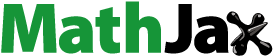
Abstract
In this paper, a study of the adsorption of carbon dioxide onto raw Chorfa silt material (Mascara, Algeria) was experimentally investigated using volumetric technique at 295 K and at a pressure range from 15.59 to 750 mm Hg. XRD, MEB, and BET analysis were used to characterize the adsorbent. This material consisted of calcite and illite as main components. Several models were used to describe the experimental isotherm such as Langmuir, Freundlich, Hill De Boer, and Sips. The best fit of adsorption isotherm data is obtained with the Sips model. The proposed methodology for the analysis of the experimental adsorption data allows the determination of the micropore volume (V0) and the characteristic energy (E) using Dubinin–Raduskevich equation. An experimental value of V0 equal to 0.875 cm3/g is obtained in very good agreement with those found using the “t-plot” method, it is equal to 0.920 cm3/g. The characteristic energy E is equal to 20.9 kJ/mol, this value indicates that the adsorption is of physical type. The unipore diffusion model was selected for sorption kinetic modeling. The diffusion process of CO2 in the silt is slow. A thermodynamic study showed spontaneous nature and feasibility of the adsorption process.
Public Interest Statement
CO2 is considered to be one of the gases that might enhance the anthropogenic greenhouse effect. Currently, one available option is to reduce CO2 emissions into the atmosphere. Adsorption has been considered to be an environment-friendly and cost-competitive process for the carbon dioxide capture.
This work aims to remove this pollutant by dam silt. This material is the sediment of the dam, which contains generally calcium carbonates and a mixture of clays. We study the mechanism of sorption and diffusion of carbon dioxide onto silt.
The novelty of this work is that it contributes in mitigating climate change. The feedstock obtained from silt, the low regeneration cost of the adsorbent and its long-term stability add more attraction for its potential use for CO2 capture.
1. Introduction
Increasing atmospheric CO2 concentrations, mainly caused by fossil fuel combustion, have become an important concern for global warming because these emissions increased significantly in the last century and still rise continuously at a faster rate. The most important sources of CO2 emissions are the fossil-fuel fired plants.
Carbon capture and storage (CCS) strategy has the potential to reduce the overall mitigation costs and to increase the flexibility in achieving a reduction in greenhouse gas (GHG) emissions (Figueroa, Fout, Plasynski, McIlvried, & Srivastava, Citation2008; Li & Fan, Citation2008; Metz, Davidson, Coninck, Loos, & Meyer, Citation2005).
International Panel on Climate Change (IPCC) predicts that, by the year 2100, the atmosphere may contain up to 570 ppmv CO2, causing a rise of mean global temperature of around 1.9°C and an increase in mean sea level of 38 cm (Yang et al., Citation2008). Currently, one available option is to reduce CO2 emissions into the atmosphere.
Thus, both geological or oceanic sequestration, and utilization of carbon dioxide for friendly environmental physical or chemical processes require the development of techniques for the cost-effective separation and capture of CO2. Therefore, the development of more efficient and less-energy intensive processes for selective separation of CO2 is one of the important research topics in the field of environmental engineering (Song, Citation2006). However, different technologies have been used to capture and separate CO2 from a mixture of gases, such as absorption (Wang, Joel, Ramshaw, Eimer, & Musa, Citation2015), physical and chemical adsorption (Montagnaro et al., Citation2015), cryogenic processes (Xu et al., Citation2012), and membrane separation (Yan et al., Citation2008).
From all the capture processes, the most studied is absorption in alkanol-amines such us monoethanol-amine MEA, diethanolamine (DEA), and methyl-diethanolamine (MDEA) are often used as absorbents (Tamajón, Álvarez, Cerdeira, & Gómez-Díaz, Citation2016). Nonetheless, there are several drawbacks using alkanolamines such as high corrosion at installation, oxidation–reduction of the absorbent in the presence of air, and energy penalty to regenerate alakanolamine. Alkanolamine solvent regeneration needs a large amount of energy due to the strong linkage between CO2 molecules and amine absorbents (Sayari, Belmabkhout, & Serna-Guerrero, Citation2011). In this context, adsorption onto porous solids is an effective technique for the removal of different type of pollutants from gaseous or liquid streams, thanks to the potentially high selectivity toward the target contaminant and its general low maintenance costs (Balsamo et al., Citation2011). For CCS application, different adsorbents, such as carbonaceous materials, zeolites, mesoporous silicas, metal organic frameworks, clays, etc., are currently being investigated (Hefti, Marx, Joss, & Mazzotti, Citation2015; Hong, Perera, & Burrows, Citation2015; Keramati & Ghoreyshi, Citation2014; Pires, Bestilleiro, Pinto, & Gil, Citation2008; Samanta, Zhao, Shimizu, Sarkar, & Gupta, Citation2012). So, in the adsorption technology, regeneration of adsorbents needs the minimum energy. The adsorbents can be used in multiple cycles without any loss.
In recent years, many porous mineral materials found increasing interest as adsorbents due to their abundance in nature, low cost, good cation adsorptive properties, and large surface area (Bhattacharyya & Gupta, Citation2008). Mineral materials used to capture carbon dioxide include smectite (Pires et al., Citation2008), montmorillonite (Azzouz et al., Citation2010), dolomite (Gallucci, Stendardo, & Foscolo, Citation2008), and carbonate (Blamey, Al-Jeboori, Manovic, Fennell, & Anthony, Citation2016), these materials were applied successfully.
Among the microporous materials most abundant in nature, one finds the silt, which can be used as adsorbent for CO2 capture. This material is the sediment of the dam, which contains generally calcium carbonates and a mixture of clays (Ouadjenia-Marouf, Marouf, Schott, & Yahiaoui, Citation2013). In the present work we examined the possibility of application the silt as adsorbent to capture the carbon dioxide at low pressure and ambient temperature. This material was obtained from Chorfa dam (Mascara, western Algeria).
