Abstract
To investigate the symbiotic interaction of Trichoderma harzianum Rifai on Chenopodium quinoa Willd. in isolation, we studied axenic co-culture of the T. harzianum isolates T-22 and BOL-12QD and the C. quinoa cultivars Kurmi and Maniqueña real. Neither T-22 nor BOL-12QD affected seedling growth during two days of co-culture in the early growth phase of rapid primary root extension. However, after longer axenic co-culture, T-22 and BOL-12 were found to significantly inhibit the overall growth of C. quinoa cv. Kurmi and Real, affecting also vitality parameters as seen for chlorophyll and betalains. Lateral root development was strongly inhibited in all plant−fungal combinations, leaving stunted lateral roots. These results suggest that T. harzianum has a general capacity to inhibit the growth of C. quinoa plants with a main effect on the lateral root development.
PUBLIC INTEREST STATEMENT
Cultivation of quinoa (Chenopodium quinoa) is expanding because it has a high nutrient content grain. Quinoa has a high abiotic stress resistance but is sensitive to pathogens. The fungus Trichoderma harzianum is a biocontrol agent widely used against agricultural diseases, because it can enhance plant growth, counteract detrimental microbes and activate the plant immune system. However, the mechanism of plant−Trichoderma interactions is not completely understood, and some plant varieties can even experience negative effects from these fungi. We have developed a sterile co-culture system for two Trichoderma strains and two quinoa cultivars. T. harzianum did not affect seedling growth during two days of co-culture, but over longer time, they inhibited the overall growth of quinoa. Lateral root development was strongly inhibited in all plant−fungal combinations. Thus, T. harzianum has the capacity to inhibit the growth of C. quinoa plants in long co-culture under sterile conditions.
Competing Interests
The authors declares no competing interests.
1. Introduction
Chenopodium quinoa Willd. (Amaranthaceae) is an emerging crop because of its high nutritional value combined with its ability to endure and grow under harsh cultivation conditions (Bazile et al. Citation2016; Jacobsen, Mujica, & Jensen, Citation2003; Ruiz et al., Citation2014; Vega-Gálvez et al., Citation2010). However, quinoa crops in highlands, e.g. in the Andean plateau, are heavily affected by the downy mildew disease caused by the oomycete Peronospora variabilis (Danielsen & Ames, Citation2000; Danielsen, Bonifacio, & Ames, Citation2003; Gandarillas, Saravia, Plata, Quispe, & Ortiz-Romero, Citation2015; Testen, Del Mar Jiménez-Gasco, Ochoa, & Backman, Citation2014), which can result in drastic yield reductions (Danielsen & Munk, Citation2004). In order to halt the development of the downy mildew disease, heavy loads of chemical pesticide are being applied, but this is not the solution for countries like Bolivia that have chosen an organic production. Therefore, novel organic solutions like biological control strategies need to be developed. In this respect, Trichoderma, a fungal genus known for improving crop production, could be an interesting option to alleviate the damages caused by the downy mildew disease as observed for other crops (Nagaraju, Sudisha, Murthy, & Ito, Citation2012; Nandini, Hariprasad, Niranjana, Shetty, & Geetha, Citation2013; Perazzolli et al., Citation2012).
Trichoderma is known by many beneficial effects on plants like promoting plant growth, inducing plant systemic resistance and also directly antagonizing phytopathogens (Harman, Howell, Viterbo, Chet, & Lorito, Citation2004; Kubicek et al., Citation2011). Therefore, strains like Trichoderma harzianum T-22 are in commercial use for several crops (Contreras-Cornejo, Macías-Rodríguez, del-Val, & Larsen, Citation2016; Harman, Citation2011). However, studies of maize and tomato growth promotion by T-22 have shown a highly variable response depending on the plant genotype and its combination with Trichoderma strains (Harman, Citation2006; Schuster & Schmoll, Citation2010; Tucci, Ruocco, De Masi, De Palma, & Lorito, Citation2011). Hence, the optimal Trichoderma effect will depend on the choice of plant cultivar and Trichoderma strains, e.g. Trichoderma strains from the same species from different geographic regions might cause different growth effects. Native strains of Trichoderma from quinoa soils (T. harzianum and Trichoderma koningiopsis) have been reported to improve agricultural quinoa yields (Ortuño et al., Citation2013; Ortuño, Castillo, Miranda, Claros, & Soto, Citation2016). These two strains as well as a recent T. harzianum BOL-12QD were also shown to have antimycotic properties (Espinal Churata, Huanca, Terrazas Siles, & Giménez Turba, Citation2010; García-Espejo, Mamani-Mamani, Chávez-Lizárraga, & Álvarez-Aliaga, Citation2016).
To gain more detail on the interaction of C. quinoa and T. harzianum in isolation, we have here developed a system for axenic co-culture of C. quinoa and two biocontrol strains T-22 and BOL-12QD that allow both shoot and root analysis. The outcome of these interactions produced a general growth inhibition, where a lateral root growth inhibition appears to be the main cause.
2. Materials and methods
2.1. Biological material
Seeds of quinoa (Chenopodium quinoa Willd.) cultivars Maniqueña Real (Real) and Kurmi were kindly supplied by PROINPA (Quipaquipani, Bolivia). Trichoderma harzianum, Rifai, T-22, anamorph ATCC 20847 originated from a protoplast fusion of T12m, isolated from NY, USA (Hadar, Harman, & Taylor, Citation1984) and T95, which is a UV-induced mutant of T-Co isolated from Bogotá, Colombia (Ahmad & Ralph, Citation1987; Chet & Baker, Citation1981; Stasz, Harman, & Weeden, Citation1988). T-22 was purchased from the American Type Culture Collection (Manassas, VA, USA). Trichoderma harzianum BOL-12QD (BOL-12) was isolated and provided by the Instituto de Investigaciones Farmaco-bioquímicas (IIFB-UMSA, La Paz, Bolivia).
2.2. Fungal growth
T-22 and BOL-12QD were maintained on potato dextrose agar (BD-Difco, Detroit, USA) at 25°C. To isolate spore suspensions, 1 mL of sterile water was added to 2-week-old Trichoderma cultures on potato dextrose agar and collected conidia were filtered through a sterile piece of absorbent cotton. The spores were washed twice with sterile ddH2O and pelleting at 3700g for 5 min at 4°C in an Allegra X-12R centrifuge (Beckman, Brea, CA, USA). Spores were resuspended in sterile ddH2O and kept at 4°C until experiments.
