Abstract
High-quality cobalt vanadate crystals have been synthesized by solid-state reaction route. Structure and morphology of the synthesized powders were investigated by X-ray diffraction (XRD), scanning electron microscopy (SEM), and Fourier transform infrared (FT-IR) spectroscopy. The XRD patterns revealed that the as prepared materials are of high crystallinity and high quality. The SEM images showed that the crystalline CoV2O6 material is very uniform and well separated, with particle (of) area ~252 μm. The electronic and optical properties were investigated by impedance analyzer and UV–visible spectrophotometer. Temperature-dependent electrical resistivity was measured using four-probe technique. The crystalline CoV2O6 material is a semiconductor and its activation energy is 0.05 eV.
Public Interest Statement
In this work, high-quality cobalt vanadate crystals have been synthesized by solid-state reaction method. The cobalt vanadate crystals might be used as a potential anode material for lithium-ion rechargeable batteries. Lithium-ion rechargeable batteries are most important parts of the modern science and technology due to its usage as power sources in portable electronic devices. The efficiency of the lithium-ion batteries depends on the properties of anode and cathode materials. In this work, we tried to improve the efficiency of lithium-ion batteries by enhancing quality of anode materials.
1. Introduction
Recently, lithium-ion rechargeable batteries (LIBs) have been much studied for their superior performance including high power and energy density, long life cycle, and safety. Nowadays, LIBs have wide applications in portable electronic devices such as cell phones, laptop computers, digital cameras, electronic vehicles, and power tools (Goodenough & Kim, Citation2010; Myung, Amine, & Sun, Citation2010; Song, Lee, Kim, Nazar, & Cho, Citation2010; Tarascon & Armand, Citation2001; Yoshino, Citation2012). A rechargeable LIB is basically composed of an anode, a cathode, and an electrolyte. Typically, graphite is used as anode of commercial LIBs (Fan & Chen, Citation2011; Li, Wang, Chen, & Huang, Citation2009). However, graphite anode materials cannot meet the need of large-scale batteries in the future because of their some demerits such as low voltage, low capacity, and poor cyclability (Jiang, Li, Liu, & Huang, Citation2011). To overcome the problem, considerable efforts should be devoted to find out alternative anode materials to replace graphite anode. Therefore, materials for cathodes in rechargeable LIBs have known a renewed research interest both from the experimental and theoretical point of view (Catti, Citation2000; Koksbang, Barker, Shi, & Saidi, Citation1996; Thackeray, Citation1995).
At present, transition metal vanadium-based oxides have attracted extensive attractions as anode materials of LIBs for their special chemical properties. They have also applications in the fields such as catalysts, chemical sensors, optical devices, and super capacitors for their extraordinary physical and chemical properties (Ma, Zhang, Ji, Tao, & Chen, Citation2008; Mai et al., Citation2010; Ramkumar & Minakshi, Citation2015; Shi, Li, Kou, Ye, & Zou, Citation2011; Xu, Lin, Jia, & Zhaorigetu, Citation2008). Among this group, cobalt-based vanadate is one of the most promising materials for anode of LIBs because of its favorable features such as good capacity, high discharge voltage, excellent electrochemical stability, and life cycle (Khatun, Gafur, Ali, Islam, & Sarker, Citation2014). The good performance of these materials depends on the properties such as grain size, quality, and perfection. The properties of cobalt vanadate (CoV2O6) are governed by the condition of synthesis and subsequent processes.
The various efforts have been devoted to develop new approaches for the synthesis of CoV2O6 microstructures such as hydrothermal growth, sol–gel, co-precipitation, and solid-state synthesis methods. However, these processes except solid-state reaction route require different conditions or large quantity of solvent and organic materials (Dong, Kumada, Yonesaki, Takei, & Kinomura, Citation2011). In this work, CoV2O6 of high quality as anode materials with high quality and crystallinity have been prepared by solid-state reaction technique.
