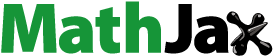
Abstract
Chitosan–transition metal compounds with Mn2+, Co2+, Ni2+, Zn2+, and Cd2+ were obtained containing 0–4.0% weight fraction of metal ions. The microstructure of these compounds was determined, and the effect of the metal ions on the thermal degradation of chitosan in nitrogen was studied. The results showed that the second thermal degradation of chitosan was significantly affected by the metal ion species. The temperature of maximal weight loss rate was dependent on the content of the metal ion, with a higher metallic ion concentration sample presenting a lower temperature, and also the metal ion species, which decreased in the order Cd2+ > Ni2+ > Mn2+ > Co2+ > Zn2+. However, the weight loss, which decreased with an increasing weight fraction of the metal ion, was not proportional to the initial metal concentration in the compound. The activation energies of the second thermal degradation of the compounds were calculated and compared.
Public Interest Statement
Chitosan is a natural polymer of 2-amino-2-deoxy-d-glucose and 2-acetylamino-2-deoxy-d-glucose units. It has reactive amine and hydroxy side groups, so that chitosan and its derivatives have stable coordination with metal ions. Chitosan–metal ion complexes have many applications. But the thermal stability of chitosan is bad, and the effect of metal ion on the degradation of chitosan is obvious. Therefore, knowledge of the effect of various metal ions on the thermal decomposition reactions of chitosan is essential.
In the work reported here, the thermal degradation of chitosan–transition metal compounds with Mn2+, Co2+, Ni2+, Zn2+, and Cd2+ was investigated, and the reaction activation energies were evaluated. The effect of the metal ions on the thermal degradation of chitosan is discussed in detail. This paper also attempts to correlate the thermal degradation of the compounds with the nature of a series of transition metal ions.
1. Introduction
Chitosan is a natural polymer of 2-amino-2-deoxy-d-glucose and 2-acetylamino-2-deoxy-d-glucose units (Signini, Citation1999). It has reactive amine and hydroxy side groups, which permit the formation of the cage form of a net structure through hydrogen bonds or salt bonds, so that chitosan and its derivatives have stable coordination with metal ions (Guibal, Citation2004; Varma, Deshpande, & Kennedy, Citation2004). Chitosan–metal ion complexes have many applications: for example, in wastewater treatment (Zamariotto, Lakard, Fievet, & Fatin-Rouge, Citation2010), in chemical catalysis (Martina et al., Citation2011), and in medicine through their anticancer (Karagozlu & Kim, Citation2014) and antibacterial activities (Higazy, Hashem, ElShafei, Shaker, & Hady, Citation2010). In recent years, much effort has been devoted to new developments from chitosan by the activation of salts. Bengisu and Yilmaz (Citation2002) suggested that the oxidation and pyrolysis of chitosan after pretreatment with ammonium chloride may provide a source of carbon fibers, and metals such as copper and nickel can be used as catalysts in this process (Kim, Rodriguez, & Baker, Citation1991). Wang, Christian, Deng, Qiao and Hou (Citation2013) used a concentrated aqueous solution of ZnCl2 to directly convert chitin biomass to 5-hydroxymethylfurfural. Shao et al. (Citation2015) considered chitosan to be one of the most promising precursors of N-doped carbon, and metal salts such as nickel nitrate and copper nitrate are able to promote the conversion of chitosan (Ilnicka, Walczyk, & Lukaszewicz, Citation2015; Liang et al., Citation2014).
It is well known that the coordination of the amino groups in chitosan chains with metal ions affect the conformation of the polymer and, consequently, its thermal stability (Sreenivasan, Citation1996). Therefore, knowledge of the effect of various metal ions on the thermal decomposition reactions of chitosan is essential. Trimukhe and Varma (Citation2009) reported on the correlation between the thermal degradation of metal complexes of cross-linked chitosans and their saturated heavy metal ion complexation values. Taboada, Cabrera, Jimenez and Cardenas (Citation2009) studied the thermal behavior of metal complexes formed by chitosan with Cu2+, Ni2+, Co2+, and Hg2+ at different metal concentrations. However, the thermal degradation of chitosan catalyzed by metal ions is influenced by the species and concentration of the metal ions, the structure of the complex, and the interaction strength of the polymer with the metal ions. There are, unfortunately, few reports on the specific effect of different metal ions on the thermal properties of the polymer.