Germination of T-22 and BOL-12QD spores for C. quinoa treatment was performed as described by Yedidia, Benhamou, and Chet (Citation1999) using 15 mL tubes shaken at 200 rpm for 18 h. The germinated spore suspension was washed twice by centrifugation as described above and finally resuspended in sterile ddH2O. The final spore concentration was adjusted to be 1 germinated spore/µl and verified by colony forming unit (CFU) counts on potato dextrose agar Petri dishes.
For DNA extraction, growth tubes with 10 mL of Potato Dextrose Broth (BD-Difco, Detroit, USA) were inoculated with Trichoderma harzianum BOL-12QD and incubated for two days at 25°C in darkness. The biomass developed (<100 mg) was transferred to a microcentrifuge tube for later use.
2.3. Sterilization of C. quinoa seedlings and germination
Seeds of C. quinoa were surface-sterilized by soaking in commercial bleach (NaClO; 27 gkg-1) for 20 min., followed by 6 rinses in sterile ddH2O. Immediately thereafter, the seeds were placed on sterile water agar (8 gL-1) in Petri dishes and incubated in darkness at 24°C for 14 h.
2.4. Co-culture of quinoa and T. harzianum in growth boxes
Three germinated axenic seedlings of each cultivar Kurmi and Real with similar root length were aligned on a straight line in 11.4 cm × 8.6 cm × 10.2 cm growth boxes (Phytatray II, Sigma). These contained 0.1× Murashige and Skoog Basal Salts Mixture (MS; Duchefa, Haarlem, The Netherlands), supplemented with 8 gL-1 agar in which the agar medium had solidified while the boxes were tilted to 45°. The seedlings were incubated at 24°C for 4 h under regular light (fluorescent tubes; 50 µmol m−2s−1) prior to T-22 and BOL-12QD treatment.
C. quinoa seedlings were treated by adding 10 µL [1 CFU µL-1] of either T-22 or BOL-12QD germinated spore suspension on top of the neck of the primary root. Ten microliters of sterile ddH2O were added to each seedling in the mock control group. After treatment, the seedlings were incubated for 14 days at 24°C under regular light with a 16 h light/8 h darkness photoperiod.
2.5. Co-culture of quinoa and T. harzianum in Petri dishes
Co-culture of T. harzianum and C. quinoa on square Petri dishes were carried out as for the growth box system, with the following changes: After germination, 5 seedlings of each cultivar (Kurmi and Real) with similar length were aligned in a straight line on 12 × 12 cm square Petri dishes containing 0.1× MS, 8 gL-1 agar, in which the agar had been solidified with the Petri dishes in a horizontal position. The Petri dishes were then tilted 45° during growth with the agar/air interface facing upwards and seedlings having the roots pointing towards the bottom part of the Petri dish. The seedlings were incubated at 24° C for 4 h under regular lights and then treated with T-22 or BOL-12QD as described above. After treatment, the seedlings were incubated at 24°C in a 16 h light/8 h darkness photoperiod either at regular or at high light intensity.
2.6. Co-culture under different light systems in Petri dishes
Co-cultivation under regular light intensity was done with fluorescent lights (Polylux XLr 30W, GE, Budapest, Hungary) 50 µmol m−2s−1 for 2 and 7 days. Co-cultivation under higher light intensity was done with white LED growth lights (UFO LED Grow Light 90W, JDSweden AB, Råå, Sweden) 175 µmol m−2s−1 for 10 and 6 days. For six days of co-cultivation, growth incubation was carried out as described above but on larger square Petri dishes (24 × 24 cm).
2.7. Seedling growth analysis
Main root length, shoot length, hypocotyl length, lateral root number and lateral root length were analyzed through images taken with a Digital Camera Canon EOS Rebel T3. Measurements from the photographs were done with the segmented line tool of ImageJ 1.49 (Abramoff, Magalhães, & Ram, Citation2004). For fresh weight analysis, shoots were separated from the roots with a scalpel and mass determined using an analytical scale (Sartorius ED 124S, Goettingen, Germany). Root weights were not determined because of lateral root damage upon lifting. All seedlings with an incomplete expansion (cotyledons remaining attached to the seed husk) were discarded from analysis.
2.8. Chlorophyll and betalain determination
Intact whole shoots were placed in a pre-cooled mortar and ground under liquid nitrogen. One milliliter of water was added and the whole sample recovered and centrifuged at 12,300g for 5 min. The supernatant was used for betalain quantification and the pellet for chlorophyll quantification.
Betalain concentration was determined according to Castellar, Obón, Alacid, and Fernández-López (Citation2003) from the absorbance at 535 nm in a Multiskan GO plate reader (Thermo Fisher Scientific, Vantaa, Finland), using an extinction coefficient of E1%1cm = 1120 (Kujala, Loponen, & Pihlaja, Citation2001).
For chlorophyll determination, the pellet was resuspended with acetone to a final concentration of 80% (v/v), left in the dark for 1 min and centrifuged for 5 min. The absorbance was read at 645, 663 and 750 nm in a Multiskan GO plate reader (Thermo Fisher Scientific, Vantaa, Finland). The total chlorophyll in the seedling was calculated according to Ni, Kim, and Chen (Citation2009): μg chl/mg tissue = 8.02 × (A663 − A750) + 20.2 × (A645 − A750)] × (V/1000) × W based on Arnon, Allen, and Whatley (Citation1954).
2.9. Fungal DNA extraction and PCR
For DNA extraction, fungal biomass was collected in microcentrifuge tubes, frozen with liquid nitrogen and ground with a disposable tissue grinder. Then, 400 µL of lysis solution buffer AP1 (Qiagen, Valencia, CA, USA) was added, vortexed and ground again. The remaining procedure was carried out according to the manufacturer’s instructions using DNeasy Plant Mini Kit (Qiagen, Valencia, CA, USA).
PCR of the Internal Transcribed Spacer (ITS) region was done with primer pairs ITS1/ITS4 as described by White, Bruns, Lee, and Taylor et al. (Citation1990) using DreamTaq polymerase (Thermo Scientific, Carlsbad, CA, USA) supplemented with 0.2 mM dNTP Mix (Thermo Scientific), 1.25 mM MgCl2 and with 0.25 µM of each primer. The PCR program had the following conditions: 1 cycle of: 95°C, 5 min; 30 cycles of 95° C, 30 s; 53°C, 30s; 72°C, 45s; 1 final cycle of 72°C for 5 min.
2.10. ITS sequencing
PCR products (150 ng) from the ITS region of the biocontrol strain T. harzianum BOL-12QD were directly sequenced by the Sanger method (Eurofins, Ebersberg, Germany) and confirmed for the complementary strand. T. harzianum BOL-12QD ITS sequence was deposited in the NCBI GenBank under accession number KY644517.1.