However to characterize the material, we used the XRD, BET, and chemical composition technique. Several models have also been discussed to describe the experimental isotherms, which are Langmuir, Freundlich, Hill De Boer, and Sips. The porous texture of the solid is characterized by the adsorption of the carbon dioxide gas at low pressure since its saturation pressure at 295 K. The micropore volume is determined, different computation approaches are proposed depending on the type of pores present in the solid. The method for determination of the micropore volume was proposed by Dubinin–Radushkevich (DR), based on the Polanyi’s potential theory of adsorption (Dubinin & Radushkevich, Citation1947; Polanyi, Citation1914). Alternatively, the method, known as the “t-plot”, developed by Deboer, Linsen, and Osinga (Citation1965) allows the determination of the microporous volume and the external surface of the solids as well. Finally, the results obtained with the two methods above-mentioned were compared.
For more explaining the phenomenon of diffusion of carbon dioxide in the silt, we carried out a kinetic study. The conventional approach for modeling matrix gas transport is the application of the unipore diffusion model. This approach gives a first approximation of diffusion parameters. The Gibbs energy will be also determined in this study.
2. Material and methods
2.1. Materials and characterization
The silt used in this investigation was obtained from Chorfa dam in Sig region (Mascara, Algeria), in the form of fine powder of yellowish brown color. The chemical composition of the raw silt was determined by a Cameca SX-50 electronic microprobe. Before using, the material was washed with deionized water Milli-Q, dried at 95°C for 24 h, sieved and ground to 75 μm. X-ray analyze was performed using INEL CPS 120 diffractometer employing cobalt Kα radiation (λ = 0.178 nm). The specific surface area was measured by adsorption of nitrogen at 77 K (BET method) via a Quantachrome instrument. The crystallite morphology of the samples was performed by scanning electronic microscopy (JEOL, JSM-6360, Japan).
2.2. CO2 adsorption measurement
Sorption isotherm of carbon dioxide on the silt was measured volumetrically using the Micromeritics ASAP 2020 device. A detailed description of this device was given in a previous work (Khelifa, Derriche, & Bengueddach, Citation1999). The experiment was performed with a sample mass of the raw silt 0.4497 g, at constant temperature 22°C ± 0.1°C and the pressure range from 15.59 to 750 mm Hg. The carbon dioxide gas used as adsorbate is provided from air liquid. For the collection of adsorption data, the doses of adsorbate were sequentially introduced to atmospheric pressure. After each addition, the pressure and the mass balance are identified in order to trace the isotherm representing the amounts of adsorbed DOC according to the relative pressure P/P0 at equilibrium. The time for the establishment of adsorption equilibrium was 2 h 26 min.
3. Results and discussion
3.1. Textural properties of the silt
The chemical composition of natural silt is given in the Table . This result indicates the presence of silica, lime, and alumina as major constituents along with traces of iron, magnesium, potassium, titanium, sodium, and manganese oxides in the form of impurities. The XRD pattern of natural silt was recorded on Figure and the most intense peaks were observed at d = 3.34 and 3.03 Å which attributed to illite and calcite, respectively. We note also the existence of kaolinite, quartz, montmorillonite, and dolomite as accessory minerals. SEM images of raw silt mineral are shown in Figure . It is evident from the obtained SEM images that the crystallites observed have the same morphological form as that of illite crystallites. The specific surface area calculated by the BET equation was estimated as 65.57 m2/g. We notice that this value is more significant compared with surfaces area of other materials such as montmorillonite (Bhattacharyya & Gupta, Citation2008), kaolinite (Sari, Tuzen, Citak, & Soylak, Citation2007), and china clay (Sharma, Citation2008).
Table 1. Chemical composition of raw silt
3.2. Adsorption isotherm
The isotherm for carbon dioxide adsorption on silt is presented in Figure . According to the IUPAC recommendations (IUPAC Commission on Colloid & Surface Chemistry, Citation1994), the first step is to identify the isotherm type and hence the nature of the adsorption process. The experimental isotherm obtained is of type I from this classification, characteristic of a microporous material. The isotherm shows a gradual increase of CO2 adsorbed amount with increasing pressure. Furthermore, it is noticed that there are no plateaus in the adsorption isotherm within the investigated pressure range, but a linear shape in beginning of isotherm. The uptake of CO2 on silt was 2.68 cm3/g (0.12 mmol/g) at 295 K and atmospheric pressure. This value was compared with that found in other works (Table ).
Table 2. Comparison between the CO2 uptake values found in the literature and those obtained in this work
From this table, it can be said that the realization of an adsorption isotherm in order to deduce the maximum adsorbed amount depends on several parameters, namely the structural characteristics of the adsorbent and the operating conditions such as temperature and pressure.
3.3. Models fitting
Several sorption isotherm models have been proposed to represent the adsorption of gases on solids. Some of these, the Langmuir, Freundlich, Hill de Boer, and Sips models are explained in more detail below.
The fit of the experimental curves with these models makes it possible to obtain information concerning the capacity, the force of adsorption and the energy heterogeneity of the sample during the adsorption of CO2.
The accuracy of the fit of a model with the experimental data is given by the regression coefficient and the average relative error. The model that best describes our experimental data that which has a regression coefficient close to unity and the average relative error higher than 10%. The average relative error, Erm, is determined by the relation:(1)
(1)
where Vexp is the experimental amount of adsorbate, Vcal that calculated with the model, and Nexp the number of experimental data.
