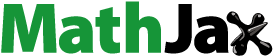
Abstract
This paper presents the result of an experimental study on the shear behaviour of a pre-sheared clayey soil. The effect of shear rate on the residual strength of pre-sheared clays was investigated in a ring shear apparatus. A pre-sheared surface was initially developed at a slow rate of 0.1 mm/min. Fast shear rates were then applied to the pre-sheared specimens to investigate the impact of the shear rates on the results. The laboratory results show that there is an immediate tendency for the residual strength to increase with increasing shear rate. Following this, the fast residual strength continues to increase with further displacement, reaching the peak value of fast residual strength (i.e. positive rate effect). Finally, the fast residual strength drops with increasing displacement to a value less than slow residual strength (i.e. negative rate effect). Overall, the relationship between the residual strength and the fast shear rates indicates a positive and negative rate effect based on the shear rates and the shear displacement.
Public Interest Statement
This paper presents the result of an experimental study on the shear behaviour of a pre-sheared clayey soil. The residual shear strength is the centre of focus of many researchers for slope stability analyses of old landfills where a slip surface exists. A residual shear strength of clayey soil is defined as the minimum shear strength of the soil along an existing shear surface. The dependency of residual shear strength and shear rate was investigated in this paper using a ring shear apparatus. Based on the experimental results, there is an increase in residual shear strength in comparison with the slow residual shear strength when the shear displacement rates increase.
1. Introduction
A residual shear strength of clayey soil under a prescribed normal stress is defined as the minimum shear strength of the soil under relatively large shear displacement (ASTM D647) (American Society for Testing and Materials [ASTM], Citation2015). The displacement required to develop the residual strength is greater than the displacements corresponding to the peak shear strength (maximum shear strength) and the critical state shear strength (fully softened) in over consolidated soils. Accordingly, the residual shear strength is not related to the first slide of slopes but related to the existence of slip surface such as old landslides (Skempton, Citation1985). The orientation of clay particles parallel to the shear direction results in post peak drop of undrained shear strength and the shear strength reaches to the critical state value at the end of the first step (smaller shear displacement). When the particle reorientation completes at larger displacement, the shear strength reaches the residual value and remains constant with the increase in displacement (Skempton, Citation1985).
Moreover, the residual shear strength of the clayey soil is the shear strength along a pre-developed shear zone. In fact, this pre-existing movement can be developed by various factors, such as an old landslide, tectonic forces, a change in groundwater, earthquake forces, blasting and dynamic/seismic loadings.
The residual shear strength of cohesive soils has been the centre of focus for many researchers all over the world for the past few decades to predict the behaviour of landslides (Gratchev & Sassa, Citation2015; Li, Wen, Aydin, & Ju, Citation2013; Tika & Hutchinson, Citation1999; Tika, Vaughan, & Lemos, Citation1996; Vulliet & Hutter, Citation1988). It is anticipated that the soil skeleton, clay type, particle size distribution, displacement rate and pore water have considerable effect on the residual shear strength of cohesive soils (Gratchev & Sassa, Citation2015; Mesri & Olson, Citation1970).
Tika et al. (Citation1996) performed a series of experimental tests on natural clays to investigate the effect of fast shearing on their shear behaviour. Their findings indicate that the shear strength of the soils studied increases when the shear rates are high. However, the shear strength of the fast rate test drops to a residual of shear strength, which may be greater, equal or even less than the residual shear strength at a slow rate. Tika et al. (Citation1996) also proposed three modes of failure (i.e. sliding, transitional and turbulent failure) to explain the dissimilar tendency observed for the shear rate effect.
Tika and Hutchinson (Citation1999) carried out several ring shear tests on two natural samples collected from a slip surface failure in Italy. Their laboratory results show that there is a negative correlation between the residual stress and the shear displacement rate for the samples studied. In fact, the residual shear strength at a fast rate of displacement was assessed and found to be up to 60% less than that performed at a slow rate.
