. Chinese Journal of Catalysis, 33, 1502–1507.10.1016/S1872-2067(11)60431-2
- Monkhorst, H. J., & Pack, J. D. (1976). Special points for Brillouin-zone integrations. Physical Review B, 13, 5188–5192.10.1103/PhysRevB.13.5188
- Ouyang, S. X., & Ye, J. H. (2011). β-AgAl1−xGaxO2 solid-solution photocatalysts: Continuous modulation of electronic structure toward high-performance visible-light. Journal of the American Chemical Society, 133, 7757–7763.10.1021/ja110691t
- Pan, L.-X., Xia, Q.-L., Ye, S.-L., & Ding, N. (2012). First principles study of electronic structure, chemical bonding and elastic properties of BiOCuS. Transactions of Nonferrous Metals Society of China, 22, 1197–1202.10.1016/S1003-6326(11)61305-8
- Patil, A. B., & Bhanage, B. M. (2013). Novel and green approach for the nanocrystalline magnesium oxide synthesis and its catalytic performance in Claisen–Schmidt condensation. Catalysis Communications, 36, 79–83.10.1016/j.catcom.2013.03.012
- Perdew, J. P., Burke, K., & Ernzerhof, M. (1996). Generalized gradient approximation made simple. Physical Review Letters, 77, 3865.10.1103/PhysRevLett.77.3865
- Perdew, J. P., & Zunger, A. (1981). Self-interaction correction to density-functional approximations for many-electron systems. Physical Review B, 23, 5048–5079.10.1103/PhysRevB.23.5048
- Piriyawong, V., Thongpool, V., Asanithi,P., & Limsuwan, P. (2012). Preparation and characterization of alumina nanoparticles in deionized water using laser ablation technique. Hindawi Publishing Corporation Journal of Nanomaterials, 819403, 1–6.
- Ponce, C. A., Casali, R. A., & Caravaca, M. A. (2008). Ab initio study of mechanical and thermo-acoustic properties of tough ceramics: Applications to HfO2 in its cubic and orthorhombic phase. Journal of Physics: Condensed Matter, 20(4), 045213.
- Pugh, S. F. (1954). XCII. Relations between the elastic moduli and the plastic properties of polycrystalline pure metals. The London, Edinburgh, and Dublin Philosophical Magazine and Journal of Science, 45, 823.10.1080/14786440808520496
- Reimers, J. N., Greedan, J. E., Stager, C. V., & Kremer, R. (1989). Crystal structure and magnetism in CoSb2O6 and CoTa2O6. Journal of Solid State Chemistry, 83, 20.10.1016/0022-4596(89)90049-2
- Reuss, A., & Angew, Z. (1929). Mathematics and Mechanics, 9, 49.
- Roknuzzaman, M., & Islam, A. K. M. A. (2013). Ab initio investigation of nitride in comparison with carbide phase of superconducting Ti2InX (X = C, N). Condensed Matter Physics, 2013, 646042. doi:10.1155/2013/646042
- Saniz, R. (2006). Structural, electronic, and optical properties of NiAl 3 : First-principles calculations. Physical Review B, 74, 014209–14209.10.1103/PhysRevB.74.014209
- Shi, H. F., Li, Z. S., Kou, J. H., Ye, J. H., & Zou, Z. G. (2011). Facile synthesis of single-crystalline Ag2V4O11 nanotube material as a novel visible-light-sensitive photocatalyst. The Journal of Physical Chemistry C, 115, 145–151.10.1021/jp102680y
- Tamilselvi, P., Yelilarasi, A., Hema, M., & Anbarasan, R. (2013). Synthesis of hierarchical structured MgO By sol-gel method. Nano Bulletin, 2, 1–6.10.3390/ma3073794
- Vanderbilt, D. (1990). Soft self-consistent pseudopotentials in a generalized eigenvalue formalism. Physical Review B, 41, 7892–7895.10.1103/PhysRevB.41.7892
- Vatsha, B., Tetyana, P., Shumbula, P. M., Ngila, J. C., Sikhwivhil, L. M., & Moutloali, R. M. (2013). Effects of precipitation temperature on nanoparticle surface area and antibacterial behaviour of Mg(OH)2 and MgO nanoparticles. Journal of Biomaterials and Nanobiotechnology, 04, 365–373.10.4236/jbnb.2013.44046
- Xu, M., Wang, S., Yin, G., Li, J., Zheng, Y., Chen, L., & Jia, Y. (2006). Optical properties of cubic Ti3N4, Zr3N4, and Hf3N4. Applied Physics Letters, 89, 1519083. doi:10.1063/1.2360937
- Yu, J. G., Dai, G. P., & Huang, B. B. (2009). Fabrication and characterization of visible-light-driven plasmonic photocatalyst Ag/AgCl/TiO2 nanotube arrays. The Journal of Physical Chemistry C, 113, 16394–16401.10.1021/jp905247j
- Zhu, S., Xu, T., Fu, H., Zhao, J., & Zhu, Y. (2007). Synergetic effect of Bi2WO6 photocatalyst with C60 and enhanced photoactivity under visible irradiation. Environmental Science & Technology, 41, 6234–6239.10.1021/es070953y