In this study, we devoted the efforts to prepare good quality crystalline CoV2O6 materials for use as anode in LIBss with high performance. The efficiency of this material as anode depends on the crystallinity, dislocation density, and micro-strain. The performance of this anode material also depends on the activation energy and electrical and ionic conductivity at ambient and high temperature. The purpose of this work is to investigate the temperature dependence of resistivity, activation energy, frequency dependence of impedance, charge capacity, photon wavelength dependence of absorption of the prepared crystalline CoV2O6 materials. The electrochemical study of this material is our next step. This study might play an important role to realize crystalline CoV2O6 as high-efficiency anode material.
2. Experimental
High-purity (99.99%) powders of Co3O4 and V2O5 were used as the starting material for preparation of CoV2O6. Initially, we studied the phase formation employing a thermobalance (TG/DTA 630) in order to establish the procedure for synthesizing CoV2O6. A stoichiometric mixture of Co3O4 and V2O5 was heated in an air atmosphere with a heating program as shown in the inset of Figure . A representative thermogravimetric (TG) curve obtained for the raw material mixture is shown in Figure . From this TG analysis, it was found that the weight loss at 50–600°C is too large. This suggests that at temperature lower than 50°C, the chemical reaction between the raw materials may not be active yet and there is no weight change above 700°C where the phase formation is completed.
Figure 1. Thermogravimetric curve for phase formation process of CoV2O6 sample. The inset represents the heating program employed.
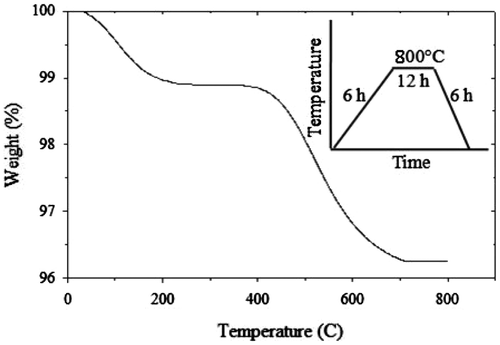
At the first step of synthesis, the reactants were dried in air in an oven at 100°C for 12 h. A stoichiometric mixture of Co3O4 and V2O5 was mixed well in an agate mortar using ethanol, then dried and calcined at 700°C for 24 h in an air atmosphere. Before next heat treatment, the powder was grinded thoroughly to ensure homogeneity. The powder was calcined again second time at 750°C for 12 h in an air atmosphere. After second heat treatment, the powder was grinded and pelletized in 12 mm diameter under pressure of 80 Kilonewton using the pressure gauze. The compressed pellets were sintered at 850°C for 12 h under an air atmosphere. In the all heat treatments, the temperature rising and cooling rates were 3°C/min.
The powder samples were analyzed by X-ray diffraction (XRD) using a CuKα (λ = 0.154 nm) radiation source using a Rigaku HYGD Multiflex X-ray diffractometer at room temperature in the center for crystal science and technology, Japan. The diffraction angle (2θ) range between 5° and 85° was scanned. The morphologies and sizes of the prepared crystalline products were characterized by scanning electron microscopy (SEM). The Fourier transform infrared spectroscopy (FT-IR) spectrums were obtained within the range 200–4,000 cm−1 region on a spectrophotometer (Spectrum 100, Perkin Elmer) using KBr as binders. Resistivity measurements were carried out by the conventional four probe method. Fine polishing was done for the disc-like samples. The Agilent Precision Impedance Analyzer (Agilent technologies, Model 4294A Japan) was used for measurements of frequency-dependent ac conductance, impedance, dielectric constant, capacitance and inductance. The samples were characterized by UV–visible spectrophotometer (Shimizu UV–1,650 PC) for wavelength-dependent absorption spectrum.