The effects of the cupric ion on the thermal degradation of chitosan and quaternized chitosan have been studied by the authors (Li et al., Citation2010; Ou et al., Citation2008; Ou, Yang, Li, Hong, & She, Citation2010), who subsequently investigated the effect of other transition metal ions on the thermal degradation of chitosan. In the work reported here, the thermal degradation of chitosan–transition metal compounds with Mn2+, Co2+, Ni2+, Zn2+, and Cd2+ was investigated, and the reaction activation energies evaluated. The effect of the metal ions on the thermal degradation of chitosan is discussed in detail below. This paper also attempts to correlate the thermal degradation of the compounds with the nature of a series of transition metal ions.
2. Experimental
2.1. Materials
Chitosan was bought from Shanghai Greenbird Science and Technology Development Co. (Shanghai, China). Its viscosity average molecular weight was 180 kDa, and the degree of deacetylation of chitosan was greater than 90%.
2.2. Preparation of chitosan–transition metal compounds
The chitosan–transition metal compounds were obtained by mixing an aqueous solution of the metal chloride with a chitosan solution in 1% acetic acid, casting, and then drying. The products so obtained were transparent and homogeneous films containing 0–4% weight fraction of the transition metal ion.
The samples prepared were pure chitosan (CTS), CTS–Mn compounds (CTS–Mn-1, Mn2+/CTS = 1%; CTS–Mn-2, Mn2+/CTS = 2%; CTS–Mn-3, Mn2+/CTS = 3%; CTS–Mn-4, Mn2+/CTS = 4%), CTS–Co compounds (CTS–Co-1, Co2+/CTS = 1%; CTS–Co-2, Co2+/CTS = 2%; CTS–Co-3, Co2+/CTS = 3%; CTS–Co-4, Co2+/CTS = 4%), CTS–Ni compounds (CTS–Ni-1, Ni2+/CTS = 1%; CTS–Ni-2, Ni2+/CTS = 2%; CTS–Ni-3, Ni2+/CTS = 3%; CTS–Ni-4, Ni2+/CTS = 4%), CTS–Zn compounds (CTS–Zn-1, Zn2+/CTS = 1%; CTS–Zn-2, Zn2+/CTS = 2%; CTS–Zn-3, Zn2+/CTS = 3%; CTS–Zn-4, Zn2+/CTS = 4%), and CTS–Cd compounds (CTS–Cd-1, Cd2+/CTS = 1%; CTS–Cd-2, Cd2+/CTS = 2%; CTS–Cd-3, Cd2+/CTS = 3%; CTS–Cd-4, Cd2+/CTS = 4%).
2.3. Attenuated total reflectance-Fourier transform infrared (ATR-FTIR) spectroscopy
FTIR spectra were recorded on a Nicolet 5700 instrument with an ATR accessory. First, the crystal area was cleaned and the background was collected, and sufficient solid material was then placed to just cover the crystal area. Next, the pressure arm was positioned over the crystal/sample area, and force was applied to the sample. The FTIR spectrum of the sample was obtained using a resolution of 16 cm−1 and 32 scans ranging from 500 to 4,000 cm−1.
2.4. Powder X-ray diffraction
Powder X-ray diffraction (PXRD) was carried out on the freshly prepared sample using a D/max IIIA diffractometer (Rigaku, Japan) and a Cu Kα target. The standard run was carried out using a voltage of 40 kV, a current of 100 mA, and a scanning rate of 8°min−1 over a 2θ range of 2°–60° with a step size of 0.04° and a counting time of 0.5 s step−1.
2.5. Thermal analysis
Thermogravimetric analysis (TGA) was undertaken using a Perkin Elmer TG/DTA6300 analyzer (USA). The accuracy of the measured sample temperature was checked by the onset fusion temperatures of indium (156.6 ± 0.2°C) and tin (232 ± 0.2°C) with heating/cooling dynamic segments. The sample (5 ± 0.1 mg) was heated in an alumina crucible, and the loss of mass as a function of temperature was recorded from room temperature to 800°C. The experiment was performed at a heating rate of 10°C min−1, using a nitrogen flow rate of 50 ml min−1. All the experiments were carried out in triplicate.
2.6. Data processing
The kinetic parameters of the thermal decomposition reactions were evaluated from dynamic experiments in which a sample was heated from room temperature until its complete decomposition at a linearly programed rate. The method used to investigate the effect of the cupric ion on the thermal degradation of chitosan was also used in this work (Ou et al., Citation2008):(1)
(1)
where α is the extent of the decomposition, Eα is the activation energy (kJ mol−1), T is the absolute temperature (K), is the temperature of the maximum reaction velocity (K), u is the heating rate (K min−1), R is the gas constant (8.314 J mol−1 K−1), and Z is a constant.
