Abstract
Degenerative retinal diseases are a leading cause of visual loss and irreversible blindness, particularly in the developed world. Retinal pigment cell (RPE) transplantation is nowadays considered the most promising therapeutic approach for certain retinal diseases, and the presence of a supportive scaffold has been considered essential to ensure the success of the implant. In this work, collagen IV was covalently immobilized to the surface of polyimide membranes, with the purpose of developing scaffold materials for RPE cell culture. The covalent modification method involved four steps: argon-plasma treatment, acrylic acid graft polymerization, surface activation, and finally immobilization of collagen type IV. Collagen-modified membranes did not become more rough but became significantly more hydrophilic than the unmodified and dip-coated controls. ARPE-19 cell morphology and attachment were studied by immunofluorescence staining and confocal microscopy. Covalently modified surfaces showed cell attachment and cell properties comparable to the uncoated and dip-coated controls. This work demonstrated the potential of collagen IV-immobilized polyimide membranes as substrates for the growth of ARPE-19 cells.
Public Interest Statement
Neurodegenerative diseases of the retina are an increasingly common problem due to the worldwide aging population. Age-related macular degeneration, for instance, is one of the most prevalent diseases of the posterior eye and a leading cause of blindness in developed countries. This disease is characterized by the degeneration of a specific cell layer at the back of the eye—the retinal pigment epithelium (RPE). Currently, transplantation of cells using a supportive scaffold as cell carrier is considered the most promising means to restore the lost vision. In this work, the surface of polyimide membranes was chemically modified by immobilization of collagen, a natural component of the RPE basement membrane, having in mind the development of an appropriate environment for cell growth with stable presence of collagen. Biomaterial characterization studies and cell culture experiments demonstrated the potential of the newly developed materials as substrates for RPE cells.
1. Introduction
Visual impairment due to retinal neurodegenerative diseases is an increasingly common problem due to the worldwide aging population. Diseases such as age-related macular degeneration (AMD) not only compromise patients’ quality of life, but also cause a significant economic impact (Bull & Martin, Citation2011). For example, AMD alone is estimated to cause visual loss in more than 30 million people worldwide, with more than US$300 billion being spent annually with the disease (Ambati, Atkinson, & Gelfand, Citation2013).
Retinal pigment epithelium (RPE) is a pigmented cell monolayer that sits on the Bruch’s membrane and is located between the choriocapillaris and the photoreceptors (Bhutto & Lutty, Citation2012). RPE cells are involved in a number of important functions such as the transportation of nutrients and waste products in the retina, and performance of phagocytosis of the photoreceptor outer segments, thereby ensuring the survival and function of these light-sensitive cells (Kaarniranta et al., Citation2013). This central role of RPE cells makes them the main cause of pathogenesis in several retinal degenerative diseases, particularly when dysfunction or irreversible tissue damage occurs (Bhutto & Lutty, Citation2012).
In spite of the global impact of retinal degenerative diseases, current drug-based therapies have been shown inadequate to recover the lost vision. In contrast, cell-based therapy holds great promise and some studies have shown that RPE transplantation can indeed help to improve visual function (Binder et al., Citation2004; Bull & Martin, Citation2011; Huang, Enzmann, & Ildstad, Citation2011). Even so, the presence of a supportive surface/scaffold is needed for proper RPE cell culture and maturation in vitro and may be essential to ensure adequate cell orientation with the photoreceptors and tissue integration following transplantation (Diniz et al., Citation2013; Skottman, Citation2012; Warnke et al., Citation2013). In this context, different natural (e.g. fibroin (Shadforth, George, Kwan, Chirila, & Harkin, Citation2012)) and synthetic (e.g. poly(hydroxybutyrate-co-hydroxyvalerate) (Tezcaner, Bugra, & Hasırcı, Citation2003)) polymers have been studied as scaffolds for RPE cell culture and transplantation, but the use of synthetic polymers outmatches natural polymers in some aspects including degradation, processability, and strength. In addition, synthetic polymers can be surface-modified by proteins to improve their properties and performance such as hydrophilicity, cell adhesion, and biocompatibility (Ying et al., Citation2003).
Polyimides are synthetic polymers with high performance in medical implants. They are flexible, bio-inert, and electrically insulating, thus suitable for biosensor encapsulation or as substrates for subretinal and epiretinal prosthesis (Julien et al., Citation2011; Sachs et al., Citation2004; Van Vlierberghe et al., Citation2010; Zrenner et al., Citation2011). It has been demonstrated that dip-coated polyimide membranes with adhesive molecules such as collagen type IV and laminins can promote the maturation of human embryonic stem cell (hESC)-RPE cells, while cell attachment and growth are poor on uncoated PI membranes, or PI membranes that are dip-coated with synthetic laminin peptides, heparin sulfate (HS) and hyaluronic acid (HA) (Subrizi et al., Citation2012). Laminin and collagen type IV are both major and natural constituents of RPE basal lamina, which serves as an anchoring surface for the RPE (Subrizi et al., Citation2012). Dip-coating as surface modification method is a simple and common approach in which proteins are simply adsorbed to the surface through attractive forces such as ionic, hydrophobic, or van der Waals, and it is commonly used in cell culture procedures. However, shear forces and changes in pH of the solution can easily remove the physically absorbed protein layer. Chemical surface modification is a more stable approach for protein immobilization compared to physical modification. Using this strategy, proteins can be grafted onto the surface covalently with good stability (Cheng & Teoh, Citation2004; Custódio, Alves, Reis, & Mano, Citation2010; Xue et al., Citation2014).