3. Results and discussion
3.1. Structural properties
Crystalline CoV2O6 powder was obtained by conventional solid-state reaction technique with a final firing at 850°C. The prepared CoV2O6 crystalline powders were characterized at the room temperature by a Rigaku Multiflex diffractometer range from 2θ = 5–85° with CuKα radiation (λ = 1.5418Å) at 40 kV and 30 mA. The unit cell refinement was accomplished by CellCal software using XRD data as input. Typical XRD patterns of CoV2O6 crystalline powder shown in Figure reveals that the material is single phase with monoclinic crystal structure of space group C2/m. The prepared samples were identified as highly crystalline and homogeneous by indexing these XRD patterns using JCPDS data No. 38–0090. No peaks of other phases have been detected, indicating high purity of the material. The sharp peaks indicate the good crystallinity whish shows that high-quality CoV2O6 crystals can be synthesized fast by conventional solid-state reaction route. The good crystallinity of CoV2O6 crystals would improve the rate capability of Li-ion cells (Minakshi, Singh, Appadoo, & Martin, Citation2011).
In the XRD patterns, (201) peak indicates the property of brannerite-type structure of CoV2O6, (021) peaks indicates the property of basic unit of V–O–V bond, and the intensity ratio of (201)/(021) peaks indicates the perfection of crystallization (Guo-rong et al., Citation2004). The larger value of intensity ratio demonstrates the better crystallinity (Liu et al., Citation2002). The lattice parameters obtained from indexing of XRD pattern using CellCal program as follows: a = 7.76Å, b = 7.41Å, c = 6.78Å, β = 110.62, and V = 365.049Å3. The calculated lattice parameters are approximately equal to the standard lattice constants obtained from the stated JCPDS data. The anion ordering in the crystal structure of anode material is one of the most important factors for good performance that is measured by the intensity ratio of the main peaks in the XRD patterns. Here, the intensity ratio of the highest peaks and second largest peak is 2.69 that are the largest among the reported results. This value represents the highest degree of anion ordering in the crystal structure of prepared cobalt vanadate. It has been proposed that the electrochemical performance of CoV2O6 anode material is remarkably improved when the intensity ratio of (201)/(021) peaks is higher than 1.2 (Hao, Lai, Xu, Liu, & Ji, Citation2005). In the XRD pattern (−201)−(200) and (110)−(210) peak doublets are well defined and separated. This suggests a dimensionally stable structure with a highly ordered distribution of anions in the lattice.
The performance of the lithium ion battery is affected by a numerous factors such as anode and cathode particle size (D), microstrain (ε), and dislocation density (δ) (Yilmaz, Aydin, Turgut, Dilber, & Ertugrul, Citation2012). The average grain size (D) and microstrain (e) of the CoV2O6 crystalline particles were calculated using Scherrer formulae, Yilmaz, Turgut, Aydin, and Ertugrul Citation(2012) and (Rajasekar, Kungumadevi, Subbarayan, and Sathyamoorthy (Citation2008), respectively, where λ is the X-ray wavelength (1.5418Å), 0.94 is the crystal shape constant, θ is the reflection angle for the highest peak, and β is the full width at half maximum (FWHM) of the highest peak in radians. The dislocation density (δ) of the crystalline CoV2O6 was obtained using the equation δ = 1/D2 (lines/m2) (Ravichandran, Muruganantham, & Sakthivel, Citation2009). The calculated values are dislocation density δ = 8.206 × 1014 lines/m2, microstrain ε = 1.3 × 10−3 lines.m−4 and average particle size D = 110.4 nm.
The dislocation density (δ) indicates the length of dislocation lines per unit volume and measures the amount of defects in a crystal. The microstrain (ε) indicates strain induced in the crystalline materials that also measures the amount of defects. Here, the microstrain is very low and it means the quality of prepared CoV2O6 crystal was very high. The average grain size of the crystalline material is the most important factor for its applications. Here, calculated average grain size of the prepared crystalline CoV2O6 is 110.4 nm that is very large and hence this crystal is suitable for use as anode material in the high-efficiency Li-ion batteries.
In order to get physical insights of the chemical bonding and phase formation, we also studied the structure by FT-IR spectroscopy. FT-IR spectroscopic technique is powerful for investigating the local structure and cation environment in oxides (Amdouni, Citation2003; Julien, Citation2000). The spectroscopic spectrum of crystalline CoV2O6 is shown in the Figure that contains the main absorption bands at: 279, 390, 528, 847, 1,010, 1,103, 1,385, 1,638, 3,411, 3,806, 3,853, and 3,951 cm−1. These results confirm the XRD observations showing that the vibration bands for precursors vanished and the vibration bands for the oxide network developed.