2.11. Statistics
Data of each set of experiments was analyzed separately for cultivars and was carried out by one-way analysis of variance. Statistical differences between treatments were tested with Tukey’s HSD post-hoc test. Student’s t test was used to compare controls of Kurmi and Real. Statistical analysis was carried out using R packages plyr (Wickham, Citation2011) and stats (R Core Team, Citation2016). Images were produced using ggplot2 (Wickham Citation2016).
3. Results
3.1. Molecular identification of T. harzianum BOL-12QD by ITS sequencing
In order to verify the native Trichoderma BOL-12QD isolate with molecular tools and provide a barcode, we sequenced the ITS region. The BOL-12QD strain, previously annotated as Trichoderma inhamatum based on a morphological description (Espinal Churata et al., Citation2010), had an ITS sequence that was 524 bp long and had a match identity of 100% to 13 T. harzianum accessions registered at NCBI. Eleven nucleotides varied as compared to the T-22 ITS region. Therefore, the fungal BOL-12QD isolate is classified as T. harzianum BOL-12QD.
3.2. Growth of C. quinoa in axenic systems for treatment withT. harzianum
The effects of T-22 or BOL-12QD on C. quinoa in isolation were studied through axenic growth systems, where C. quinoa seedlings were grown on 0.1× MS and 0.8% agar in square Petri dishes or in growth boxes. To allow analyses of root growth on an agar surface, we made initial test with square Petri dishes standing vertically. However, seedling primary root tips were prone to lift from the agar surface and grow into the air, with consequentially restricted primary root growth. In contrast, in square Petri dishes tilted 45°, and in growth boxes with a similarly slanted agar surface, the roots followed the agar surface with a minimum of root lifting into the air phase or root growth into the agar medium. In this system, the seedlings grew fast and homogenously, extending to 8.0 ± 0.3 cm and 8.6 ± 0.3 cm (mean ± SE; n = 15) in Kurmi and Real, respectively, over 2 days of cultivation (data not shown).
3.3. Short-term treatment of C. quinoa with T-22 and BOL-12QD
C. quinoa seedlings of 18 h old and having similar sizes were treated with a T-22 or BOL-12QD germinated spore suspension or mock control and incubated for two days under regular light in square Petri dishes (Figure ). The primary root length of each cultivar 2 days postinoculation (dpi) with T-22 or BOL-12QD was similar to the control (Figure ,). However, the primary root length of the mock control real was significantly larger than control Kurmi (p < 0.05). Shoot length of both cultivars was unaffected after 2 days of co-culture with T-22 or BOL-12QD (Figure )). Comparing the mock controls, the shoot length of Kurmi was larger than real (p < 0.05). After this time of co-cultivation, there was not a measurable effect on the growth of the plant (Figure ,), and quinoa seedlings were as large as the side of the square Petri dish, and thus close to reaching the Petri dish walls.
Figure 1. Short-term treatment of C. quinoa seedlings with T-22 and BOL-12 on square Petri dishes. (A) Representative images of at least 10 quinoa seedlings treated with T-22 or BOL-12 at 2 dpi. (B) Effect of T-22 and BOL-12 on primary root growth in C. quinoa seedlings at inoculation time (0 dpi; black bars; n = 10) and 2 dpi (gray bars; n = 24). (C) Effect of T-22 and BOL-12 on shoot growth in C. quinoa seedlings at 2 dpi (n = 15). Data shows means ± SE per treatment. Shoots were not measurable at time of inoculation (0 dpi) because they were still surrounding the seed coat.
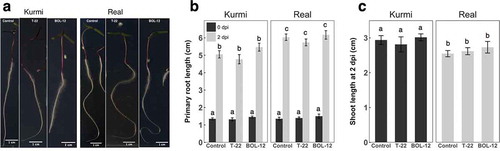
3.4. Long-term treatment of C. quinoa with T-22 and BOL-12QD in growth boxes
For investigating long-term effects, we co-cultivated 18-h-old quinoa seedlings with T-22 or BOL-12QD in growth boxes with slanted agar medium for 14 days. Shoot fresh weight was significantly reduced, similarly in cultivars Real and Kurmi (Figure )). In controls, the shoot fresh weight of real was similar to Kurmi. Root fresh weight was not quantified because lateral roots were stuck on the agar, thus detaching unevenly from the primary root when lifted.
Figure 2. Long-term treatment of C. quinoa seedlings with T-22 and BOL-12 in growth boxes. Effect of T-22 and BOL-12 in C. quinoa seedlings at 14 dpi on (A) shoot growth (n = 17) (B) chlorophyll content (n = 3) and (C) betalain content (n = 3). Data shows means ± SE per treatment. Statistically significant differences (p < 0.05) are denoted with different letters.
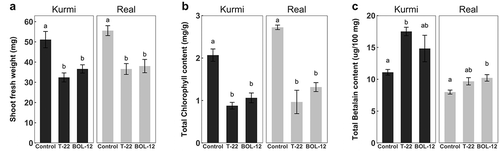
Functional differences between cultivars and treatments were investigated through pigment shoot contents. Chlorophyll content was measured as an indicator of general vitality and Caryophyllales-specific betalain pigments as a marker for general defense response activation level (Polturak & Aharoni, Citation2018). The chlorophyll concentrations of mock-treated control seedlings were similar in real and Kurmi (Figure )). However, chlorophyll concentration was significantly decreased in both cultivars upon interaction with either strain of Trichoderma. The betalain concentration of the mock-treated control seedlings was significantly larger (p < 0.05) in Kurmi than in real (Figure )). Upon interaction with T-22 and BOL-12, the betalain level increased in both cultivars, being significantly different in Kurmi seedlings treated with T-22 and in real seedlings treated with BOL-12. Under these growth conditions, quinoa seedlings had very long hypocotyls, indicating a possible restriction by light.
Growth inhibition in axenic co-culture of Arabidopsis thaliana with Trichoderma sp. has been reported to be due to acidification of the growth media (Pelagio-Flores, Esparza-Reynoso, Garnica-Vergara, López-Bucio, & Herrera-Estrella, Citation2017). However, the roots of 2-day-old quinoa seedlings acidified the medium to a pH below 3.5 in the absence of Trichoderma (Supplementary Figure 1). Trichoderma was found to acidify the medium during the first days of mycelial growth (2 dpi), whereas as conidiation started, the pH increased to above 6 (5 dpi; Supplementary Figure 1). This indicates that the Trichoderma inhibition of the quinoa growth was not due to a lowered pH.