Li et al. (Citation2013) carried out 27 large ring shear tests on the specimens collected from the slip surfaces of three existing landslides. Three different shear rates were employed in their investigation to study the effect of fast rate on the results (i.e. 0.1, 1 and 10 mm/s). According to Li et al. (Citation2013), the Atterberg limits and the particle size distribution, as well as the gravel and the fine fraction are additional factors that affect the residual shear strength. It was also found that the residual shear strength for the natural soils studied has a negative correlation with the plasticity index and the surface smoothness. This means that the residual strength decreases with an increase in either of these parameters. On the other hand, the particle symmetry defined by the elongation of the soil particles has a positive effect on the residual strength of the soil studied.
Gratchev and Sassa (Citation2015) studied the shear strength of three different clays at different rates of shear displacement. It was concluded that the displacement rate had a considerable impact on all three clays studied. Based on their findings, the shear strength increases slightly when the shear rate decreases. It was also discovered that the increase of the shear strength depends on the confining pressure. Additionally, the increase in the shear strength is significant for confining pressures greater than 100 kPa.
Scaringi and Di Maio (Citation2016) performed a series of controlled, direct shear tests on several mixtures of bentonite, kaolin and sand. In their study, the shear rate varied between 0.0001 and 100 mm/min. It was concluded that there is a positive correlation between the shear displacement rate and the residual strength of clayey soils when the clay fraction is higher than 50% and the displacement rate is greater than 1 mm/min. However, the displacement rate has no effect on the results for rates ranging from 0.0001 to 1 mm/min. Ring shear tests with various rates were also performed on a mixture of the soils and sodium chloride solution to study the effect of salt concentration on the results. The results revealed that the salt concentration has a positive rate effect. In other words, the rate impact increases with an increasing salt concentration. It was also established that this increase can be explained well by the decrease in the void ratio and the increase in the solid particle contacts due to the solution concentration.
Most studies on the shear-rate effect show that the excess pore-water pressure developed at the shearing stage affects the undrained shear strength of clayey soils (Casacrande & Wilson, Citation1951; Gratchev & Sassa, Citation2015; Mesri & Olson, Citation1970; Richardson & Whitman, Citation1963). Casacrande and Wilson (Citation1951) proposed that the pore-water pressure generated in the sample at the low shear rate is higher than at higher shear rate. Accordingly, a change in the generated pore-water pressure during the shearing stage results in the dependency of undrained shear strength (Casacrande & Wilson, Citation1951; Richardson & Whitman, Citation1963).
However, many studies have been carried out on the residual shear strength of cohesive soils, and some inconsistent or even opposite results have been reported in the literature (Gratchev & Sassa, Citation2015; Scaringi & Di Maio, Citation2016), which indicates that there is still a lack of experimental data on this topic. Correspondingly, more laboratory tests are required to ascertain the shear behaviour of clayey soils at large shear displacements. In this study, the residual shear strength of an expansive clay was measured after pre-shearing at various shear rates and normal stresses to investigate the effect of these factors on the shear behaviour of the soil studied.
2. Materials and test procedure
A clayey soil collected from the south of Perth, Western Australia was used in this study. This clay referred herein as “black clay”, is a highly expansive clay containing 68% clay, 20% sand and 12% silt. The clay had a high value liquid limit (wL) of 82, and a plasticity index (IP) of 47 (Habibbeygi, Nikraz, & Chegenizadeh, Citation2017; Habibbeygi, Nikraz, & Verheyde, Citation2017). Analyses of the mineralogy of the samples indicate that the predominant clay mineral is smectite. The results of one-dimensional consolidation tests illustrate a high potential of expansion and compressibility for the black clay (Habibbeygi et al., Citation2017). The in situ water content of the black clay was measured shortly after sample collection, and then assessed and found to be approximately 40% (Habibbeygi et al., Citation2017).