In this study, we chemically modified the surface of PI membranes by covalent immobilization of collagen type IV using carbodiimides and N-hydroxysuccinimide crosslinkers, and we investigated the effect of this functionalization on the adhesion and properties of ARPE-19 cells, a commercial immortalized human RPE cell line which is commonly used to model RPE cells (Hornof, Toropainen, & Urtti, Citation2005). The results of this work demonstrate that covalently modified polyimide membranes have the potential to constitute an appropriate substrate for the growth of RPE.
2. Materials and methods
2.1. Materials
Track-etched polyimide membranes (ipCELLCULTURE™, thereafter called PI membranes) were supplied by it4ip (Seneffe, Belgium). Membranes were 24 μm thick, with pore size of 1 μm and pore density of 2.27 × 107 pores/cm2. Non-porous PI membranes of same thickness were supplied from the same company.
Aqueous acrylic acid (AAc, 99%), N-hydroxysuccinimide (NHS, 98%), N-(3-dimethylaminopropyl)-N-ethylcarbodiimide hydrochloride (EDC, crystalline), and type IV collagen from human placenta used for membrane coating were all purchased from Sigma-Aldrich (St. Louis, USA).
2.2. Surface modification
The surface modification steps of PI membranes are illustrated in Figure . In short, the method involves argon-plasma treatment, AAc graft polymerization, surface activation, and collagen immobilization. In all steps, membranes were fixed to CellCrownTM 12 inserts (Scaffdex, Tampere, Finland).
Figure 1. Schematic representation of the processes of thermally-induced AAc grafting and collagen immobilization.
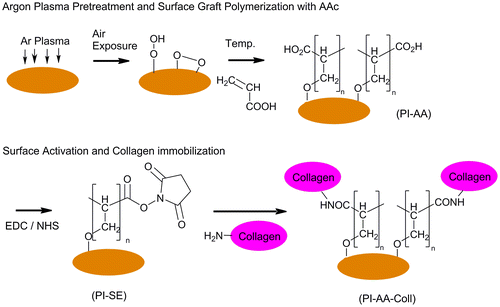
2.2.1. Argon-plasma surface treatment and AAc graft polymerization
The argon-plasma surface treatment was carried out in a Vision 320 MK II RIE system (Advance Vacuum, Lomma, Sweden). The membranes were placed inside the chamber for 60 s at an argon pressure of 250 mTorr and flow rate of 100 sccm (Bhusari, Hayden, Tanikella, Allen, & Kohl, Citation2005; Inagaki, Citation2006). The plasma power was kept at 60 W at a radio frequency of 13.6 kHz. Then, the membranes were preserved in a desiccator at atmospheric pressure and room temperature (RT) for 20 min to facilitate the formation of surface peroxides and hydroperoxides (Yu, Kang, & Neoh, Citation2002).
Membranes were further treated either with 25% or 35% (v/v) aqueous AAc solutions. The AAc graft polymerization was carried out at 60°C for 6 h under nitrogen atmosphere (Gupta, Hilborn, Bisson, & Frey, Citation2001). Thereafter, membranes were washed with excess amount of deionized water for 24 h to remove the residual monomers adsorbed on the surface. The resulting membranes, designated as PI-AA (25%) or PI-AA (35%), were dried in a desiccator before collagen immobilization.
2.2.2. Surface activation and immobilization of collagen IV on PI-AA
For surface activation, either 5 mM/5 mM or 10 mM/10 mM EDC/NHS solutions prepared in DPBS were added to PI-AA (25%) membranes (Sam et al., Citation2010). This step was carried out at RT under nitrogen (N2) atmosphere shaking manually every 15 min for 1 h, leading to succinimidyl esterification (SE) of the carboxyl groups on PI-AA (Zhang, Thompson, Markland, & Swenson, Citation2011). Membranes were rinsed 2 × 10 min with DPBS. Samples for surface characterization were dried under the stream of N2 and stored at 4°C until analyzed. The resulting films are designated as PI-SE (5) and PI-SE (10) according to EDC/NHS solution concentration used in the experiment.