3.3.1. Langmuir equation
The most common theoretical model for monolayer adsorption is the Langmuir model (Langmuir, Citation1918). The model is based on various assumptions, such as the adsorbent surface is energetically homogeneous with respect to adsorption of one gas (Ruthven, Citation1984). In addition, a dynamic equilibrium is assumed between adsorbed and non-adsorbed molecules as well as formation of a single monolayer of molecules at constant temperature T and pressure P. The Langmuir equation can be written in the following form:(2)
(2)
where V is the volume of adsorbate, Vm that adsorbed at saturation, θ is the surface coverage, and K a coefficient depending on the temperature. Linear plots are obtained in coordinates P/V = f (P), if this equation is verified. The parameters of linearization are summarized in Table . The Langmuir equation provides a satisfactory representation of adsorption isotherm of carbon dioxide by the silt. The error does not exceed 10% and that the coefficient of determination is equal to 0.97 but the amount adsorbed at saturation is greater than that found experimentally. Poor correlation with the Langmuir equation was observed for CO2 adsorption, except for particular case, some studies found a close agreement between the experimental and Langmuir theoretical isotherms for the systems, CO2/activated carbon (Gao et al., Citation2013), CO2/coal (Dutta, Harpalani, & Prusty, Citation2008), and CO2/NaETS-4 (Pillai, Peter, & Jasra, Citation2008).
Table 3. Parameters of linearization of the Langmuir, Freundlich, Hill de Boer, and Sips models
3.3.2. Freundlich equation
The Freundlich isotherm model is an exponential equation that applies to adsorption on heterogeneous surfaces with interaction between adsorbed molecules. The expression of the Freundlich model is given as (Freundlich, Citation1926):(3)
(3)
where KF and n are Freundlich model constants. According to Equation (3), the plot of ln (V) against ln (P) should be linear (Figure ). The slope ranges between 0 and 1 is a measure of adsorption intensity or surface heterogeneity, becoming more heterogeneous as its value gets closer to zero. The estimated values of the parameters of the best fitting isotherm model are presented in Table .
The Freundlich model was found to give the best fit for the adsorption of carbon dioxide onto silt. The value of regression coefficient is close to unit, proving good fitting of the experimental results by this model. The value of 1/n less than 1 reflected that carbon dioxide molecules are favorably adsorbed on the silt adsorbent. The representativeness of this model could be explained by the fact that the surface of solid is heterogeneous, therefore it is a multilayer adsorption with interactions between the adsorbed molecules. In previous work, the model of Freundlich describes well the experimental data of carbon dioxide sorption by the activated carbon (Montagnaro et al., Citation2015), natural zeolite (Siriwardane, Shen, & Fisher, Citation2003), and silica mesoporous materials (Chalal, Bouhali, Hamaizi, Lebeau, & Bengueddach, Citation2015).
3.3.4. Hill–De Boer equation
Hill and De Boer gave an equation for the isotherm, which takes account of interactions and the mobility of the adsorbed phase (De Boer, Citation1953; Hill, Citation1946). The Hill–De Boer multilayer local isotherm equation was adopted since it allowed for both lateral adsorbate interactions and multilayer interactions (House & Jaycock, Citation1974).
(4)
(4)
where K1 and K2 represent adsorbate–adsorbent and the adsorbate–adsorbate interactions, respectively. A number of investigators have reported an appreciable range of fit of Equation (4), although generally this is not found above θ = 0.5 (Sing, Citation1973).
Equation (4) may be rearranged to give the linear form:(5)
(5)
The test for the applicability of this equation to the experimental data is a linear plot of y = f (θ). The parameters of linearization of this model are given in Table . It should be noted that the k2 constant is positive, which would indicate interaction between the adsorbed molecules.
The coefficient of determination R2 is very low (0.769) and the average relative error is higher than 10% which means that the model of Hill–De Boer did not represent our experimental data.
The very poor description of this model with respect to experimental data would lie in the assumptions of this equation: both the mobility of the adsorbed phase and adsorbate–adsorbent interactions seem to be unlikely in CO2 adsorption on silt, at least at low coverage.
3.3.5. Sips equation
The Sips model is an improvement of the Freundlich and Langmuir equations. The simplest isotherm equation describing localized adsorbed molecules on sites which are independent of each other (Sips, Citation1948), expressed as:(6)
(6)
The linearized form of this equation is given by:(7)
(7)
The constant A could be regarded as being representative of the strength of adsorption. A higher value of A implies a stronger adsorbate–adsorbent interaction, at least at low coverage. When the constant n is equal to 1, the Sips equation is reduced to that of Langmuir; therefore the deviation of 1/n from unity may be taken as a measure of the deviation from the Langmuir isotherm.
If the Sips equation is checked, straight lines must be obtained in coordinates log(θ/(1 − θ)) = f (log P). The parameters of linearization are summarized in Table . The determination of parameters A and n by linear regression makes possible the calculation of theoretical isotherms and their comparison with the experimental data (Figure ). As seen from Table , the correlation coefficient of the Sips model is close to unit and average relative error is smallest compared to found in the other equations. The Sips equation provides a very satisfactory description of CO2 adsorption on silt. As showed by the constant A, the silt solid has weak interaction with adsorbate. Factor n is higher than unity which implies that the silt has a heterogeneous surface. The value of the theoretical adsorbed capacity is equal to 2.81 cm3/g, it is close to that found in experiment (2.68 cm3/g). Good agreement between the experimental isotherms and the model of Sips was also found, in the case of zeolithe (Behvandi & Taurani, Citation2011; Belin, Mve Mfoumou, Mignard, & Pouilloux, Citation2013)
3.4. Micropore volume
The exploitation of isotherm of carbon dioxide adsorption makes it possible to evaluate the porous texture of the adsorbent. Contrary to nitrogen, the carbon dioxide, characterized by a strong saturating vapor pressure and a weak surface coverage, allows the quantification of microporosity. The microporous volume of adsorbent is estimated by two different methods by way of comparison, the Dubinin–Raduskevich (DR) equation and t-plot method.
3.4.1. Dubinin–Radushkevitch
The DR equation is normally used in microporous materials (<2 nm) for the calculation of micropore volume, V0 and the monolayer equivalent surface area, SCO2, from the adsorption isotherm in the low relative pressure range (Marsh & Siemieniewska, Citation1965). This equation is expressed as:(8)
(8)
The linear form of Equation (8) is:(9)
(9)
where V is the volume of adsorbed gas (cm3/g) given in absolute temperature (T) and relative pressure (P/P0), V0 is the microporous volume (cm3/g), R is the gas constant, β is the affinity coefficient (0.35 for carbon dioxide) (Guillot & Stoeckli, Citation2001), and E (kJ/mol) is the characteristic energy for the interaction between the adsorbate and the surface.