The activation energy was determined from the slope of the straight line that resulted from plotting ln { ln [1/(1 − α)]} against 1/T.
3. Results and discussion
3.1. FTIR analysis
Figure S1 (Supporting Information) shows the FTIR spectra of the samples, and Table lists the characteristic FTIR bands of the samples. The main bands appearing in the spectrum of chitosan were due to axial stretching vibration of O–H superimposed on the N–H stretching band and the interhydrogen bonds of the polysaccharide in the range 3,750–3,000 cm−1, and the C–H bond in –CH2 (2,930 cm−1) and –CH3 (2,875 cm−1) groups (Paluszkiewicz, Stodolak, Hasik, & Blazewicz, Citation2011). The other bands observed in the vibration spectrum of chitosan were C=O stretching of the amide group coupled with N–H bending (1,613 cm−1) (Marchessault, Ravenelle, & Zhu, Citation2006), C–N stretching coupled with N–H plane deformation (1,406 cm−1) (Antony, Theodore David Manickam, Saravanan, Karuppasamy, & Balakumar, Citation2013), C–O–C bridge stretching vibration (1,024 cm−1), and the specific bands of the β-(1–4) glycoside bridge (1,133 and 875 cm−1) (Antony, Theodore David, Saravanan, Karuppasamy, & Balakumar, Citation2013). The absorption band at 706 cm−1 resulted from N–H plane bending vibration (Yin, Yuan, Lin, Tan, & Zhang, Citation2006).
Table 1. Characteristic FTIR bands of chitosan–transition metal compounds
In the FTIR spectra of the compounds, it was anticipated that coordination of the nitrogen center with the metal ion would reduce the electron density in the amino group and shifts the frequencies of N–H stretching and N–H plane bending to lower wave numbers (Thangadurai & Ihm, Citation2003). The shift in N–H stretching and N–H plane bending to lower regions was found for all the compounds, indicating successful coordination of this amino nitrogen with the metal center. The characteristic bands of chitosan, the C–O–C stretching band (at around 1,030 cm−1), and β-(1–4) glycoside bridge bands (at around 1,135 and 870 cm−1) were also seen in the FTIR spectra of the compounds, which indicated that the metal complexes had successfully anchored to the chitosan backbone (Antony, Theodore David et al., Citation2013). However, the decrease in the wave numbers and the change in the absorption intensities were not proportional to the fraction of the transition metal ion in the chitosan, which may be a result of variation in the coordination behaviors for the different transition metal compounds.
3.2. PXRD studies
Figure S2 (Supporting Information) shows the PXRD patterns of the chitosan–transition metal compounds. The PXRD pattern of chitosan exhibited three characteristic peaks at 2θ of 11.3°, 17.8°, and 22.8°. These three peaks gradually became weaker or were disappeared for the chitosan–transition metal compounds as the weight fraction of the transition metal ion in the chitosan increased. These results indicate that the chitosan–transition metal complexes formed were amorphous because of the interaction of the amino group in chitosan with the transition metal ions, which resulted in their thermal instability.
3.3. Thermal analysis
The thermal properties of the chitosan–transition metal compounds were studied by TGA under a nitrogen atmosphere. In general, it has been found that the coordination of a metal ion with the functional group of the chitosan moiety causes thermal instability and affects thermal degradation (Trimukhe & Varma, Citation2009). In this work, the effect of transition metal ions on the thermal degradation and stability of chitosan was studied in detail, and different metal ion species compared. The TG curves and the corresponding DTG curves of chitosan–transition metal compounds are shown in Figure S3 (Supporting Information). As can be seen, there were two peaks in the DTG curves for all the samples studied, showing their two distinct decomposition stages.
The first peak appeared at about 100°C, which Bihari-Varga, Sepulchre and Moczár (Citation1975) explained as resulting from the evaporation of water (this stage will be ignored in the following discussion). The second peak, corresponding to the main degradation of chitosan, occurred in the temperature range 200–350°C. From the TG and DTG curves, this stage began at lower temperatures for the chitosan–transition metal compounds compared with chitosan, indicating that these compounds were less thermally stable than chitosan. The characteristic temperatures and the corresponding mass losses in the main degradation stage for all samples are listed in Table . The data in Table show that the temperature at which the maximum reaction rate occurred was dependent on the content of the transition metal ion (with a high-metallic ion concentration resulting in a lower temperature) and on the transition metal ion species (which decreased the temperature in the following order: Cd2+ > Ni2+ > Mn2+ > Co2+ > Zn2+). However, the weight loss in the second stage of degradation, which decreased with an increasing weight fraction of the metal ion, was not proportional to the initial metal concentration in the compound, which may be due to the formation of a thermally stable metal oxide. These results are consistent with the conclusion of Taboada et al. (Citation2009).