In the far-infrared region, we observed the single well-resolved band at 279 cm−1. This sharp band in CoV2O6 is assigned due to an asymmetric stretching motion of the CoO6 octahedron that is highly distorted (Kim et al., Citation2012). It is the unique finger print of the Co site occupancy in the monoclinc structure of CoV2O6 crystal. The absorption bond at 847 and 552 cm−1 can be attributed to the symmetric and asymmetric stretching of VO5 units, respectively (Tang, Zhou, Liu, Liu, & Liang, Citation2013). The absorption bands at 854 cm−1 of CoV2O6 may be assigned to V = O stretching mode (Song et al., Citation2009). The absorption bands in the region of 500–700 cm−1 are due to the V–O–V bonds corresponding to the asymmetric or symmetric stretches (Gao, Ruiz, Xin, Guo, & Delmon, Citation1994) and the bands at 386 cm−1 can be assigned to the stretching of Co–O modes. There is a sharp medium absorption peaks around 1,104 cm−1, which is due to the vibrations of the cobalt vanadate crystal lattice. The results of FT-IR analysis were good agreement with XRD results.
The morphologies of the as-prepared CoV2O6 crystalline materials were examined by SEM. Figure shows the typical SEM micrographs of the CoV2O6 crystalline materials with different magnifications. The SEM images reveal that the CoV2O6 crystals consists of large grain size of about 2–10 μm, mixed with some small particles of sizes about 500 nm. The SEM images also reveal that the particles are very uniform and their surfaces are smooth. The particles are well separated from each other. Therefore, ample space between the particles can be clearly observed. The prepared CoV2O6 material with highly crystalline large size particles was expected to have better electrochemical performances.
3.2. Electronic properties
The temperature-dependent dc resistivity of the samples was measured using four probe techniques from room temperature to 500 K as shown in Figure . The obtained results reveal that the resistivity of crystalline CoV2O6 decrease with temperature and it was signature of the semiconducting behavior. At ambient pressure, ρ(T) of crystalline CoV2O6 materials exhibits semiconducting-like behavior in which dρ/dT < 0 over the entire range of temperature. The variation of ln of conductivity (lnσ) with inverse temperature (K−1) for CoV2O6 crystals have been shown in Figure . The activation energy can be obtained using Arrhenius relation σ = σ0 exp(−δE/2KBT), where δE is the activation energy, KB in the Boltzmann constant, and σ0 is the pre-exponential factor. From slope of the lns vs. K−1 plot, the activation energy was calculated at low- and high-temperature region (Sivaprakash, Majumder, Nieto, & Katiyar, Citation2007). The activation energy δE2 = 0.15 eV is higher than that of δE1 = 0.013 The higher values indicate that conductivity is associated with the free band transition of carriers, i.e. transition from donor level to conduction band and the low values of δE1 may be associated with the localized levels hopping due to the excitation of carriers from one defect state to another.
The frequency-dependant impendent was measured by impedance analyzer, frequency was varied from 100 Hz to 10 MHz and oscillatory voltage was 300 mV. Figure (a) shows the frequency-dependent impedance of CoV2O6 crystals. Figure shows two regions, high-frequency and low-frequency regions. At high frequencies, the real part of the impedance is almost independent of the frequency, which is attributed to the resistance effect. In the low-frequency regions, impedance considerably decreases as the frequency increases. This indicates that the components of capacitance and resistance of the equivalent element is active in this region.
Figure 7. Frequency-dependent (a) impedance, (b) capacitance, (c) dielectric constant (d), and magnetic induction of the CoV2O6 sample.