PI membranes were covered with collagen IV solution (16 μg/ml) in DPBS (pH 7.4) and were incubated overnight at 4°C. After incubation, membranes were washed twice with DPBS to remove any remaining acetic acid, and subsequently with 0.1 M Na2HPO4 solution (2 h) and DPBS (2 × 10 min). Membranes, designated as PI-AA-Coll (5) and PI-AA-Coll (10), were finally dried under laminar flow and stored at 4°C until surface characterization and cell culture studies.
Dip-coated control samples were also prepared by dipping the PI membranes in collagen IV solution (16 μg/ml) in DPBS (pH 7.4) for 24 h at 4°C. Samples were rinsed twice with DPBS to remove any unbound protein and were stored at 4°C. In this study, dip-coated controls are designated as PI-Coll.
2.3. Surface characterization
2.3.1. Surface density of carboxyl groups
The number of carboxyl groups on PI-AA membranes introduced by AAc graft polymerization was determined by a colorimetric method using Toluidine Blue O (TBO) (Gupta et al., Citation2001). TBO binds to carboxyl groups in alkaline solution to form a stable electrostatic complex which can be detached from the surface in acidic solutions. In brief, the control PI, PI-AA (25%), and PI-AA (35%) samples with a reactive surface area of approximately 0.39 cm2 were incubated in 3 ml of TBO solution (0.5 mM) in 0.1 mM NaOH for 6 h at 37°C under constant agitation. Membranes were rinsed with an excess amount of fresh NaOH solution (pH~9) to remove the unbound dye. After thorough rinsing, membranes were placed in 50% (v/v) acetic acid solution for 30 min at 37°C under stirring. During this step, the TBO molecules bound to the acidic groups of PI-AA membranes were eluted from the surface and diffused into solution, coloring it blue. The light absorbance of the solution was measured at 634 nm wavelength on a Unicam UV 540 UV/VIS Spectrophotometer (Thermo Spectronic, Cambridge, England). The number of reactive carboxyl groups on the membrane was calculated from a calibration curve of TBO, with the assumption that 1 mol of TBO combines with 1 mol of carboxyl groups of the AAc-grafted polymer.
2.3.2. Water contact angle measurements
The static angle measurement of PI, PI-AA-Coll (10), PI-AA-Coll (5), and PI-Coll non-porous membranes was performed by a Theta Lite optical tensiometer (Attension, Biolin Scientific AB, Sweden) at RT. A minimum of six measurements were carried out for each sample.
2.3.3. Atomic force microscopy
The surface morphologies of unmodified PI, PI-AA-Coll (10), PI-AA-Coll (5), and PI-Coll non-porous membranes were characterized using atomic force microscope (AFM, XE-100, Park Systems Corp, USA). The AFM images were recorded by scanning in non-contact mode, under air, and at RT. The probe was supported on an APPNANOTM AFM cantilever (Applied NanoStructures Inc., USA, type: ACTA). The spring constant was 25–75 Nm−1. A total of nine randomly selected areas of 1 μm2 was analyzed in three parallel scans for each membrane. The arithmetic mean of the surface average roughness (Ra) was calculated from the roughness profile determined by AFM with XEI image processing software (Park Systems).
2.4. ARPE-19 cell culture
ARPE-19 (ATCC Manassas, VA, USA), a commercial immortalized human RPE cell line, was cultured at 37°C under 5% CO2. Cells were cultured in Dulbecco’s modified Eagle’s medium (DMEM/F12; Gibco Invitrogen, Carlsbad, CA, USA) supplemented with 10% fetal bovine serum (PAA Laboratories, Cölbe, German), 2 mM glutamax (Life Technologies, Carlsbad, CA, USA), and an antibiotic mixture of 100 U/ml penicillin/streptomycin (Cambrex Bio Science, Walkersville, MD, USA). Cells were seeded at a density of 8 × 104 cells/cm2 onto unmodified and modified PI membranes and allowed to grow to confluence. Prior to cell seeding, samples were placed under a UV lamp for 20 min to ensure sterility. Cells were cultured on unmodified and surface-modified membranes for 3 days.