According to the above equation, the plot of ln (V) against (ln2 (P0/P)) is a straight line having an intercept with the y-axis equal to the total micropore volume V0 and the slope equal to the characteristic energy E. The linearized DR plot is represented in Figure . The graphical representation of the DR equation is a descendant curve with decrease in pressure. Such appearance can easily be attributed to the formation of multilayer and capillary condensation. We can also notice that the linearity of the plot extends over a very wide range of relative pressures (0.006 < P/P0 < 0.04). In this region the process is unlikely to be due to the filling of the micropores but rather to the adsorption on the external surface and on the few mesopores. The mesoporous volume Vmes is obtained by the difference between the total V (P/P0 = 0.98) and V0. The linearization parameters are given in Table .
Table 4. Parameters of linearization of the DR and t-plot equation
It is found that DR model well describes our experimental data, because the regression coefficient is close to unity (R2 = 0.99). The characteristic energy E is equal to 20.9 kJ/mol, typical for a physisorption process and similar to other materials such as activated carbons (Sevilla, Falco, Titirici, & Fuertesa, Citation2012; Sevilla & Fuertes, Citation2011) and zeolithe (Ghezini, Sassi, & Bengueddach, Citation2008).
3.4.2. t-plot method
This method, developed by De-Boer and al., allows the determination of the microporous volume and the external surface of the solids as well. When using the “t-plot” for the study of microporosity, the choice of the correct “t-curve” is of major importance. For that purpose, we admit that at an adsorbed state assimilated to the liquid state at the same temperature. It is considered that molecule of CO2 occupies an area σ = 17 A2 at 295 K. The liquid state density of CO2 at 295 K is ρ = 0.7711 cm3/g (Raznjevic, Citation1970). The average thickness, “t”, of the adsorbed layers is given by the following equation:(10)
(10)
where N is the amount adsorbed (mol/g) at a given P/P0 value and Nm (mol/g) the amount adsorbed necessary to form a monolayer. Nm is determined using the BET equation. Finally, the “t” curve is plotted by representing the liquid volume of adsorbed CO2 gas as a function of the average thickness of adsorbed layer. The experimental graph is given in Figure . The intercept and the slope of the linear part of the t-curve for “t” values ranging from 13.2 up to 15.8 Å give microporous volume and the external surface, respectively. The results were summarized in Table .
Figure 7. The liquid volume of the adsorbed CO2 gas over silt versus the average thickness of adsorbate.
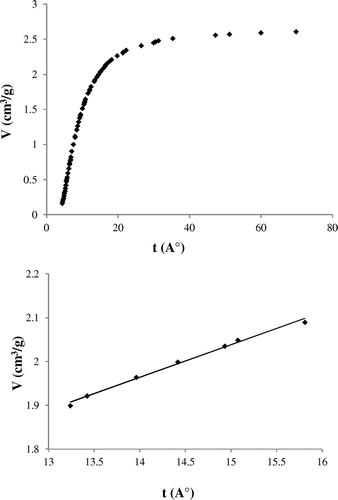
The linear t-plot range will be found at higher relative pressures and is dependent on the size distribution of micropores. The abrupt break in the two linear parts of the t-plot shown in Figure , indicates the presence of a group of micropores in a narrow pore size range, whereas the curvature between the two linear portions is an indication of a wider distribution of micropores (Lowell, Shields, Thomas, & Thommes, Citation2004).
Note that the microporous volume calculated by the t-plot is equal to 0.92 cm3/g, this value is in very good agreement with those found using the DR equation. Same results were obtained in case adsorption of CO2 onto zeolithe (Ghezini et al., Citation2008). In such micropores, adsorbed molecules interact with two surfaces as opposed to only one surface on the external surface. Thus, adsorption in micropores is stronger and occurs at lower pressures than on the external surface.
The external surface area Sext of the microporous sample can be derived from the slope of the t-plot, it was equal to 0.074 m2/g. This value appears to be very small compared to the total specific surface determined by BET (65.57 m2/g). The micropore surface area (Smicro) can be calculated from the relation Smicro = SBET − Sext (Lowell et al., Citation2004). It will be found equal to 65.49 m2/g. Vyas and Kumar utilized another method from micropore volume (V0 determined by DR method) using cross-sectional area (σ) of adsorbate molecule by solving following expression (Vyas & Kumar, Citation2004):(11)
(11)
where N is Avogadro’s number and is volume of 1 g mol of adsorbate at STP. Surface area calculated by DR equation is directly proportional to the value of cross-sectional area selected. Molecular cross-sectional area of carbon dioxide, based on liquid density data, ranges from 17 Å2 calculated by Emmett and Brunauer (Emmett & Brunauer, Citation1937) to 24.4 Å2 reported by Pickering and Eckstrom (Pickering & Eckstrom, Citation1952). A value of 17 Å2 was used for the studies reported here.
For this purpose, a carbon dioxide microporous surface area of 65 m²/g was found. This value is in reasonable agreement with the difference between the total (BET) surface area of 65.57 m²/g and the surface not in micropores (external surface) of 0.07 m²/g determined by the t-plot method (Table ). Therefore, it can be concluded that the carbon dioxide surface area of silt corresponds to the micropore surface area.
Given that the average pore diameter of the silt is 1.93 nm (determined in this study) and the molecular diameter of CO2 gas is 0.33 nm (Shieh & Chung, Citation1999). It is clear that the kinetic diameter of the gas molecules is very low compared to the pore diameter, allowing CO2 molecules to easily diffuse into the material. Therefore, the pore size is the key parameter that should be controlled in the design of porous sorbents for CO2 capture, trying to maximize the volume of narrow micropores.