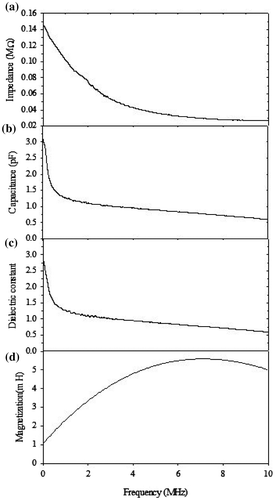
The capacitance and dielectric properties of the samples were measured by precision impedance analyzer at the same condition as impedance measurement. Silver paste was coated on both surface of the sample before measurement. Dielectric constant is a measure of materials ability to store electric charge. The frequency-dependent capacitance of samples measured at room temperature is shown in Figure (b). The capacitance of CoV2O6 crystal is high at low-frequency region due to contribution of all kinds of polarization at low frequency, then decreases with increase of frequency and finally approaches to all most constant value above 8 MHz. This is due to the change of space charge, ionic and orientation polarization at higher frequencies (Kingegy, Citation1960). Figure (c) shows the frequency-dependent dielectric constant of the CoV2O6 crystals that implies the same tendency of capacitance. Figure (d) shows the frequency-dependent magnetic induction of the CoV2O6 crystals at the room temperature. Initially, the magnetic induction increases with frequency and then decreases. This plot shows that the sample was free of magnetic impurities.
3.3. Optical properties
The optical properties of a material are very important in some applications such as optical coatings, reflectors, absorbers, and various optoelectronic devices. The reported results on optical absorption or transmission of CoV2O6 crystals are not available. In this study, UV–visible absorption spectrum of the CoV2O6 crystals was recorded using a UV–visible spectrophotometer (Shimazu: UV-1650 PC) in the photon wavelength range 200–800 nm. Figure shows the absorption spectrum of CoV2O6 crystals. The absorption slightly increases with increase of wavelength in the visible region. There are some absorption peaks in the ultraviolet region. There are two very strong absorption peaks at 200 and 206 nm and a medium peak at 209 nm. These peaks reveal the absorption criteria of cobalt vanadate in the ultraviolet–visible region.
4. Conclusions
In this study, cobalt vanadate (CoV2O6) crystalline materials of high crystallinity and high purity have been successfully prepared by solid-state reaction technique. This technique is most effective to grow high-quality crystalline material at required high temperature. The CoV2O6 poly crystals were obtained after two times calcinations at 700 and 750°C. Dense crystalline pellets were obtained by sintering at 750°C and its structural, electrical, and optical properties were studied. From structural properties and crystal quality studies, it has been assessed that high-quality CoV2O6 was successfully synthesized. We want to propose here that high-quality CoV2O6 crystals prepared by solid-state reaction technique may enhance the efficiency of Li-ion batteries. The charge capacity and dielectric constant of CoV2O6 crystal is high at room temperature but activation energy is high at above the room temperature. The CoV2O6 crystal might be a weak absorber at the ultraviolet region. In the next stop, we want to investigate the electrochemical properties of this material for battery applications.
Funding
This work was partially supported by the Rajshahi University Research [grant number A774] and University Grand Commission, Bangladesh.
Acknowledgments
Authors would like to thanks Rajshahi University, Rajshahi, Bangladesh authority for providing funds under the University Research Grant. Authors also would like to thanks Ministry of Science and Technology, Govt. of Bangladesh for providing scholarship to carry out this work.
Additional information
Notes on contributors
Md. Abdur Razzaque Sarker
The author and co-authors of this article are engaged in teaching and research in the recognized public universities in Bangladesh. They are working in a research topic related with synthesis and characterization of some functional oxide materials. The aim of this group is the synthesis of crystalline functional materials for various device applications such as rechargeable batteries, thermoelectric power generator, high-performance capacitors, and optical polarizer. This work is about the synthesis of good quality crystalline anode materials for rechargeable Li-ion batteries.