2.5. Immunofluorescence staining
The ARPE-19 cells grown on the PI membranes were washed once with DPBS, fixed with 4% paraformaldehyde (PFA, pH 7.4; Sigma-Aldrich) for 10 min at RT, and washed with DPBS for 3 times. Cells were then permeabilized with 0.1% Triton X-100 in DPBS (Sigma-Aldrich) for 10 min. Thereafter, samples were rinsed several times with DPBS. Nonspecific binding sites were blocked with 3% bovine serum albumin in DPBS (BSA-DPBS) for 1 h at RT. Primary antibody incubations were done in 0.5% BSA-DPBS for 1 h at RT. Tight junctions were visualized using mouse anti-Zonula occludens-1 (ZO-1, Invitrogen, USA, 1:250). The incubation with primary antibody was followed by thorough washing with DPBS. Samples were incubated for 1 h with the secondary antibody donkey anti-mouse IgG (H + L) (Alexa Fluor® 488, Invitrogen, Molecular Probes, USA) diluted 1:800 in 0.5% BSA-DPBS. Phalloidin-tetramethylrhodamine B isothiocyanate (Sigma-Aldrich, UK) was used for labeling filamentous actin (Sigma Aldrich, 1:300). Cells were washed several times with DPBS. 4’,6’-Diamidino-2-phenylidole (DAPI) included in the mounting media was used for staining the nuclei (Vector Laboratories, Inc., Burlingame, CA). Images were either acquired with a fluorescence microscope (Olympus IX51, Olympus Corporation, Tokyo, Japan) or a Zeiss LSM 780 confocal microscope (Carl Zeiss, Jena, Germany). Eight randomly chosen areas of each sample were captured with a 10x objective. The number of cells in each image was determined using Image J Image Processing and the Cell Counter plugin. Images were processed in ZEN-software (Carl Zeiss). Cell number on investigated membranes was assessed by counting of nuclei from fluorescence microscopy images.
2.6. Statistical analyses
The statistical significance of the results was analyzed with PASW Statistics, version 18, with two-tailed Mann-Whitney U test.
3. Results and discussion
3.1. Surface modification
The surface chemistry and topology of biomaterials have an important role in the biological response and the final biocompatibility of an implant. Hence, the principle of surface modification of biomaterials is to keep their main physical properties while modifying the surface to influence cell–biomaterial interactions. In order to improve the RPE cell interaction with PI substrates, a four-step surface modification method was developed and evaluated in this study. Collagen type IV was selected as the conjugated biomolecule due to its presence in native human Bruch’s membrane and due to different studies showing the in vitro differentiation and maturation of hESC toward RPE phenotype in the presence of collagen IV (Sorkio et al., Citation2014; Subrizi et al., Citation2012).
In this study, PI membranes were firstly pre-treated by Ar plasma and exposed to air to create peroxides on the surface, as shown in Figure . Graft polymerization of aqueous acrylic acid (AAc) on PI was carried out under the effect of polymerization initiator (peroxides) and thermal energy. Thermal-induced acrylic acid grafting on plasma-pre-treated PI membrane was carried out with two monomer concentrations of 25 and 35%. The surface density of carboxyl groups determined by the TBO method was 12.1 ± 0.3 μg/cm2 for PI-AA (25%) and 7.8 ± 0.6 μg/cm2 for PI-AA (35%) membranes, and 6.0 μg/cm2 for the unmodified PI membrane. Previous studies have shown that an increase in AAc monomer concentration used for graft copolymerization generally causes an increase in the concentration of the surface-grafted carboxyl groups (Ying et al., Citation2003). However, it has also been observed that once a maximum peak in surface concentration of carboxyl groups is achieved, a subsequent sharp decrease follows at higher monomer concentrations, which has been attributed to extensive homopolymerization in the system (Gupta, Srivastava, Grover, & Saxena, Citation2010). In our study, it is supposed that 25% AAc monomer concentration is located in the rising region of the curve and 35% AAc monomer concentration is located in the decreasing part of the curve, where the carboxyl concentration on the surface tends to decrease by increasing monomer concentrations. In this study, PI-AA (25%) membranes were selected for further surface activation and collagen immobilization, since it was expected that the higher surface density of carboxyl groups could lead to a higher concentration of immobilized collagen (Cheng & Teoh, Citation2004; Gupta, Plummer, Bisson, Frey, & Hilborn, Citation2002).
In the subsequent step, EDC/NHS reactivated the carboxyl groups on the surface of PI membrane by formation of amine-reactive NHS-esters. Different studies report various concentrations of NHS and EDC from a few M down to the mM range, but the optimal concentration for the activation of carboxylic acids has previously been found in the range of 5 mM < [EDC] ~ [NHS] < 10 mM (Sam et al., Citation2010). In the current study, both 5 mM/5 mM and 10 mM/10 mM EDC/NHS concentrations were hence tested for surface activation in order to determine the conditions leading to optimal collagen immobilization, cell adhesion, and cell properties, as discussed in the following sections. Surface modification was further confirmed by Attenuated Total Reflectance-Fourier Transform Infrared Spectroscopy (ATR-FTIR), and the spectra are provided as Supplementary material. In spite of the predominance of peaks related with the presence of PI, sharper peaks in the region 3,000–2,800 cm−1 were found for the AAc grafted membranes, associated with H-bonding of carboxylic acids. The new peaks found in the region of 1,440–1,400 cm−1 are further related to the presence of O-H bend in the carboxyl group. In addition, a qualitative measurement of the bound collagen obtained using indirect immunofluorescence staining and image analysis (Sorkio et al., Citation2014) (Supplementary material) suggested that the modified membranes PI-AA-Coll had a higher presence of collagen than the unmodified or dip-coated counterparts.