3.5. Diffusion kinetic
Models based on the adsorbent structure used to estimate the distribution of gas in the micropores as the unipore model, which assumes that micropores in material have only one size (Bhowmik & Dutta, Citation2013; Nandi & Walker, Citation1970), and the bidisperse model, which assumes two sizes: micropore and macropore (Cui, Bustin, & Dipple, Citation2004; Smith & Williams, Citation1984). In this study, assuming that the pores of the silt are homogeneous, therefore, the application of the model unipore is more judicious.
For very small values of time t and for homogeneous spherical particles, the relationship between the effective diffusivity De (s−1), time t (s), gas uptake Mt (g), and total gas uptake M∞ (g) can be approximated to (Crank, Citation1975):(12)
(12)
A plot of Mt/M∞ vs. t1/2 will have a slope of 6 (De/π)1/2, experimental data for the sorption kinetic of CO2 on silt was shown in Figure . The effective diffusivity De is related to the diffusion coefficient D, according to Smith and Williams (Citation1984):(13)
(13)
Figure 8. CO2 adsorption kinetic: (a) up to equilibrium time, and (b) for small times corresponding to Mt/M∞ < 0.5.
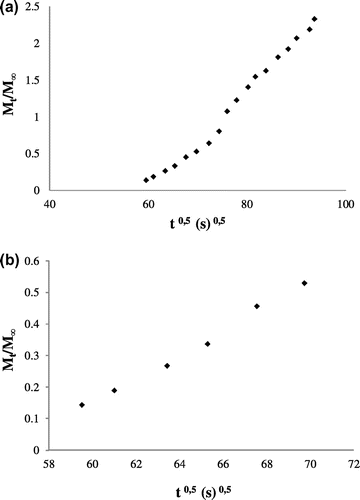
with D the diffusion coefficient (m2/s) and r0 the diffusion path length (m) but this parameter is difficult to measure. If the average particle radius is substituted for r0, the diffusion coefficient can be calculated.
As shown in Figure for CO2 adsorption kinetic, the gas uptake depends linearly on t1/2, for small times corresponding to Mt/M∞ < 0.5. Equilibrium was established after two hours thirty minutes of contact between the gas and the adsorbent at ambient temperature and atmospheric pressure. This duration remains low to that found during CO2 adsorption on coal, equilibrium is reached at 40 h (0.1 MPa) and 15 h (5 MPa) at ambient temperature (Charrière, Citation2009). The equilibrium depends on the pressure, it decreases when it increases.
Therefore, the adsorption kinetics follows Fick’s law. From the slope of straight line (Mt/M∞ < 0.5), the effective diffusivity, De, and diffusion, D, were estimated and were reported in Table .
Table 5. Diffusion parameters for CO2 adsorption onto silt
From this table, we note that the effective diffusion coefficient of CO2 adsorption at atmospheric pressure is about 1.26 × 10−4 s−1. Applying the unipore model, effective diffusivities have been estimated at between 10−3 and 10−4 s−1 for the adsorption of CO2 on the dry coal to a pressure range of 0.3–0.6 MPa (Charrière, Citation2009; Clarkson & Bustin, Citation1999). The diffusion coefficient, D, is on the order of 0.18 × 10−12 m2 s−1. This value is lower than that obtained in the case of CO2 adsorption by the coal; they estimated between 0.54 and 1.9 × 10−12 m2 s−1 under pressure ranging from 0.1 to 5 MPa. It is noted that the diffusion coefficient D, increase as the pressure (Charrière, Citation2009).
Siemons, Wolf, and Bruining (Citation2007) have assumed superimposition of fast and slow processes and estimated coefficients which are between 10−8 and 10−10 m2 s−1 for the fast process. The slow diffusion rate is about one to two orders of magnitude lower, i.e. between 10−9 and 10−12 m2 s−1. By comparison, our low diffusion coefficients would be consistent with a slow diffusion process control of the gas uptake by the silt.
In previous study, Charriere estimated a CO2 diffusion time at 5000 years in a coal sphere diameter of 1 m and diffusion coefficient of 10−12 m2 s−1. Assuming that the diffusion is strictly Fickian and the coal not including any fracture (no flow) (Charrière, Citation2009). By analogy, we can evaluate CO2 storage time in the silt after their recovery, following the same assumptions. For a silt sphere 10 cm in diameter and D value equal to 10−12 m2 s−1, the time taken for the gas to travel that distance is about 1010 s, that is to say 500 years. This time is very important for the storage of CO2 in the silt, but this calculation is approximate. It gives us an idea about the possibility of using the silt as a CO2 sequestration deposit. In reality and in a large scale, we must take into account several parameters especially the pressure, temperature, physic-chemical properties of materials such as permeability, grain size.
3.6. Gibbs energy change
If gas phase ideality is assumed, when 1 mol of gaseous sorbate is transferred reversibly and isothermally from the gas phase at standard pressure, P0, to infinite amount of sorbate–sorbent mixture at equilibrium pressure, P, than the decrease in the Gibbs energy is given as (Sigg, Behra, & Stumm, Citation2006):(14)
(14)
where ∆G is the Gibbs free energy (J/mol), R is the universal gas constant (J mol/K), and T is the temperature (K). The change in the Gibbs energy ∆G as a function of amount adsorbed at the temperature 295 K is shown in Figure .
The Gibbs energy appears as a decreasing function of the amount adsorbed. The free energy of adsorption is negative it means that the adsorption of carbon dioxide on silt is a thermodynamically spontaneous. The adsorption energy decreases from 6.47 to 2.29 kcal/mol, with the increase of the amount adsorbed, this may be due to the heterogeneity of the surface. Once the active sites are saturated, a further step (linear portion) was observed at about 2.29 kJ/mol corresponds to filling of remaining micropore. Finally, the decrease in heat to 0.04 kJ/mol indicating the accomplishment of the micropore filled.