3.5. Trichoderma interactions at a higher light intensity
We then investigated co-cultivation under higher light intensity (175 µmol m−2 s−1) in 45° angled square Petri dishes, which allows documentation of finer root and shoot structures without lifting seedlings from the agar surface. Quinoa seedlings grown under higher light intensity were almost as large as the sides of square Petri dishes (24 × 24 cm) after 6 days of incubation (Figure )) and had shorter hypocotyls than under regular light intensity (Figure )). Quinoa seedlings co-cultivated for 7 days under regular light intensity had already significantly longer hypocotyls than seedlings co-cultivated for 10 days under higher light intensity (Figure )). Real hypocotyls were significantly shorter when treated with BOL-12, and this was only observed under higher light intensity (Figure )).
Figure 3. Co-cultivation of T-22 and BOL-12 with C. quinoa seedlings under higher light intensity in square Petri dishes. (A) Representative images of at least 3 quinoa seedlings treated with T-22 or BOL-12 at 6 dpi. (B) Effect of higher light intensity (black bars) on hypocotyl growth in C. quinoa seedlings treated with T-22 or BOL-12 compared with regular light intensity (gray bars; n = 17). Effect of T-22 and BOL-12 in C. quinoa seedlings at 10 dpi on (C) shoot growth (n = 19), (D) lateral root number (n = 12) and (E) lateral root growth (n = 19). Data shows means ± SE per condition. Statistically significant differences (p < 0.05) are denoted with different letters.
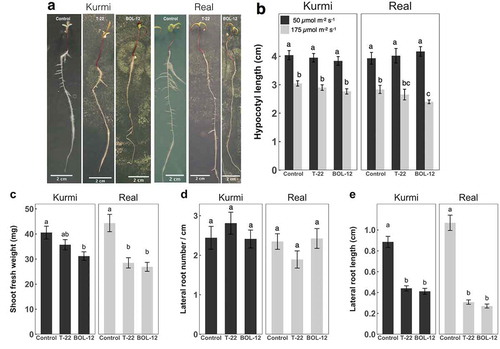
Quinoa seedlings grown at higher light showed also growth inhibition after treatment with T-22 or BOL-12 (Figure ), like observed under regular light intensity (Figure ). In cultivar real, shoot fresh weight was significantly decreased (by approximately 36%) when treated with T-22 or BOL-12, as compared to the mock control. Shoot fresh weight in Kurmi was less affected by treatment with T-22 and BOL-12, displaying a decrease of 12% and 23%, respectively, and only the latter being significant (Figure )). We observed changes in the quinoa root architecture after treatment with T. harzianum. The number of lateral roots per length unit of primary root was similar in plantlets treated with T-22 or BOL-12 and in the mock control. Also, for both mock-treated cultivars, the number of lateral roots was similar (Figure )). In contrast, extension of lateral roots (Figure )) was strongly and significantly inhibited by treatment with either strain of T. harzianum. The reduction by the two T. harzianum strains was stronger in real (71–75%) than in Kurmi (50–53%) (Figure ,). Lateral roots in both Kurmi and real seedlings treated with T-22 or BOL-12 had a stunted appearance (Figure ), and this phenomenon was accompanied by browning of the lateral roots (Figure ) and a decrease in lateral root hairs (not shown). Further, the primary root red color, especially in Kurmi, was decreased upon treatment with either strain of T. harzianum, indicating a loss of root betalain (Figure ).
Figure 4. Effects of T-22 and BOL-12 on quinoa lateral roots. The figure shows representative images among three C. quinoa seedling replicate sets, examined for lateral roots emergences after treatment with T-22 or BOL-12 for 10 days. The images were taken at 3 cm from the root neck. Arrowheads denote stunted lateral roots. The scale bars represent 500 µm.
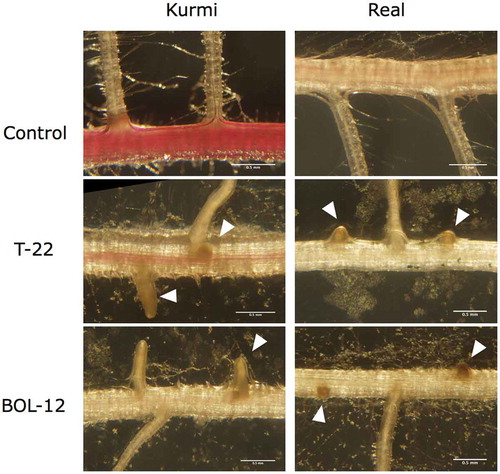
4. Discussion
Trichoderma species is the agriculturally most used microbial organism group for plant biocontrol and growth stimulation (Mukherjee, Citation2013). Growth stimulation by T. harzianum has been observed in many crop plants grown on soil (Harman, Taylor, & Stasz, Citation1989; Maag et al., Citation2013; Tucci et al., Citation2011; Yedidia, Srivastva, Kapulnik, & Chet, Citation2001), including also quinoa (Ortuño et al., Citation2013, Citation2016). However, the effect of Trichoderma on the growth outcome of plants has been found highly variable depending on fungal and plant genotypes as well as the growth conditions established (Harman, Citation2006; Tucci et al., Citation2011). For example, the commercially used biocontrol agent T-22 was found to inhibit the growth of certain cultivars of tomato (Tucci et al., Citation2011) and maize (Harman, Citation2006) in soil experiments. The large variation of outcomes by the plant-Trichoderma interactions is, however, difficult to investigate in soil systems because of the potential effects of other organisms, which cannot be controlled.