3.2. Surface wettability
Water contact angles were measured in order to evaluate the hydrophilicity of the modified membranes (Figure ). Unmodified PI surface was found to be rather hydrophobic with average measured contact angle of 76.6 ± 4.4°. The dip-coated control (PI-Coll) showed a significant decrease (p < 0.001) in contact angle to 52.8 ± 4.9°, in agreement with the physical adsorption of the protein to the surface. AAc graft polymerization (PI-AA) also caused a significant increase (p < 0.001) in the hydrophilicity of PI membranes (58.1 ± 6.4°), which can be explained by the presence of carboxyl groups on the material surface capable of forming additional hydrogen bonds with water molecules.
Figure 2. Static contact angle of unmodified and modified PI membranes.
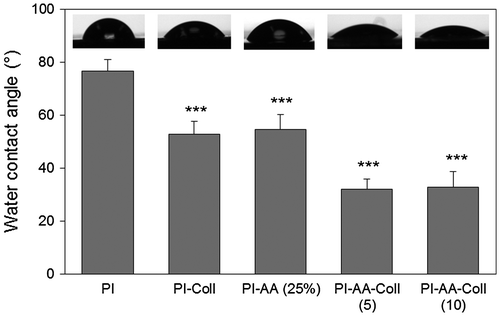
Samples that were covalently modified with collagen (PI-AA-Coll (5) and PI-AA-Coll (10)) showed significantly lower contact angle (31.1 ± 3.6° and 32.7 ± 5.9°, respectively) than the unmodified, PI-AA and dip-coated PI membranes (p ≤ 0.001). When PI-AA-Coll (5) and PI-AA-Coll (10) were compared to the AAc graft polymerized (PI-AA) sample, differences were also highly significant (p < 0.001). The increased hydrophilicity of these membranes could be due to a combination of factors such as the high hydrophilicity of collagen IV, improved surface coverage and high surface exposure of hydrophilic groups following immobilization. However, differences between PI-AA-Coll (5) and PI-AA-Coll (10) were not found statistically significant, suggesting that the membranes were similarly modified despite the different concentration of crosslinker employed in the modification.
3.3. Surface morphology
Surface roughness can have a significant effect over cell-material interactions (Tezcaner et al., Citation2003). For this reason, the effect of surface modification on the surface roughness of PI membranes was evaluated by AFM. In all cases, surfaces were found to be rather smooth, and the functionalization steps did not introduce significant changes to the topography of the membranes (Figure ).
Figure 3. Representative AFM images of the (A) Unmodified PI membrane, (B) PI-AA (25%) and (C) PI-AA-Coll (5).
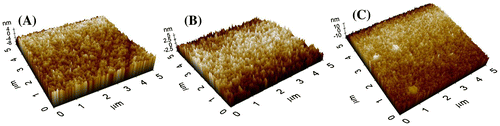
The arithmetic mean of the surface roughness (Ra) of the control PI was 0.87 ± 0.12 nm (Figure ). The surface roughness slightly increased with collagen adsorption in PI-Coll surfaces, although the differences were not found significant. The surface roughness of PI-AA (25%) was similar to control PI, with a Ra of 0.82 ± 0.10 nm. However, covalently modified surfaces PI-AA-Coll (5) and PI-AA-Coll (10) became significantly rougher than the unmodified membrane PI (p = 0.006 and p = 0.003, respectively) and PI-AA (p < 0.001), with average surface roughness of 1.08 ± 0.08 for PI-AA-Coll (5) and 1.13 ± 0.13 nm for PI-AA-Coll (10).
Figure 4. Arithmetic mean of the surface roughness of control and modified PI membranes determined from AFM images (n = 9).
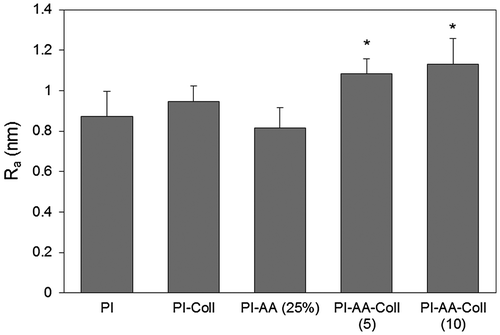
Differences between PI-AA-Coll (5) and PI-AA-Coll (10) were not found statistically significant, but the former was significantly rougher than the dip-coated control PI-Coll (p = 0.001). Considering the etching effect of plasma treatment, the surface roughness was expected to increase and a hill-valley structure to be formed on the surface, with the grafted acrylic acid chains on the surface of PI membranes filling up the valleys and leading to a smoother surface (Gupta et al., Citation2002). In addition, the small increase in the surface roughness of covalently modified membranes probably arises from the activation of grafted carboxyl groups on the surface and collagen immobilization. In any case, considering the absolute values of surface roughness, these results demonstrate that the surface modification method does not cause a noticeable change.