References
- Amdouni, N. (2003). Structural and electrochemical properties of LiCoO2 and LiAlyCo1−yO2 (y=0.1 and 0.2) oxides: A comparative study of electrodes prepared by the citrate precursor route. Ionics, 9, 47–55.10.1007/BF02376536
- Catti, M. (2000). Ab initio study of Li+ diffusion paths in the monoclinic Li 0.5 CoO 2 intercalate. Physical Review B, 61, 1795–1803. doi:10.1103/PhysRevB.61.1795
- Dong, Q., Kumada, N., Yonesaki, Y., Takei, T., & Kinomura, N. (2011). Synthesis of LiCoO2 via a facile hydrothermal-assisted route. Journal of the Ceramic Society of Japan, 119, 538–540.10.2109/jcersj2.119.538
- Fan, C. L., & Chen, H. (2011). Preparation, structure, and electrochemical performance of anodes from artificial graphite scrap for lithium ion batteries. Journal of Materials Science, 46, 2140–2147.10.1007/s10853-010-5050-y
- Gao, X. G., Ruiz, P., Xin, Q., Guo, X. X., & Delmon, B. (1994). Preparation and characterization of three pure magnesium vanadate phases as catalysts for selective oxidation of propane to propene. Catalysis Letters, 23, 321–337. 10.1007/BF00811367
- Goodenough, J. B., & Kim, Y. (2010). Challenges for rechargeable Li batteries. Chemistry of Materials, 22, 587–203.10.1021/cm901452z
- Guo-rong, H., Gang, L., Zhong-dong, P., Jin, X., Xin-lon, Z., & Xiao-yuan, Y. (2004). Structure and electrochemical properties of LiCoO2 synthesized by microwave heating. Journal of Central South University of Technology, 11, 261. doi:10.1007/s11771-004-0053-y
- Hao, Y., Lai, Q., Xu, Z., Liu, X., & Ji, X. (2005). Synthesis by TEA sol–gel method and electrochemical properties of LiTiO anode material for lithium-ion battery. Solid State Ionics, 176, 1201–1206. doi:10.1016/j.ssi.2005.02.010
- Jiang, J., Li, Y. Y., Liu, J. P., & Huang, X. T. (2011). Building one-dimensional oxide nanostructure arrays on conductive metal substrates for lithium-ion battery anodes. Nanoscale, 3, 45–58.10.1039/C0NR00472C
- Julien, C. (2000). Local cationic environment in lithium nickel-cobalt oxides used as cathode materials for lithium batteries. Solid State Ionics, 136–137, 887–896.
- Khatun, F., Gafur, M. A., Ali, M. S., Islam, M. S., & Sarker, M. A. R. (2014). Impact of lithium composition on structural, electronic and optical properties of lithium cobaltite prepared by solid state reaction. Journal of Science Research, 6, 214–231.
- Kim, B., Kim, B. H., Kim, K., Choi, H. C., Park, S. Y., Jeong, Y. H., & Min, B. I. (2012). Unusual magnetic properties induced by local structure in a quasi-one-dimensional Ising chain system: α-CoV2O6. Physical Review B, 85, 220 407 (R)–220 413 (R).10.1103/PhysRevB.85.220407
- Kingegy, W. D. (1960). Introduction to ceramic. New York, NY: Wiley.
- Koksbang, R., Barker, J., Shi, H., & Saidi, M. Y. (1996). Cathode materials for lithium rocking chair batteries. Solid State Ionics, 84, 1–21. doi:10.1016/S0167-2738(96)83001-3
- Li, H., Wang, Z. X., Chen, L. Q., & Huang, X. J. (2009). Research on advanced materials for Li-ion batteries. Advanced Materials, 21, 4593–4607.10.1002/adma.v21:45
- Liu, J., Wen, Z. Y., Wu, M. M., Gu, Z. H., Chao, J. D., & Lin, Z. X. (2002). Synthesis by a complexation route and characterization of LiCoO2 cathode materials. Journal of Inorganic Materials, 17, 1157–1162.