Table 2. Characteristic temperatures and the extent of decomposition for the main thermal degradation of chitosan–transition metal compounds
The apparent activation energies (Eα) for the second thermal degradation of the chitosan–transition metal compounds in nitrogen were calculated from the TG curves using Equation (1). Plots of ln { ln [1/(1 − α)]} vs. 1/T are shown in Figure S4 (Supporting Information).
It can be seen that there were three distinct degradation periods. The Eα obtained for the three degradation periods is listed in Table . In period I (225–252°C), the deacetylation of chitosan occurred (Debritto & Campana-Filho, Citation2004): Eα increased with an increasing weight fraction of the metal ion. Period II (252–280°C) was the cleavage of the glycosidic linkages in chitosan (Holme, Foros, & Pettersen, Citation2001): a decrease in Eα was observed with an increasing amount of the metal ion. In period III (280–350°C), further cleavage of the glycosidic linkages of chitosan occurred: Eα decreased slightly with an increasing weight fraction of the metal ion.
Table 3. Activation energies during the second thermal degradation stage of chitosan–transition metal compounds
These results indicated that the conformation change in chitosan resulted from the mixing of the metal ion in chitosan, and had an effect on the formation of the new phase of the polymer, which partly decreased the bond energies of the glycosidic linkages in chitosan, consequently leading to its thermal instability. This conclusion is in close agreement with that of Sreenivasan (Citation1996). When the activation energies of the chitosan–transition metal compound were compared at the same concentration, it was observed that they decreased in the order Cd2+ > Ni2+ > Mn2+ > Co2+ > Zn2+, indicating that the order of thermal stability was Cd2+ < Ni2+ < Mn2+ < Co2+ < Zn2+ in the thermal degradation of chitosan.
Pearson’s principle of hard and soft acids and bases states that hard acids bind strongly to hard bases, and that soft acids bind strongly to soft bases (Pearson, Citation1963). In accordance with this theory, transition metal complexes are stable in the presence of typical soft ligands (Chatt & Shaw, Citation1959).
Chitosan coordinates with a metal ion via the hard base amino group, according to FTIR analysis. Therefore, the chitosan–metal complex has less thermal stability than chitosan. The greater the hardness of the metallic ion, the stronger is its interaction with the ligand group, and the smaller is the apparent activation energy of the degradation process. Cd2+ is a soft acid, while Co2+, Ni2+, and Zn2+ are borderline cases (Holme et al., Citation2001), and was observed that the effect of Ni2+, Mn2+, Co2+, and Zn2+ on the pyrolysis process of the polymer matrix was stronger than that of Cd2+.
4. Conclusion
(1) | Chitosan–transition metal compounds with Mn2+, Co2+, Ni2+, Zn2+, and Cd2+ were obtained by casting solutions in the form of transparent films containing 0–4.0% weight fraction of transition metal ions. | ||||
(2) | The microstructure of the compounds was characterized by FTIR and PXRD. A shift of N–H stretching and N–H plane bending to lower spectral regions was observed, and XRD peaks became gradually weaker or disappeared with an increasing weight fraction of transition metal ions in chitosan, which indicated coordination of the amino nitrogen of chitosan with the metal center to form amorphous complexes. | ||||
(3) | The thermal degradation of the compounds in nitrogen was studied by TGA from room temperature to 800°C, and the effect of transition metal ions on the thermal degradation of chitosan was investigated. The results showed that the thermal degradation of the compounds could be divided into two distinct stages. The effect of transition metal ions on the second thermal degradation stage of chitosan was notable. The temperature at which the maximum weight loss rate occurred was dependent on the transition metal ion content (a high metallic ion concentration resulted in a lower temperature) and on the transition metal ion species (which decreased the temperature in the order Cd2+ > Ni2+ > Mn2+ > Co2+ > Zn2+). However, the weight loss—which decreased as the weight fraction of metal ion increased—was not proportional to the initial metal concentration in the compound. | ||||
(4) | The activation energies of the main thermal degradation of the compounds were calculated and compared, and showed that the effect of the thermal degradation for chitosan was Cd2+ < Ni2+ < Mn2+ < Co2+ < Zn2+. |
Funding
The authors gratefully acknowledge the financial support of the National Natural Science Foundation of China [grant number 51403104], [grant number 81274095], [grant number 31271938], the Natural Science Foundation of Jiangsu Province [grant number BK20131414], [grant number BK20141465], and the Priority Academic Program Development of Jiangsu Higher Education Institutions [grant number TAPP-PPZY2015A070].