In axenic co-cultivation, we observed negative effects of two strains of T. harzianum on two cultivars of quinoa, affecting shoot growth as well as chlorophyll levels. The similar acidification of the medium by both quinoa and T. harzianum indicates that the inhibition of quinoa growth was not caused by Trichoderma acidification of the medium, which has been observed for A. thaliana (Pelagio-Flores et al., Citation2017). This is also consistent with the low pH observed for the germination of some quinoa cultivars (germination above 90% at pH 4.5) (González, Languasco, & Prado, Citation2014) and growth in a wide range of soils, ranging from acid soils with a pH of 4.5 to alkaline soils with a pH of 8.5 (Bhargava & Srivastava, Citation2013). In order to set a precise time for the beginning of the interaction in the axenic co-cultures, we inoculated pregerminated T. harzianum spores on top of the seedling root necks. In previous reports on sterile co-culture of plants and Trichoderma, interactions have generally been observed to promote plant growth. Examples include A. thaliana, tomato and tobacco seedlings in axenic co-cultures with Trichoderma virens Gv29.8, Trichoderma atroviride IMI 206040 and T. harzianum CECT 2413, respectively (Chacón, Rofríguez-Galán, & Al, Citation2007; Contreras-Cornejo, Macías-Rodríguez, Alfaro-Cuevas, & López-Bucio, Citation2014; Contreras-Cornejo, Macías-Rodríguez, Cortés-Penagos, & López-Bucio, Citation2009; Samolski, Rincón, Pinzón, Viterbo, & Monte, Citation2012). In these investigations, Trichoderma inoculum has been positioned a few centimeters away from the plant on the agar surface. Such physical separation yet indirect contact between Trichoderma and the plant allows for a period of time before physical interaction. During this period, the organisms can interchange molecular compounds and signals that may affect the outcome of the following physical interaction. For example, Trichoderma volatile organic compounds alone can strongly promote growth of A. thaliana, most likely by stimulating lateral root growth (Contreras-Cornejo, Macías-Rodríguez, Herrera-Estrella, & López-Bucio, Citation2014; Hung, Lee, & Bennett, Citation2013; Kottb, Gigolashvili, Großkinsky, & Piechulla, Citation2015). In addition, symbiotic mycorrhizal fungi start colonization only after chemical signaling, where plants secrete the appropriate signal into the soil and the fungi recognize and respond to it (Jones & Dangl, Citation2006; Oldroyd, Citation2013). Whether a similar preparatory signaling occurs between plant roots and Trichoderma is not known.
Overall, the two Trichoderma strains did not qualitatively differ regarding the effect on the growth of either of the two quinoa cultivars or vice versa. As described above, a small number of Trichoderma strains and plant genotypes that are non-compatible for growth promotion have been reported (Harman, Citation2006; Tucci et al., Citation2011). Though it cannot be excluded that the quinoa and T. harzianum genotypes tested were untypical incompatible variants, the qualitatively similar effects on the two plant cultivars indicate that the T. harzianum-induced growth inhibition may be typical for quinoa in the described axenic co-cultures. The largest differences observed between Kurmi and real on the response to the strains of Trichoderma were a somewhat stronger inhibitory effect on growth in Real (Figure ). This may be a consequence of real growing significantly faster than Kurmi (Figures and ). Trade-off between growth rate and stress resistance has been described for several plant species (Weih, Citation2003).
Consistently, a main differences between previously analyzed Trichoderma-plant interactions in axenic cultures and this T. harzianum-quinoa study is the low age of the quinoa seedlings upon treatment; 4–25 days in previous studies (Contreras-Cornejo, et al. 2014; Contreras-Cornejo et al., Citation2009; Saenz-Mata & Jimenez-Bremont, Citation2012; Sáenz-Mata, Salazar-Badillo, & Jiménez-Bremont, Citation2014; Samolski et al., Citation2012) versus 18 h in quinoa. The young age of treated quinoa was a necessity, due to the extremely high growth rate (approximately 4 cm/day) in axenic culture, also necessitating large growth vessels to be used. The young age and high rate of growth may have been contributing factors to the negative effect on quinoa by T. harzianum. In general, biotic stress resistance depends of plant age, the younger the plant the more susceptible it is. This correlation has been shown for several plant species against different pathogens, e.g. wheat against Puccinia striiformis (Farber & Mundt, Citation2017), broccoli against Peronospora parasitica (Dickson & Petzoldt, Citation1993) and Arabidopsis against Pseudomonas syringae (Carella, Wilson, & Cameron, Citation2015; Kus, Zaton, Sarkar, & Cameron, Citation2002; Panter & Jones, Citation2002).
In the axenic cultures of quinoa, lateral root growth was the parameter most strongly affected by Trichoderma treatment. Stunting of lateral root growth (Figure (a,e)) as well as browning of lateral root primordia and entire lateral roots was observed (Figure ). This indicates that quinoa is directly damaged by Trichoderma. Similar lateral root damage has been observed in Medicago truncatula roots infected by the phytopathogenic fungi Fusarium solani f. sp. phaseoli (Salzer et al., Citation2000). Consistently, the general changes in betalain content in quinoa upon T. harzianum treatment (Figure )) may be a sign of plant defense as previously seen in red beets infiltrated with P. syringae (Sepúlveda-Jiménez, Rueda-Benítez, Porta, & Rocha-Sosa, Citation2004) and betalain-producing transgenic tobacco against Botrytis cinerea (Polturak et al., Citation2017). Interestingly, Kurmi had a larger content of betalain than Real (Figures ) and ) and was less negatively affected by T. harzianum. Betalains are analogous to anthocyanins, which likewise are induced by biotic and abiotic stress (Khan & Giridhar, Citation2015). A representative anthocyanin, camalexin, is usually induced in response to phytopathogenic attacks (Lemarié et al., Citation2015; Mert-Türk, Bennett, Mansfield, & Holub, Citation2003). However, an increase in camalexin concentration has also been observed when A. thaliana growth was treated with Trichoderma spp., inducing growth enhancement (Contreras-Cornejo, Macías-Rodríguez, Beltrán-Peña, Herrera-Estrella, & López-Bucio, Citation2011; Salas-Marina et al., Citation2011). Changes in betalain in quinoa may have an analogous function.
In summary, the plant growth inhibition and lateral root stunting observed when co-culturing C. quinoa with biocontrol strains of T. harzianum under axenic conditions indicate that T. harzianum in particular growth regimes can cause harm to plants. Elucidating the mechanisms behind this damage may explain the exceptions from growth enhancement that have been observed for Trichoderma treatment of crop plants growing on soil.
Supplemental Material
Download PNG Image (366.4 KB)Acknowledgements
We are grateful to Proinpa Institute (Quipaquipani, Bolivia) for the generous donation of quinoa seeds and the Instituto de Investigaciones Farmaco-bioquímicas - UMSA (La Paz, Bolivia) for providing Trichoderma harzianum BOL-12QD.
Supplementary material
Supplemental material for this article can be accessed here.
Additional information
Funding
Notes on contributors
Oscar M. Rollano-Peñaloza
Oscar M. Rollano-Peñaloza is PhD student in Biology at Lund University, Sweden in cooperation with Universidad Mayor de San Andrés, Bolivia. His main research involves plant–microbe interactions using molecular and genomic approaches.
Susanne Widell
Susanne Widell is professor emerita in Plant Physiology at Lund University in Sweden. Her research is centered on plant membranes and the molecular mechanisms in the interaction between plants and benevolent fungi.
Patricia Mollinedo
Patricia Mollinedo is a full-time professor at Chemical Sciences Department at Universidad Mayor de San Andrés in La Paz, Bolivia. She is a specialist in Natural Products and Molecular Oxidative Stress. Her research work is focused on Molecular interaction and novel Biocontrol agents against phytopathogens in food crops.