4. Conclusion
In this work, the adsorption of carbon dioxide at low pressure and at the temperature of 295 K in silt is studied. XRD analysis shows that this material origin Chorfa deposit contains some minerals such as calcite, quartz, and clays. The nature of these clays is illite, with the existence of very small quantity of the kaolinite. The obtained results show that the dam silt can be used as an adsorbent for the capture of carbon dioxide. The adsorption capacity of CO2 on silt was estimated as 2.68 cm3/g at atmospheric pressure and room temperature. The Sips adsorption isotherm was found to have the best fit to the experimental data that means the surface is heterogeneous as well as formation of multilayer with interactions between the adsorbed molecules. The micropore volume of adsorbent was estimated by the DR equation and t-plot method. The results obtained were in very good agreement. The carbon dioxide surface area of this material corresponds to the micropore surface area. The characteristic energy E determined by the DR equation is equal to 20.9 kJ/mol, this value indicates that the adsorption is physical in nature. Kinetic results revealed that the diffusion of the gas in our material was a slow process, as the diffusion coefficient estimated at 10−12 m2 s−1 is relatively low. Thermodynamic study found that the recovery of CO2 by the silt is a spontaneous process. Finally, we conclude that dam silt can be used as an effective and low-cost microporous adsorbent to capture carbon dioxide and minimized their concentration in atmosphere.
Acknowledgments
Many thanks to Pr. A. Bengueddach, Director of Laboratory of Chemistry Materials, University of Oran, Algeria for his helpful suggestions to achieve the BET analysis of CO2 adsorption.
Additional information
Funding
Notes on contributors
Fatima Ouadjenia
Fatima Ouadjenia works as a researcher teacher at Mascara University, Technical Science Department.
Ouadjenia received her Engineering degree in Chemical Engineering in 2001 from Mostaganem University, Mostaganem, Algeria, and her Magister in Environmental Engineering in 2004. She received her Doctorate in environmental Chemistry in 2014 from Sidi Bel ABBES University, Sidi Bel ABBES, Algeria. Ouadjenia is a member in the Laboratory of Material, Applications and Environment (LMAE), University of Mascara, Algeria.
References
- Azzouz, A., Assaad, E., Ursu, A. V., Sajin, T., Nistor, D., & Roy, R. (2010). Carbon dioxide retention over montmorillonite-dendrimer materials. Applied Clay Science, 48, 133–137.10.1016/j.clay.2009.11.021
- Balsamo, M., Di Natale, F., Erto, A., Lancia, A., Montagnaro, F., & Santoro, L. (2011). Cadmium adsorption by coal combustion ashes-based sorbents—Relationship between sorbent properties and adsorption capacity. Journal of Hazardous Materials, 187, 371–378.10.1016/j.jhazmat.2011.01.029
- Behvandi, A., & Taurani, S. (2011). Equilibrium modeling of carbon dioxide adsorption zeolites. World Academy of Science, Engineering and Technology, 52, 617–619.
- Belin, T., Mve Mfoumou, C., Mignard, S., & Pouilloux, Y. (2013). Study of physisorbed carbon dioxide on zeolites modified by addition of oxides or acetate impregnation. Microporous and Mesoporous Materials, 182, 109–116.10.1016/j.micromeso.2013.08.020
- Bhattacharyya, K. G., & Gupta, S. S. (2008). Influence of acid activation on adsorption of Ni(II) and Cu(II) on kaolinite and montmorillonite: Kinetic and thermodynamic study. Chemical Engineering Journal, 136, 1–13.10.1016/j.cej.2007.03.005
- Bhowmik, S., & Dutta, P. (2013). Adsorption rate characteristics of methane and CO2 in coal samples from Raniganj and Jharia coal fields of India. International Journal of Coal Geology, 113, 50–59.10.1016/j.coal.2013.02.005
- Blamey, J., Al-Jeboori, M. J., Manovic, V., Fennell, P. S., & Anthony, E. J. (2016). CO2 capture by calcium aluminate pellets fluidized bed. Fuel Processing Technology, 142, 100–106.10.1016/j.fuproc.2015.09.015
- Chalal, N., Bouhali, H., Hamaizi, H., Lebeau, B., & Bengueddach, A. (2015). CO2 sorption onto silica mesoporous materials made from nonionic surfactants. Microporous and Mesoporous Materials, 210, 32–38.10.1016/j.micromeso.2015.02.016
- Charrière, D. (2009). Characterization of gas sorption on coals, Application to the geological storage of carbon dioxide into coal seams (PhD thesis). University of Toulouse, Toulouse.
- Clarkson, C. R., & Bustin, R. M. (1999). The effect of pore structure and gas pressure upon the transport properties of coal: A laboratory and modeling study, 2. Adsorption rate modeling. Fuel, 78, 1345–1362.
- Crank, J. (1975). The mathematics of diffusion (2nd ed.). London: Oxford University Press.
- Cui, X., Bustin, R. M., & Dipple, G. (2004). Selective transport of CO2, CH4 and N2 in coals: Insights from modeling of experimental gas adsorption data. Fuel, 83, 293–303.10.1016/j.fuel.2003.09.001
- De Boer, J. H. (1953). The dynamical character of adsorption. London: Oxford University Press.
- Deboer, J. H., Linsen, B. G., & Osinga, T. J. (1965). Studies on pore systems in catalysts: VI. The universal t curve. Journal of Catalysis, 4, 643–648.10.1016/0021-9517(65)90263-0
- Dubinin, M. M., & Radushkevich, L. V. (1947). Sorption and structure of active carbons, I. Adsorption of organics vapors. Zhurnal Fizicheskoi Khimii, 21, 1351–1362.
- Dutta, P., Harpalani, S., & Prusty, B. (2008). Modeling of CO2 sorption on coal. Fuel, 87, 2023–2036.10.1016/j.fuel.2007.12.015
- Emmett, P. H., & Brunauer, S. (1937). The use of low temperature van der Waals adsorption isotherms in determining the surface area of iron synthetic ammonia catalysts. Journal of the American Chemical Society, 59, 1553–1564.10.1021/ja01287a041
- Figueroa, J., Fout, T., Plasynski, S., McIlvried, H., & Srivastava, R. (2008). Advances in CO2 capture technology – The U.S Department of Energy’s Carbon Sequestration Program. International Journal of Greenhouse Gas Control, 2, 9–20.10.1016/S1750-5836(07)00094-1
- Freundlich, H. (1926). Colloid and capillary chemistry. London: Methuen.