Supplementary material
The supplementary material for this paper is available online at http://dx.doi.org/10.1080/23312009.2016.1216247.
supporting_information.docx
Download MS Word (1.1 MB)Additional information
Notes on contributors
Chunyan Ou
Chunyan Ou, specializing in applied chemistry, has engaged in the extraction, modification of chitosan, and its structure characterization. At present, the author’s group is beginning to do the research about the effect of transition metal ion on the thermal degradation of chitosan, which is supported from the National Natural Science Foundation of China.
References
- Antony, R., Theodore David, S., Saravanan, K., Karuppasamy, K., & Balakumar, S. (2013). Synthesis, spectrochemical characterisation and catalytic activity of transition metal complexes derived from Schiff base modified chitosan. Spectrochimica Acta Part A: Molecular and Biomolecular Spectroscopy, 103, 423–430.10.1016/j.saa.2012.09.101
- Antony, R., Theodore David Manickam, S., Saravanan, K., Karuppasamy, K., & Balakumar, S. (2013). Synthesis, spectroscopic and catalytic studies of Cu(II), Co(II) and Ni(II) complexes immobilized on Schiff base modified chitosan. Journal of Molecular Structure, 1050, 53–60.10.1016/j.molstruc.2013.07.006
- Bengisu, M., & Yilmaz, E. (2002). Oxidation and pyrolysis of chitosan as a route for carbon fiber derivation. Carbohydrate Polymers, 50, 165–175.10.1016/S0144-8617(02)00018-8
- Bihari-Varga, M., Sepulchre, C., & Moczár, E. J. (1975). Thermoanalytical studies on protein-polysaccharide complexes of connective tissues. Journal of Thermal Analysis, 7, 675–683.10.1007/BF01912027
- Chatt, J., & Shaw, B. L. (1959). Alkyls and aryls of transition metals. Part I. Complex methylplatinum(II) derivatives. Journal of the Chemical Society, 705–716. doi:10.1039/JR9590000705
- Debritto, D., & Campana-Filho, S. P. (2004). A kinetic study on the thermal degradation of N,N,N-trimethylchitosan. Polymer Degradation and Stability, 84, 353–361.10.1016/j.polymdegradstab.2004.02.005
- Guibal, E. (2004). Interactions of metal ions with chitosan-based sorbents: A review. Separation and Purification Technology, 38, 43–74.10.1016/j.seppur.2003.10.004
- Higazy, A., Hashem, M., ElShafei, A., Shaker, N., & Hady, M. A. (2010). Development of antimicrobial jute packaging using chitosan and chitosan–metal complex. Carbohydrate Polymers, 79, 867–874.10.1016/j.carbpol.2009.10.011
- Holme, H. K., Foros, H., & Pettersen, H. (2001). Thermal depolymerization of chitosan chloride. Carbohydrate Polymers, 46, 287–294.10.1016/S0144-8617(00)00332-5
- Ilnicka, A., Walczyk, M., & Lukaszewicz, J. P. (2015). The fungicidal properties of the carbon materials obtained from chitin and chitosan promoted by copper salts. Materials Science and Engineering: C, 52, 31–36.10.1016/j.msec.2015.03.037
- Karagozlu, M., & Kim, S. K. (2014). Marine carbohydrates: Fundamentals and applications, Part A. Advances in Food and Nutrition Research, 72, 215–225.10.1016/B978-0-12-800269-8.00012-9
- Kim, M. S., Rodriguez, N. M., & Baker, R. T. K. (1991). The interaction of hydrocarbons with copper-nickel and nickel in the formation of carbon filaments. Journal of Catalysis, 131, 60–73.10.1016/0021-9517(91)90323-V
- Li, S. D., Zhang, C. H., Dong, J. J., Ou, C. Y., Quan, W. Y., Yang, L., & She, X. D. (2010). Effect of cupric ion on thermal degradation of quaternized chitosan. Carbohydrate Polymers, 81, 182–187.10.1016/j.carbpol.2010.02.049