Allan G. Rasmusson
Allan G. Rasmusson is professor of Plant Physiology at Lund University in Sweden. His research centers on molecular mechanisms in plant respiratory redox metabolism and plant-microbe interactions.
References
- Abramoff, M. D., Magalhães, P. J., & Ram, S. J. (2004). Image processing with ImageJ. Biophotonics International, 11(7), 36-42.
- Ahmad, J. S. B., & Ralph. (1987). Rhizosphere competence of Trichoderma Harzianum. Phytopathology, 77(2), 182–189. doi:10.1094/Phyto-77-182
- Arnon, D. I., Allen, M. B., & Whatley, F. R. (1954). Photosynthesis by isolated chloroplasts. Nature, 174(4426), 394–396.
- Bazile, D., Pulvento, C., Verniau, A., Al-Nusairi, M. S., Ba, D., Breidy, J., … Otambekova, M., et al. (2016). Worldwide evaluations of quinoa: Preliminary results from post international year of quinoa fao projects in nine countries. Frontiers in Plant Science, 7, 850. doi:10.3389/fpls.2016.00850
- Bhargava, A., & Srivastava, S. (2013). Crop production and management. In A. Bhargava & S. Srivastava (Eds.), Quinoa: Botany, production and uses (p. 91). Wallingford, UK: CABI.
- Carella, P., Wilson, D. C., & Cameron, R. K. (2015). Some things get better with age: Differences in salicylic acid accumulation and defense signaling in young and mature Arabidopsis. Plant Biotic Interactions, 5, 775.
- Castellar, R., Obón, J. M., Alacid, M., & Fernández-López, J. A. (2003). Color properties and stability of betacyanins from opuntia fruits. Journal of Agricultural and Food Chemistry, 51(9), 2772–2776. doi:10.1021/jf021045h
- Chacón, M., Rofríguez-Galán, O., & Al, E. (2007). Microscopic and transcriptome analyses of early colonization of tomato roots by Trichoderma Harzianum. International Microbiology, 10, 19–27.
- Chet, I., & Baker, R. (1981). Isolation and biocontrol potential of Trichoderma Hamatum from soil naturally suppressive to Rhizoctonia Solani. Phytopathology, 71, 286–290. doi:10.1094/Phyto-71-286
- Contreras-Cornejo, H. A., Macías-Rodríguez, L., Alfaro-Cuevas, R., & López-Bucio, J. (2014). Trichoderma Spp. Improve growth of Arabidopsis seedlings under salt stress through enhanced root development, osmolite production, and Na+elimination through root exudates. Molecular Plant-Microbe Interactions, 27(6), 503–514. doi:10.1094/MPMI-09-13-0265-R
- Contreras-Cornejo, H. A., Macías-Rodríguez, L., Beltrán-Peña, E., Herrera-Estrella, A., & López-Bucio, J. (2011). Trichoderma-induced plant immunity likely involves both hormonal- and camalexin-dependent mechanisms in Arabidopsis Thaliana and confers resistance against necrotrophic fungi Botrytis Cinerea. Plant Signaling & Behavior, 6(10), 1554–1563. doi:10.4161/psb.6.10.17443
- Contreras-Cornejo, H. A., Macías-Rodríguez, L., Cortés-Penagos, C., & López-Bucio, J. (2009). Trichoderma Virens, a plant beneficial fungus, enhances biomass production and promotes lateral root growth through an auxin-dependent mechanism in Arabidopsis. Plant Physiology, 149(3), 1579–1592. doi:10.1104/pp.108.130369
- Contreras-Cornejo, H. A., Macías-Rodríguez, L., del-Val, E., & Larsen, J. (2016). Ecological functions of Trichoderma Spp. And their secondary metabolites in the rhizosphere: Interactions with plants. FEMS Microbiology Ecology, 92(4), fiw036. doi:10.1093/femsec/fiw036
- Contreras-Cornejo, H. A., Macías-Rodríguez, L., Herrera-Estrella, A., & López-Bucio, J. (2014). The 4-phosphopantetheinyl transferase of Trichoderma Virens plays a role in plant protection against Botrytis Cinerea through volatile organic compound emission. Plant and Soil, 379(1–2), 261–274. doi:10.1007/s11104-014-2069-x
- Danielsen, S., & Ames, T. (2000). Mildew (Peronospora Farinosa) of Quinoa (Chenopodium Quinua Willd) in the andean region: Practical manual for the study of the disease and the pathogen (Vol. 32). Lima, Peru: International Potato Center.
- Danielsen, S., Bonifacio, A., & Ames, T. (2003). Diseases of quinoa (Chenopodium Quinoa). Food Reviews International, 19(1–2), 43–59. doi:10.1081/FRI-120018867
- Danielsen, S., & Munk, L. (2004). Evaluation of disease assessment methods in quinoa for their ability to predict yield loss caused by downy mildew. Crop Protection, 23(3), 219–228. doi:10.1016/j.cropro.2003.08.010
- Dickson, M. H., & Petzoldt, R. (1993). Plant age and isolate source affect expression of downy mildew resistance in broccoli. HortScience, 28(7), 730–731.
- Espinal Churata, C., Huanca, M., Terrazas Siles, E., & Giménez Turba, A. (2010). Evaluación De La Actividad Biocontroladora De Trichoderma inhamatum Cepa Bol 12 Qd, Frente a Botrytis fabae, Causante De La Mancha Chocolate En Cultivos De Haba (Vicia faba). BIOFARBO 18(1), 13-30.
- Farber, D. H., & Mundt, C. C. (2017). Effect of plant age and leaf position on susceptibility to wheat stripe rust. Phytopathology, 107(4), 412–417. doi:10.1094/PHYTO-07-16-0284-R
- Gandarillas, A., Saravia, R., Plata, G., Quispe, R., & Ortiz-Romero, R. (2015). Principle quinoa pests and diseases. In D. Bazile, D. Bertero, & C. Nieto (Eds.), State of the art report of quinoa in the world in 2013 (pp. 192–217). Rome: FAO & CIRAD.
- García-Espejo, C. N., Mamani-Mamani, M. M., Chávez-Lizárraga, G. A., & Álvarez-Aliaga, M. T. (2016). Evaluación De La Actividad Enzimática Del Trichoderma inhamatum (Bol-12 Qd) Como Posible Biocontrolador. Journal of the Selva Andina Research Society, 7(1), 20–32.
- González, J. A., Languasco, P., & Prado, F. E. (2014). Efecto De Las Vinazas Sobre La Germinación De Soja, Trigo Y Quinoa En Condiciones Controladas. Boletín de la Sociedad Argentina de Botánica, 49(4), 473–481.