- Gallucci, K., Stendardo, S., & Foscolo, P. U. (2008). CO2 capture by means of dolomite in hydrogen production from syngas. International Journal of Hydrogen Energy, 33, 3049–3055.10.1016/j.ijhydene.2008.03.039
- Gao, Y., Yue, Q., Gao, B., Sun, Y., Wang, W., Li, Q., & Wang, Y. (2013). Comparisons of porous, surface chemistry and adsorption properties of carbon derived from Enteromorpha prolifera activated by H4P2O7 and KOH. Chemical Engineering Journal, 232, 582–590.
- Ghezini, R., Sassi, M., & Bengueddach, A. (2008). Adsorption of carbon dioxide at high pressure over H-ZSM- type zeolite. Micropore volume determinations by using the Dubinin-Raduskevich equation and the ‘‘t-plot” method. Microporous and Mesoporous Materials, 113, 370–377.10.1016/j.micromeso.2007.11.034
- Guillot, A., & Stoeckli, F. (2001). Reference isotherm for high pressure adsorption of CO2 by carbons at 273 K. Carbon, 39, 2059–2064.10.1016/S0008-6223(01)00022-7
- Hao, W., Björkman, E., Lilliestråle, M., & Hedin, N. (2013). Activated carbons prepared from hydrothermally carbonized waste biomass used as adsorbents for CO2. Applied Energy, 112, 526–532.10.1016/j.apenergy.2013.02.028
- Hefti, M., Marx, D., Joss, L., & Mazzotti, M. (2015). Adsorption equilibrium of binary mixtures of carbon dioxide and nitrogen on zeolites ZSM-5 and 13X. Microporous Mesoporous Materials, 215, 215–228. doi:10.1016/j.micromeso.2015.05.044
- Hill, T. L. (1946). Localized and mobile adsorption and absorption. Journal of Chemical Physics, 14, 1441.
- Hong, W. Y., Perera, S. P., & Burrows, A. D. (2015). Manufacturing of metal-organic framework monoliths and their application in CO2 adsorption. Microporous and Mesoporous Materials, 214, 149–155.10.1016/j.micromeso.2015.05.014
- House, W. A., & Jaycock, M. J. (1974). A study of the surface heterogeneity of an anatase sample. Journal of Colloid Interface Science, 47, 50–58.10.1016/0021-9797(74)90078-2
- IUPAC Commission on Colloid and Surface Chemistry. (1994). Pure Applied Chemistry, 66, 1739.
- Keramati, M., & Ghoreyshi, A. A. (2014). Improving CO2 adsorption onto activated carbon through functionalization by chitosan and triethylenetetramine. Physica E: Low-Dimensional Systems and Nanostructures, 57, 161–168. doi:10.1016/j.physe.2013.10.024
- Khelifa, A., Benchehida, L., & Derriche, Z. (2004). Adsorption of carbon dioxide by X zeolites exchanged with Ni2+ and Cr3+: Isotherms and isosteric heat. Journal of Colloid and Interface Science, 278, 9–17.10.1016/j.jcis.2004.05.033
- Khelifa, A., Derriche, Z., & Bengueddach, A. (1999). Sorption of carbon dioxide by zeolite X exchanged with Zn2+ and Cu2+. Microporous and Mesoporous Materials, 32, 199–209.10.1016/S1387-1811(99)00107-9
- Langmuir, I. (1918). The adsorption of gases on plane surfaces of glass, mica and platinum. Journal of the American Chemical Society, 40, 1361–1403.10.1021/ja02242a004
- Li, F., & Fan, L. (2008). Clean coal conversion processes—Progress and challenges. Energy Environmental Science, 1, 248–267.10.1039/b809218b
- Lowell, S., Shields, J. E., Thomas, M. A., & Thommes, M. (2004). Characterization of porous solids and powders: Surface area, pore size and density (p. 16). Netherlands: Springer.10.1007/978-1-4020-2303-3
- Marsh, H., & Siemieniewska, T. (1965). The surface area of coals as evaluated from the adsorption isotherms of carbon dioxide using Dubinin-Polanyi equation. Fuel, 44, 355–367.
- Metz, B., Davidson, O., Coninck, H., Loos, M., & Meyer, L. (2005). Carbon dioxide capture and storage. Cambridge: University Press.
- Montagnaro, F., Silvestre-Albero, A. S., Silvestre-Albero, J. S., Rodríguez-Reinoso, F. R., Erto, A., Lancia, A., & Balsamo, M. (2015). Post-combustion CO2 adsorption on activated carbons with different textural properties. Microporous and Mesoporous Materials, 209, 157–164.10.1016/j.micromeso.2014.09.037
- Nandi, S., & Walker, P. (1970). Activated diffusion of methane in coal. Fuel, 49, 309–323.10.1016/0016-2361(70)90023-2
- Ouadjenia-Marouf, F., Marouf, R., Schott, J., & Yahiaoui, A. (2013). Removal of Cu(II), Cd(II) and Cr(III) ions from aqueous solution by dam silt. Arabian Journal of Chemistry, 6, 401–406.10.1016/j.arabjc.2010.10.018
- Pickering, H. L., & Eckstrom, H. C. (1952). Physical adsorption of gases on anatase. Journal of the American Chemical Society, 74, 4775–4777.10.1021/ja01139a014
- Pillai, R. S., Peter, S. A., & Jasra, R. V. (2008). Adsorption of carbon dioxide, methane, nitrogen, oxygen and argon in NaETS-4. Microporous and Mesoporous Materials, 113, 268–276.10.1016/j.micromeso.2007.11.042
- Pires, J., Bestilleiro, M., Pinto, M., & Gil, A. (2008). Selective adsorption of carbon dioxide, methane and ethane by porous clays heterostructures. Separation Purification Technology, 61, 161–167.10.1016/j.seppur.2007.10.007
- Polanyi, M. (1914). Adsorption and capillarity from standpoint of 2nd law of thermodynamics. Verhandt. Deut. Phys. Ges., 16, 1012.