3.4. Immunofluorescence staining and cell morphology
The maturation of ARPE-19 cells on different PI surfaces was assessed after 3 days of culture (Figure ). ARPE-19 cells displayed hexagonal cell morphology both on covalently modified membranes and control membranes, and expressed the tight junction protein ZO-1, a marker for RPE cell culture polarization (Burke, Citation2008). Phalloidin staining of filamentous actin additionally confirmed the typical hexagonal RPE cell morphology on all investigated samples. ARPE-19 cells both on PI-Coll and chemically modified PI membranes showed normal morphology, suggesting that the surface modification of the PI membrane does not cause any observable detrimental effects on ARPE-19 cells and that the collagen-functionalized surface can provide an appropriate substrate for the formation of RPE monolayers.
Figure 5. Immunocytochemistry of ARPE-19 cells on PI membranes after 3 days of culture.
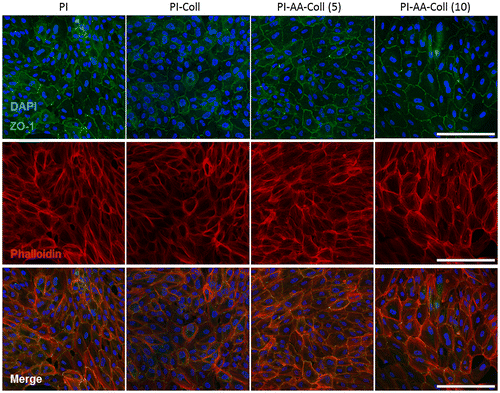
Cell number assessed from fluorescence microscopy images is shown in Figure . After 3 days, cells had formed a confluent monolayer on the covalently modified membranes, although cell number was lower (p ≤ 0.001) than that observed for the control PI and PI-Coll surfaces (Figure (A)). The large cell size and standard deviation for all samples (Figure (B)) are in consistency with the short culture period, where adhesion and cell-cell interactions dominate, as shown by the significant presence of actin stress fibers (Figure ).
Figure 6. (A) Cell number of ARPE-19 cells cultured on control and modified PI membranes for 3 days and (B) Average cell sizes (n = 40).
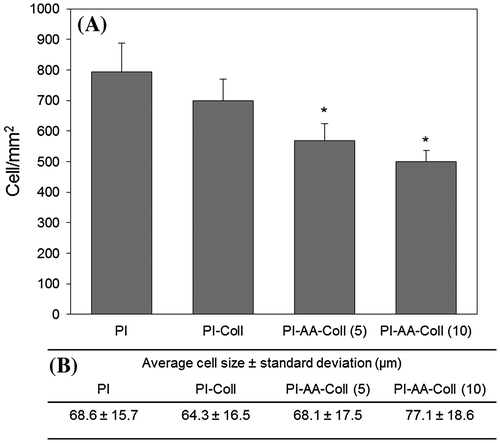
Differences in cell number between PI-AA-Coll (5) and PI-AA-Coll (10) were not found significant, which is well in agreement with the similarities found for their surface properties, such hydrophilicity and roughness. This emphasizes that the crosslinker concentration differences used in this study did not cause significant variation in terms of the activation of carboxylic acids, collagen immobilization, and subsequent cell properties.
4. Conclusion
In the present work, PI membranes were chemically modified using a controlled surface modification method for collagen IV immobilization, having in mind the production of scaffold materials for RPE transplantation. Covalent immobilization of collagen type IV caused small changes in roughness of the PI membranes and they became more hydrophilic than the unmodified and dip-coated controls. Here, we showed that ARPE-19 cells adhered both to the modified and control materials and formed a continuous epithelial cell layer demonstrating typical RPE morphology and expression of the RPE cell marker ZO-1. Thus, the covalently modified PI-membranes can constitute appropriate substrates for the growth of RPE-type cells, such as ARPE-19.
Funding
This work was supported by the Health Research Council of the Academy of Finland (HS, KJU [grant number 218050], [grant number 137801]), by the joint program of Academy of Finland and Japan Science and Technology Agency of the Academy of Finland (Active Biomaterials for Retinal Implants [grant number 253134]) and by the program of Human Spare Parts of TEKES—The Finnish Funding Agency for Innovation.
Supplementary material
Supplementary material for this article can be accessed here http://dx.doi.org/10.1080/23312009.2017.1292593.
Supplementary_material.docx
Download MS Word (695.9 KB)Acknowledgments
The funders had no role in study design, data collection and analysis, decision to publish, or preparation of the manuscript. We thank PhD Niina Ahola, PhD Elli Käpylä and BSc Elina Pajula for the technical assistance.