- Hadar, Y., Harman, G. E., & Taylor, A. G. (1984). Evaluation of Trichoderma Koningii and T. Harzianum from New York soils for biological control of seed rot caused by Pythium Spp. Phytopathology, 74(1), 106–110. doi:10.1094/Phyto-74-106
- Harman, G. E. (2006). Overview of mechanisms and uses of Trichoderma Spp. Phytopathology, 96(2), 190–194. doi:10.1094/PHYTO-96-0190
- Harman, G. E. (2011). Trichoderma—not just for biocontrol anymore. Phytoparasitica, 39(2), 103–108. doi:10.1007/s12600-011-0151-y
- Harman, G. E., Howell, C. R., Viterbo, A., Chet, I., & Lorito, M. (2004). Trichoderma species — opportunistic, avirulent plant symbionts. Nature Reviews Microbiology, 2(1), 43–56. doi:10.1038/nrmicro797
- Harman, G. E., Taylor, A. G., & Stasz, T. E. (1989). Combining effective strains of trichoderma harzianum and solid matrix priming to improve biological seed treatments. Plant Disease, 73(8), 631–637. doi:10.1094/PD-73-0631
- Hung, R., Lee, S., & Bennett, J. W. (2013). Arabidopsis thaliana as a model system for testing the effect of trichoderma volatile organic compounds. Fungal Ecology, 6(1), 19–26. doi:10.1016/j.funeco.2012.09.005
- Jacobsen, S. E., Mujica, A., & Jensen, C. R. (2003). The resistance of quinoa (Chenopodium Quinoa Willd.) to adverse abiotic factors. Food Reviews International, 19(1–2), 99–109. doi:10.1081/FRI-120018872
- Jones, J. D. G., & Dangl, J. L. (2006). The plant immune system. Nature, 444(7117), 323–329. doi:10.1038/nature05286
- Khan, M. I., & Giridhar, P. (2015). Plant betalains: Chemistry and biochemistry. Phytochemistry, 117, 267–295. doi:10.1016/j.phytochem.2015.06.008
- Kottb, M., Gigolashvili, T., Großkinsky, D. K., & Piechulla, B. (2015). Trichoderma volatiles effecting Arabidopsis: From inhibition to protection against phytopathogenic fungi. Frontiers in Microbiology, 6. doi:10.3389/fmicb.2015.00995
- Kubicek, C. P., Herrera-Estrella, A., Seidl-Seiboth, V., Martinez, D. A., Druzhinina, I. S., Thon, M., … Grigoriev, I. V. (2011). Comparative genome sequence analysis underscores mycoparasitism as the ancestral life style of trichoderma. Genome Biology, 12(4), R40. doi:10.1186/gb-2011-12-10-r102
- Kujala, T., Loponen, J., & Pihlaja, K. (2001). Betalains and phenolics in red beetroot (Beta Vulgaris) peel extracts: Extraction and characterisation. Zeitschrift für Naturforschung C, 56(5–6), 343–348. doi:10.1515/znc-2001-5-604
- Kus, J. V., Zaton, K., Sarkar, R., & Cameron, R. K. (2002). Age-related resistance in Arabidopsis is a developmentally regulated defense response to pseudomonas syringae. The Plant Cell Online, 14(2), 479–490. doi:10.1105/tpc.010481
- Lemarié, S., Robert-Seilaniantz, A., Lariagon, C., Lemoine, J., Marnet, N., Levrel, A., … Gravot, A. (2015). Camalexin contributes to the partial resistance of arabidopsis thaliana to the biotrophic soilborne protist Plasmodiophora Brassicae. Frontiers in Plant Science, 6. doi:10.3389/fpls.2015.00539
- Maag, D., Kandula, D. R. W., Müller, C., Mendoza-Mendoza, A., Wratten, S. D., Stewart, A., & Rostás, M. (2013). Trichoderma Atroviride Lu132 promotes plant growth but not induced systemic resistance to Plutella Xylostella in oilseed rape. BioControl, 59(2), 241–252. doi:10.1007/s10526-013-9554-7
- Mert-Türk, F., Bennett, M. H., Mansfield, J. W., & Holub, E. B. (2003). Camalexin accumulation in Arabidopsis Thaliana following abiotic elicitation or inoculation with virulent or avirulent Hyaloperonospora Parasitica. Physiological and Molecular Plant Pathology, 62(3), 137–145. doi:10.1016/S0885-5765(03)00047-X
- Mukherjee, P. K. (2013). Trichoderma: Biology and applications. Boston, MA: CABI.
- Nagaraju, A., Sudisha, J., Murthy, S. M., & Ito, S.-I. (2012). Seed priming with Trichoderma Harzianum isolates enhances plant growth and induces resistance against Plasmopara Halstedii, an incitant of sunflower downy mildew disease. Australasian Plant Pathology, 41(6), 609–620. doi:10.1007/s13313-012-0165-z
- Nandini, B., Hariprasad, P., Niranjana, S. R., Shetty, H. S., & Geetha, N. P. (2013). Elicitation of resistance in pearl millet by oligosaccharides of trichoderma spp. against downy mildew disease. Journal of Plant Interactions, 8(1), 45–55. doi:10.1080/17429145.2012.710655
- Ni, Z., Kim, E.-D., & Chen, Z. J. (2009). Chlorophyll and starch assays. Protocol Exchange, 1. doi:10.1038/nprot.2009.12
- Oldroyd, G. E. D. (2013). Speak, friend, and enter: signalling systems that promote beneficial symbiotic associations in plants. Nature Reviews Microbiology, 11(4), 252–263. doi:10.1038/nrmicro2990
- Ortuño, N., Castillo, J., Claros, M., Navia, O., Angulo, M., Barja, D., … Angulo, V. (2013). Enhancing the sustainability of quinoa production and soil resilience by using bioproducts made with native microorganisms. Agronomy, 3(4), 732–746. doi:10.3390/agronomy3040732
- Ortuño, N., Castillo, J. A., Miranda, C., Claros, M., & Soto, X. (2016). The use of secondary metabolites extracted from trichoderma for plant growth promotion in the Andean highlands. Renewable Agriculture and Food Systems, 32(4), 366-375.
- Panter, S. N., & Jones, D. A. (2002). Age-related resistance to plant pathogens. In J. A. Callow (Ed.), Advances in botanical research (pp. 251–280). Cambridge, MA: Academic Press.