- Raznjevic, K. (1970). Thermodynamic tables and diagrams (p. 558). Paris: Eyrolles.
- Ruthven, D. M. (1984). Principles of adsorption and adsorption processes. New York, NY: Wiley Interscience.
- Samanta, A., Zhao, A., Shimizu, G., Sarkar, P., & Gupta, R. (2012). Post-combustion CO2 capture using solid sorbents: A review. Industrial & Engineering Chemistry Research, 51, 1438–1463.10.1021/ie200686q
- Sari, A., Tuzen, M., Citak, D., & Soylak, M. (2007). Equilibrium, kinetic and thermodynamic studies of adsorption of Pb(II) from aqueous solution onto Turkish kaolinite clay. Journal of Hazardous Materials, 149, 283–291.10.1016/j.jhazmat.2007.03.078
- Sayari, A., Belmabkhout, Y., & Serna-Guerrero, R. (2011). Flue gas treatment via CO2 adsorption. Chemical Engineering Journal, 171, 760–774.10.1016/j.cej.2011.02.007
- Sevilla, M., Falco, C., Titirici, M. M., & Fuertesa, A. (2012). High-performance CO2 sorbents from algae. Royal Society of Chemistry Advances, 2, 12792–12797.
- Sevilla, M., & Fuertes, A. B. (2011). Sustainable porous carbons with a superior performance for CO2 capture. Energy & Environmental Science, 4, 1765–1771.10.1039/c0ee00784f
- Sharma, Y. C. (2008). Thermodynamics of removal of cadmium by adsorption on an indigenous clay. Chemical Engineering Journal, 145, 64–68.10.1016/j.cej.2008.03.006
- Shieh, J. J., & Chung, T. S. (1999). Gas permeability, diffusivity, and solubility of poly(4- vinylpyridine) film. Journal of Polymer Science Part B: Polymer Physics, 37, 2851–2861.10.1002/(ISSN)1099-0488
- Siemons, N., Wolf, K.-H. A.A., & Bruining, J. (2007). Interpretation of carbon dioxide diffusion behavior in coals. International Journal of Coal Geology, 72, 315–324.10.1016/j.coal.2007.04.004
- Sigg, L., Behra, P., & Stumm, W. (2006). Chemistry of aquatic environments (4th ed.). Paris: Dunod.
- Sing, K. S. W. (1973). Colloid science 1. London: The Chemical Society.
- Sips, R. (1948). On the structure of a catalyst surface. The Journal of Chemical Physics, 16, 490–495.10.1063/1.1746922
- Siriwardane, R. V., Shen, M. S., & Fisher, E. P. (2003). Adsorption of CO2, N2 and O2 on natural zeolites. Energy & Fuels, 17, 571–576.10.1021/ef020135l
- Smith, D. M., & Williams, F. L. (1984). Diffusion models for gas production from coal. Fuel, 63, 256–261.10.1016/0016-2361(84)90047-4
- Song, C. (2006). Global challenges and strategies for control, conversion and utilization of CO2 for sustainable development involving energy, catalysis, adsorption and chemical processing. Catalysis Today, 115, 2–32.10.1016/j.cattod.2006.02.029
- Tamajón, F. J., Álvarez, E., Cerdeira, F., & Gómez-Díaz, D. (2016). CO2 absorption into N-methyldiethanolamine aqueous-organic solvents. Chemical Engineering Journal, 283, 1069–1080.10.1016/j.cej.2015.08.065
- Vilarrasa-Garcia, E., Moya, E. M., Cecilia, J. A., Cavalcante Jr., C. L., Jiménez- Jiménez, J., & Azevedo, D. C. ssS. (2015). CO2 adsorption on amine modified mesoporous silicas: Effect of the progressive disorder of the honeycomb arrangement. Microporous and Mesoporous Materials, 209, 172–183.10.1016/j.micromeso.2014.08.032
- Vyas, R. K., & Kumar, S. S. (2004). Determination of micropore volume and surface area of zeolite molecular sieves by DR and DA equation: A comparative study. Indian Journal of Chemical Technology, 11, 704–709.
- Wang, M., Joel, A. S., Ramshaw, C., Eimer, D., & Musa, N. M. (2015). Process intensification for post-combustion CO2 capture with chemical absorption: A critical review. Applied Energy, 158, 275–291.10.1016/j.apenergy.2015.08.083
- Wang, Y., Zhou, Y., Liu, C., & Zhou, L. (2008). Comparative studies of CO2 and CH4 sorption on activated carbon in presence of water. Colloids and Surfaces A: Physicochemical and Engineering Aspects, 322, 14–18.10.1016/j.colsurfa.2008.02.014
- Xu, G., Li, L., Yang, Y., Tian, L., Liu, T., & Zhang, K. (2012). A novel CO2 cryogenic liquefaction and separation system. Energy, 42, 522–529.10.1016/j.energy.2012.02.048
- Yang, H., Xu, Z., Fan, M., Gupta, R., Slimane, R. B., Bland, A. E., & Wright, I. (2008). Progress in carbon dioxide separation and capture: A review. Journal of Environmental Sciences, 20, 14–27.10.1016/S1001-0742(08)60002-9
- Yan, S. P., Fang, M. X., Zhang, W. F., Zhong, W. L., Luo, Z. Y., & Cen, K. F. (2008). Comparative analysis of CO2 separation from flue gas by membrane gas absorption technology and chemical absorption technology in China. Energy Conversion and Management, 49, 3188–3197.10.1016/j.enconman.2008.05.027
- Yue, M. B., Sun, L. B., Cao, Y., & Wang, Z. J. (2008). Promoting the CO2 adsorption in the amine-containing SBA-15 by hydroxyl group. Microporous and Mesoporous. Materials, 114, 74–81.10.1016/j.micromeso.2007.12.016