Additional information
Notes on contributors
Shokoufeh Teymouri
BioMediTech is a joint research institute between Tampere University of Technology and the University of Tampere that conducts world-class research in the fields of biomedicine and medical technology. The diversity of multidisciplinary research groups in BioMediTech has as common aim, the development of personalized medicine. The Biomaterials and Tissue Engineering group has a long tradition of developing biodegradable implants made from medical polymers and composites. The Eye group has been focusing on developing novel stem cell-based tools for corneal and retinal repair through cell transplantation and ophthalmic in vitro tissue models. For this project in particular, the Biomaterials and Tissue Engineering group and the Eye group have been working together to create biodegradable scaffolds for the regeneration of the retina.
References
- Ambati, J., Atkinson, J. P., & Gelfand, B. D. (2013). Immunology of age-related macular degeneration. Nature Reviews Immunology, 13, 438–451. doi:10.1038/nri3459
- Bhusari, D., Hayden, H., Tanikella, R., Allen, S. A. B., & Kohl, P. A. (2005). Plasma treatment and surface analysis of polyimide films for electroless copper buildup process. Journal of The Electrochemical Society, 152, F162–F170. doi:10.1149/1.2006587
- Bhutto, I., & Lutty, G. (2012). Understanding age-related macular degeneration (AMD): Relationships between the photoreceptor/retinal pigment epithelium/Bruch’s membrane/choriocapillaris complex. Molecular Aspects of Medicine, 33, 295–317. doi:10.1016/j.mam.2012.04.005
- Binder, S., Krebs, I., Hilgers, R.-D., Abri, A., Stolba, U., Assadoulina, A., ... Feichtinger, H. (2004). Outcome of transplantation of autologous retinal pigment epithelium in age-related macular degeneration: A prospective trial. Investigative Opthalmology & Visual Science, 45, 4151–4160. doi:10.1167/iovs.04-0118
- Bull, N. D., & Martin, K. R. (2011). Concise review: Toward stem cell-based therapies for retinal neurodegenerative diseases. Stem Cells, 29, 1170–1175. doi:10.1002/stem.676
- Burke, J. M. (2008). Epithelial phenotype and the RPE: Is the answer blowing in the Wnt? Progress in Retinal and Eye Research, 27, 579–595. doi:10.1016/j.preteyeres.2008.08.002
- Cheng, Z., & Teoh, S.-H. (2004). Surface modification of ultra thin poly (ε-caprolactone) films using acrylic acid and collagen. Biomaterials, 25, 1991–2001. doi:10.1016/j.biomaterials.2003.08.038
- Custódio, C. A., Alves, C. M., Reis, R. L., & Mano, J. F. (2010). Immobilization of fibronectin in chitosan substrates improves cell adhesion and proliferation. Journal of Tissue Engineering and Regenerative Medicine, 4, 316–323. doi:10.1002/term.248
- Diniz, B., Thomas, P., Thomas, B., Ribeiro, R., Hu, Y., Brant, R., ... Humayun, M. S. (2013). Subretinal implantation of retinal pigment epithelial cells derived from human embryonic stem cells: Improved survival when implanted as a monolayer. Investigative Opthalmology & Visual Science, 54, 5087–5096. doi:10.1167/iovs.12-11239
- Gupta, B., Hilborn, J. G., Bisson, I., & Frey, P. (2001). Plasma-induced graft polymerization of acrylic acid onto poly(ethylene terephthalate) films. Journal of Applied Polymer Science, 81, 2993–3001. doi:10.1002/app.1749
- Gupta, B., Plummer, C., Bisson, I., Frey, P., & Hilborn, J. (2002). Plasma-induced graft polymerization of acrylic acid onto poly(ethylene terephthalate) films: Characterization and human smooth muscle cell growth on grafted films. Biomaterials, 23, 863–871. doi:10.1016/S0142-9612(01)00195-8
- Gupta, B., Srivastava, A., Grover, N., & Saxena, S. (2010). Plasma induced graft polymerization of acrylic acid onto poly(ethylene terephthalate) monofilament. Indian Journal of Fibre & Textile Research, 35, 9–14.
- Hornof, M., Toropainen, E., & Urtti, A. (2005). Cell culture models of the ocular barriers. European Journal of Pharmaceutics and Biopharmaceutics, 60, 207–225. doi:10.1016/j.ejpb.2005.01.009
- Huang, Y., Enzmann, V., & Ildstad, S. T. (2011). Stem cell-based therapeutic applications in retinal degenerative diseases. Stem Cell Reviews and Reports, 7, 434–445. doi:10.1007/s12015-010-9192-8
- Inagaki, N. (2006). Polymer films produced by plasma polymerization. In Y. Pauleau (Ed.), Materials surface processing by directed energy techniques (Vol. 20, pp. 659–707). Oxford: Elsevier.