- Pelagio-Flores, R., Esparza-Reynoso, S., Garnica-Vergara, A., López-Bucio, J., & Herrera-Estrella, A. (2017). Trichoderma-induced acidification is an early trigger for changes in Arabidopsis root growth and determines fungal phytostimulation. Frontiers in Plant Science, 8. doi:10.3389/fpls.2017.00822
- Perazzolli, M., Moretto, M., Fontana, P., Ferrarini, A., Velasco, R., Moser, C., … Pertot, I. (2012). Downy mildew resistance induced by Trichoderma Harzianum T39 in susceptible grapevines partially mimics transcriptional changes of resistant genotypes. BMC Genomics, 13(1), 660. doi:10.1186/1471-2164-13-660
- Polturak, G., & Aharoni, A. (2018). “La Vie En Rose”: Biosynthesis, sources, and applications of betalain pigments. Molecular Plant, 11(1), 7–22. doi:10.1016/j.molp.2017.10.008
- Polturak, G., Grossman, N., Vela-Corcia, D., Dong, Y., Nudel, A., Pliner, M., … Aharoni, A. (2017). Engineered gray mold resistance, antioxidant capacity, and pigmentation in betalain-producing crops and ornamentals. Proc Natl Acad Sci U S A, 114(34), 9062–9067. doi:10.1073/pnas.1707176114
- R Core Team. (2016). R: A language and environment for statistical computing. Vienna: R Foundation for Statistical Computing.
- Ruiz, K. B., Biondi, S., Oses, R., Acuña-Rodríguez, I. S., Antognoni, F., Martinez-Mosqueira, E. A., … Zurita-Silva, A., et al. (2014). Quinoa biodiversity and sustainability for food security under climate change. A review. Agronomy for Sustainable Development, 34(2), 349–359. doi:10.1007/s13593-013-0195-0
- Saenz-Mata, J., & Jimenez-Bremont, J. F. (2012). Hr4 gene is induced in the arabidopsis-trichoderma atroviride beneficial interaction. International Journal of Molecular Sciences, 13(7), 9110–9128. doi:10.3390/ijms13079110
- Sáenz-Mata, J., Salazar-Badillo, F. B., & Jiménez-Bremont, J. F. (2014). Transcriptional regulation of Arabidopsis Thaliana Wrky genes under interaction with beneficial fungus Trichoderma Atroviride. Acta Physiologiae Plantarum, 36(5), 1085–1093. doi:10.1007/s11738-013-1483-7
- Salas-Marina, M. A., Silva-Flores, M. A., Uresti-Rivera, E. E., Castro-Longoria, E., Herrera-Estrella, A., & Casas-Flores, S. (2011). Colonization of Arabidopsis roots by Trichoderma Atroviride promotes growth and enhances systemic disease resistance through jasmonic acid/ethylene and salicylic acid pathways. European Journal of Plant Pathology, 131(1), 15–26. doi:10.1007/s10658-011-9782-6
- Salzer, P., Bonanomi, A., Beyer, K., Vögeli-Lange, R., Aeschbacher, R. A., Lange, J., … Boller, T. (2000). Differential expression of eight chitinase genes in Medicago Truncatula Roots during mycorrhiza formation, nodulation, and pathogen infection. Molecular Plant-Microbe Interactions, 13(7), 763–777. doi:10.1094/MPMI.2000.13.7.763
- Samolski, I., Rincón, A. M., Pinzón, L. M., Viterbo, A., & Monte, E. (2012). The Qid74 gene from Trichoderma Harzianum has a role in root architecture and plant biofertilization. Microbiology, 158(1), 129–138. doi:10.1099/mic.0.053140-0
- Schuster, A., & Schmoll, M. (2010). Biology and biotechnology of Trichoderma. Applied Microbiology and Biotechnology, 87(3), 787–799. doi:10.1007/s00253-010-2632-1
- Sepúlveda-Jiménez, G., Rueda-Benítez, P., Porta, H., & Rocha-Sosa, M. (2004). Betacyanin Synthesis in red beet (Beta Vulgaris) leaves induced by wounding and bacterial infiltration is preceded by an oxidative burst. Physiological and Molecular Plant Pathology, 64(3), 125–133. doi:10.1016/j.pmpp.2004.08.003
- Stasz, T. E., Harman, G. E., & Weeden, N. F. (1988). Protoplast preparation and fusion in two biocontrol strains of Trichoderma Harzianum. Mycologia, 141–150. doi:10.1080/00275514.1988.12025515
- Testen, A. L., Del Mar Jiménez-Gasco, M., Ochoa, J. B., & Backman, P. A. (2014). Molecular detection of Peronospora Variabilis in quinoa seed and phylogeny of the quinoa downy mildew pathogen in South America and the United States. Phytopathology, 104(4), 379–386. doi:10.1094/PHYTO-07-13-0198-R
- Tucci, M., Ruocco, M., De Masi, L., De Palma, M., & Lorito, M. (2011). The beneficial effect of Trichoderma spp. On tomato is modulated by the plant genotype. Molecular Plant Pathology, 12(4), 341–354. doi:10.1111/j.1364-3703.2010.00674.x
- Vega-Gálvez, A., Miranda, M., Vergara, J., Uribe, E., Puente, L., & Martínez, E. A. (2010). Nutrition facts and functional potential of quinoa (Chenopodium Quinoa Willd.), an Ancient Andean grain: A review. Journal of the Science of Food and Agriculture, 90(15), 2541–2547. doi:10.1002/jsfa.4158
- Weih, M. (2003). Trade-offs in plants and the prospects for breeding using modern biotechnology. New Phytologist, 158(1), 7–9. doi:10.1046/j.1469-8137.2003.00716.x
- White, T. J., Bruns, T., Lee, S., & Taylor, J. W. (1990). Amplification and direct sequencing of fungal ribosomal rna genes for phylogenetics. PCR Protocols: a Guide to Methods and Applications, 18(1), 315–322.
- Wickham, H. (2011). The split-apply-combine strategy for data analysis. Journal of Statistical Software, 40, 1. doi:10.18637/jss.v040.i01
- Wickham, H. (2016). ggplot2: Elegant graphics for data analysis. New York, NY: Springer-Verlag.
- Yedidia, I., Benhamou, N., & Chet, I. (1999). Induction of defense responses in cucumber plants (Cucumis Sativus L.) by the biocontrol agent Trichoderma Harzianum. Applied and Environmental Microbiology, 65(3), 1061–1070.
- Yedidia, I., Srivastva, A. K., Kapulnik, Y., & Chet, I. (2001). Effect of Trichoderma Harzianum on microelement concentrations and increased growth of cucumber plants. Plant and Soil, 235(2), 235–242. doi:10.1023/A:1011990013955