- Liang, Q. Y., Su, H., Yan, J., Leung, C., Cao, S. L., & Yuan, D. S. (2014). N-doped mesoporous carbon as a bifunctional material for oxygen reduction reaction and supercapacitors. Chinese Journal of Catalysis, 35, 1078–1083.10.1016/S1872-2067(14)60044-9
- Marchessault, R. H., Ravenelle, F., & Zhu, X. X. (2006). Polysaccharides for drug delivery and pharmaceutical applications (ACS symposium series). Washington, DC: American Chemical Society.10.1021/symposium
- Martina, K., Leonhardt, S. E. S. Ondruschka, B., Curini, M., Binello, A., & Cravotto, G. (2011). In situ cross-linked chitosan Cu(I) or Pd(II) complexes as a versatile, eco-friendly recyclable solid catalyst. Journal of Molecular Catalysis A: Chemical ,334, 60–64.10.1016/j.molcata.2010.10.024
- Ou, C. Y., Li, S. D., Li, C. P., Zhang, C. H., Yang, L., & Chen, C. P. (2008). Effect of cupric ion on thermal degradation of chitosan. Journal of Applied Polymer Science, 109, 957–962.10.1002/(ISSN)1097-4628
- Ou, C. Y., Yang, L., Li, C. P., Hong, P. Z., & She, X. D. (2010). The impact of cupric ion on thermo-oxidative degradation of chitosan. Polymer International, 59, 1110–1115.
- Paluszkiewicz, C., Stodolak, E., Hasik, M., & Blazewicz, M. (2011). FT-IR study of montmorillonite–chitosan nanocomposite materials. Spectrochimica Acta Part A: Molecular and Biomolecular Spectroscopy, 79, 784–788.10.1016/j.saa.2010.08.053
- Pearson, R. G. (1963). Hard and soft acids and bases. Journal of the American Chemical Society, 85, 3533–3539.10.1021/ja00905a001
- Shao, Y., Cao, C. S., Chen, S. L., He, M., Fang, J. L., Chen, J., … Li, D. Z. (2015). Investigation of nitrogen doped and carbon species decorated TiO2 with enhanced visible light photocatalytic activity by using chitosan. Applied Catalysis B: Environmental, 179, 344–351.10.1016/j.apcatb.2015.05.023
- Signini, R. (1999). On the preparation and characterization of chitosan hydrochloride. Polymer Bulletin, 42, 159–166.10.1007/s002890050448
- Sreenivasan, K. (1996). Thermal stability studies of some chitosanmetal ion complexes using differential scanning calorimetry. Polymer Degradation and Stability, 52, 85–87.10.1016/0141-3910(95)00220-0
- Taboada, E., Cabrera, G., Jimenez, R., & Cardenas, G. (2009). A kinetic study of the thermal degradation of chitosan-metal complexes. Journal of Applied Polymer Science, 114, 2043–2052.10.1002/app.v114:4
- Thangadurai, T. D., & Ihm, S. K. (2003). Chiral Schiff base ruthenium (III) complexes: Synthesis, characterisation, catalytic and antibacterial studies. Journal of Industrial and Engineering Chemistry, 9, 563–568.
- Trimukhe, K. D., & Varma, A. J. (2009). Metal complexes of crosslinked chitosans: Correlations between metal ion complexation values and thermal properties. Carbohydrate Polymers, 75, 63–70.10.1016/j.carbpol.2008.06.011
- Varma, A. J., Deshpande, S. V., & Kennedy, J. F. (2004). Metal complexation by chitosan and its derivatives: A review. Carbohydrate Polymers, 55, 77–93.10.1016/j.carbpol.2003.08.005
- Wang, Y. X., Christian, M. P., Deng, T. S., Qiao, Y., & Hou, X. L. (2013). Direct conversion of chitin biomass to 5-hydroxymethylfurfural in concentrated ZnCl2 aqueous solution. Bioresource Technology, 143, 384–390.10.1016/j.biortech.2013.06.024
- Yin, X. Q., Yuan, W., Lin, Q., Tan, Y. P., & Zhang, Q. (2006). Low molecular weight chitosan prepared from oxidative degradation of chitosan-Cu (II) complex. Chinese Journal of Applied Chemistry, 23, 729–733.
- Zamariotto, D., Lakard, B., Fievet, P., & Fatin-Rouge, N. (2010). Retention of Cu(II)- and Ni(II)-polyaminocarboxylate complexes by ultrafiltration assisted with polyamines. Desalination, 258, 87–92.10.1016/j.desal.2010.03.040