- Julien, S., Peters, T., Ziemssen, F., Arango-Gonzalez, B., Beck, S., Thielecke, H., ... Schraermeyer, U. (2011). Implantation of ultrathin, biofunctionalized polyimide membranes into the subretinal space of rats. Biomaterials, 32, 3890–3898. doi:10.1016/j.biomaterials.2011.02.016
- Kaarniranta, K., Sinha, D., Blasiak, J., Kauppinen, A., Veréb, Z., Salminen, A., ... Petrovski, G. (2013). Autophagy and heterophagy dysregulation leads to retinal pigment epithelium dysfunction and development of age-related macular degeneration. Autophagy, 9, 973–984.10.4161/auto.24546
- Sachs, H. G., Schanze, T., Wilms, M., Rentzos, A., Brunner, U., Gekeler, F., & Hesse, L. (2004). Subretinal implantation and testing of polyimide film electrodes in cats. Graefe’s Archive for Clinical and Experimental Ophthalmology, 243, 464–468. doi:10.1007/s00417-004-1049-x
- Sam, S., Touahir, L., Salvador Andresa, J., Allongue, P., Chazalviel, J.-N., Gouget-Laemmel, A. C., ... Djebbar, S. (2010). Semiquantitative study of the EDC/NHS activation of acid terminal groups at modified porous silicon surfaces. Langmuir, 26, 809–814. doi:10.1021/la902220a
- Shadforth, A. M. A., George, K. A., Kwan, A. S., Chirila, T. V., & Harkin, D. G. (2012). The cultivation of human retinal pigment epithelial cells on Bombyx mori silk fibroin. Biomaterials, 33, 4110–4117. doi:10.1016/j.biomaterials.2012.02.040
- Skottman, H. (2012). Differentiation of human embryonic stem cells and human induced pluripotent stem cells into retinal pigment epithelium. In M. A. Hayat (Ed.), Stem cells and cancer stem cells (Vol. 7, pp. 187–194). Netherlands: Springer.
- Sorkio, A., Hongisto, H., Kaarniranta, K., Uusitalo, H., Juuti-Uusitalo, K., & Skottman, H. (2014). Structure and barrier properties of human embryonic stem cell-derived retinal pigment epithelial cells are affected by extracellular matrix protein coating. Tissue Engineering. Part A, 20, 622–634. doi:10.1089/ten.TEA.2013.0049
- Subrizi, A., Hiidenmaa, H., Ilmarinen, T., Nymark, S., Dubruel, P., Uusitalo, H., ... Skottman, H. (2012). Generation of hESC-derived retinal pigment epithelium on biopolymer coated polyimide membranes. Biomaterials, 33, 8047–8054. doi:10.1016/j.biomaterials.2012.07.033
- Tezcaner, A., Bugra, K., & Hasırcı, V. (2003). Retinal pigment epithelium cell culture on surface modified poly(hydroxybutyrate-co-hydroxyvalerate) thin films. Biomaterials, 24, 4573–4583. doi:10.1016/S0142-9612(03)00302-8
- Van Vlierberghe, S., Sirova, M., Rossmann, P., Thielecke, H., Boterberg, V., Rihova, B., ... Dubruel, P. (2010). Surface modification of polyimide sheets for regenerative medicine applications. Biomacromolecules, 11, 2731–2739. doi:10.1021/bm100783 h
- Warnke, P. H., Alamein, M., Skabo, S., Stephens, S., Bourke, R., Heiner, P., & Liu, Q. (2013). Primordium of an artificial Bruch’s membrane made of nanofibers for engineering of retinal pigment epithelium cell monolayers. Acta Biomaterialia, 9, 9414–9422. doi:10.1016/j.actbio.2013.07.029
- Xue, P., Bao, J., Chuah, Y. J., Menon, N. V., Zhang, Y., & Kang, Y. (2014). Protein covalently conjugated su-8 surface for the enhancement of mesenchymal stem cell adhesion and proliferation. Langmuir, 30, 3110–3117. doi:10.1021/la500048z
- Ying, L., Yin, C., Zhuo, R. X., Leong, K. W., Mao, H. Q., Kang, E. T., & Neoh, K. G. (2003). Immobilization of galactose ligands on acrylic acid graft-copolymerized poly(ethylene terephthalate) film and its application to hepatocyte culture. Biomacromolecules, 4, 157–165. doi:10.1021/bm025676w
- Yu, Z. J., Kang, E. T., & Neoh, K. G. (2002). Electroless plating of copper on polyimide films modified by surface grafting of tertiary and quaternary amines polymers. Polymer, 43, 4137–4146. doi:10.1016/S0032-3861(02)00263-X
- Zhang, C., Thompson, M. E., Markland, F. S., & Swenson, S. (2011). Chemical surface modification of parylene C for enhanced protein immobilization and cell proliferation. Acta Biomaterialia, 7, 3746–3756. doi:10.1016/j.actbio.2011.06.003
- Zrenner, E., Bartz-Schmidt, K. U., Benav, H., Besch, D., Bruckmann, A., Gabel, V.-P., ... Wilke, R. (2011). Subretinal electronic chips allow blind patients to read letters and combine them to words. Proceedings of the Royal Society B: Biological Sciences, 278, 1489–1497. doi:10.1098/rspb.2010.1747