- Ma, H., Zhang, S. Y., Ji, W. Q., Tao, Z. L., & Chen, J. (2008). α-CuV2O6 nanowires: Hydrothermal synthesis and primary lithium battery application. Journal of the American Chemical Society, 130, 5361–5367.10.1021/ja800109u
- Mai, L. Q., Xu, L., Gao, Q., Han, C. H., Hu, B., & Pi, Y. Q. (2010). Single β-AgVO3 nanowire H2S sensor. Nano Letters, 10, 2604–2608.10.1021/nl1013184
- Minakshi, M., Singh, P., Appadoo, D., & Martin, D. E. (2011). Synthesis and characterization of olivine LiNiPO4 for aqueous rechargeable battery. Electrochimica Acta, 56, 4356–4360.10.1016/j.electacta.2011.01.017
- Myung, S. T., Amine, K., & Sun, Y. K. (2010). Surface modification of cathode materials from nano- to microscale for rechargeable lithium-ion batteries. Journal of Materials Chemistry, 20, 7074–7095.10.1039/c0jm00508h
- Rajasekar, K., Kungumadevi, L., Subbarayan, A., & Sathyamoorthy, R. (2008). Thermal sensors based on Sb2Te3 and (Sb2Te3)70(Bi2Te3)30 thin films. Ionics, 14, 69–72. doi:10.1007/s11581-007-0146-3
- Ramkumar, R., & Minakshi, M. (2015). Fabrication of ultrathin CoMoO4 nanosheets modified with chitosan and their improved performance in energy storage device. Dalton Transactions, 44, 6158–6168.10.1039/C5DT00622H
- Ravichandran, K., Muruganantham, G., & Sakthivel, B. (2009). Highly conducting and crystalline doubly doped tin oxide films fabricated using a low-cost and simplified spray technique. Physica B: Condensed Matter, 404, 4299–4302. doi:10.1016/j.physb.2009.08.017
- Shi, H. F., Li, Z. S., Kou, J. H., Ye, J. H., & Zou, Z. G. (2011). Facile synthesis of single-crystalline Ag2V4O11 nanotube material as a novel visible-light-sensitive photocatalyst. The Journal of Physical Chemistry C, 115, 145–151.10.1021/jp102680y
- Sivaprakash, S., Majumder, S. B., Nieto, S., & Katiyar, R. S. (2007). Crystal chemistry modification of lithium nickel cobalt oxide cathodes for lithium ion rechargeable batteries. Journal of Power Sources, 170, 433–440. doi:10.1016/j.jpowsour.2007.04.029
- Song, J. M., Lin, Y. Z., Yao, H. B., Fan, F. J., Li, X. G., & Yu, S. H. (2009). Superlong β-AgVO3 nanoribbons: High-yield synthesis by a pyridine-assisted solution approach, their stability, electrical and electrochemical properties. ACS Nano, 3, 653–660.10.1021/nn800813s
- Song, H. K., Lee, K. T., Kim, M. G., Nazar, L. F., & Cho, J. (2010). Recent progress in nanostructured cathode materials for lithium secondary batteries. Advanced Functional Materials, 20, 3818–3834.10.1002/adfm.201000231
- Tang, Y., Zhou, J., Liu, J., Liu, L., & Liang, S. (2013). Facile synthesis of cobalt vanadium oxides and their applications in lithium ion batteries. International Journal of Electrochemical Science, 8, 1138–1145.
- Tarascon, J. M., & Armand, M. (2001). Issues and challenges facing rechargeable lithium batteries. Nature, 414, 359–367.10.1038/35104644
- Thackeray, M. M. (1995). Structural considerations of layered and spinel lithiated oxides for lithium ion batteries. Journal of The Electrochemical Society, 142, 2558–2563. doi:10.1149/1.2050053
- Xu, A. J., Lin, Q., Jia, M. L., & Zhaorigetu, B. (2008). Studies on the oxidative dehydrogenation of propane to propene over Co-V-O catalysts. Reaction Kinetics and Catalysis Letters, 93, 273–280.10.1007/s11144-008-5220-y
- Yilmaz, M., Aydin, S., Turgut, G., Dilber, R., & Ertugrul, M. (2012). Preparation of LiCoO2 and LiNixCo1-xO2 by solid state reaction technique. Progress in Nanotechnology and Nanomaterials, 1, 5–8.
- Yilmaz, M., Turgut, G., Aydin, S., & Ertugrul, M. (2012). LiCoO2 structures by spray pyrolysis technique for rechargeable Li-ion battery. Journal of the Chemical Society of Pakistan, 34, 283–286.
- Yoshino, A. (2012). The birth of the lithium ion battery. Angewandte Chemie International Edition, 51, 5798–5800.