The ring shear device used in this study is shown in Figure . This device can provide predefined torsional shear on an annular specimen under a fully saturated condition to measure the residual shear strength of the specimen. The specimen is placed vertically between two porous stones. A counter balance (10:1 ratio lever) loading system is employed to apply the required pressure. A variable speed motor controlled by computer applies the rotation to the lower platen. A pair of load cells automatically measure the torque transmitted to the specimen. Both load cells and linear transducer are connected to a data acquisition system to record and process data. The shear strength of the specimen and the vertical load can be calculated for the predefined normal stress and shear rate using Equations (1) and (2):(1)
(1)
(2)
(2)
where τ is the shear stress (MPa), F1, F2 are the shear forces being measured by load cells (N), R1, R2 are the inner radius and outer radius of the specimens respectively (mm), L is the length of the torque arm (mm), P is the vertical load being applied to the specimens (N), and is the normal stress being applied vertically to the specimens (MPa).
First, the specimens were prepared following Head’s procedure for preparing a remoulded sample (Head, Citation1986). Remoulded specimens were prepared by crushing an air-dried sample using a rubber pestle and mortar. The predefined amount of water was then added to the oven-dried soil to achieve a water content equal to the in situ water content. All samples were kneaded on a glass plate in order to be mixed uniformly and were stored in a three-layer air-tight bag for one day to mature prior to torsional shear tests. Finally, the specimens were placed into an annular mould using a spatula. The specimen filling was carried out in small horizontal layers to avoid any voids in the cell. The top of the specimen was flattened with the specimen container. They were then kept for 24 h in the container fully filled with water to be saturated before applying the normal stress increments (Figure ). The container was kept full during the consolidation stage.
Secondly, the specimens were consolidated under the stress increments to achieve the final desired normal stresses of 50, 100, and 200 kPa. Prior to proceeding to the pre-shear step, the normal stress increment at each stage of consolidation and the completion of each increment was verified following the test method, ASTM D2435. The vertical load required to be applied to the annular specimen was calculated using Equation (1).
Following this, the specimens were pre-sheared to develop a shear surface. The specimens were sheared slowly at the rate of 0.1 mm/min for one day. A slow rate of shear displacement was selected to prevent any sample extrusion or to generate any pore water pressure during this step. To investigate the effect of shear rate on the shear behaviour of the pre-sheared specimens, various shear rates were applied to the specimens (i.e. 200, 100, 50, 10, 1 mm/min). The test procedure used in this study is schematically presented in Figure . According to this figure, the pre-sheared specimens were sheared subsequently at five faster shear rates. The shear rates begin with a high value of 200 mm/min and eventually decrease to a relatively slow rate of 1 mm/min. The shear strength of the specimens at each step was then calculated for the predefined normal stress and shear rate of that step using Equation (2).
3. Results and discussion
The ring shear tests under controlled shear displacement were performed on the black clay specimens consolidated at various normal stresses (i.e. 50, 100, 200 kPa). The specimens were sheared initially at a slow rate of 0.1 mm/min to develop a predefined shear surface. The shear rate was retained at a slow rate to prevent any sample extrusion, and to develop pore water pressure (step 1). In accordance with ASTM D6467, at least one revolution of the ring was employed to create a pre-sheared surface. At this stage, the shear strength neither increased nor decreased when the shear displacement increased. Therefore, it can be concluded that an existing sheared surface was present before conducting fast shearing stages. The experimental tests show that the residual shear strength of the clayey soil studied is 14.9, 45.3 and 51.1 kPa for the applied normal stresses of 50, 100 and 200 kPa, respectively. Figure illustrates the results of the shear behaviour for the fast shear steps for different normal stresses (i.e. steps 2 to 6). As illustrated, the fast residual strength increases the slow residual strength at all fast shear rates after a relatively small shear displacement. For example, the increase in percentage relative to the slow residual strength is approximately between 5 and 10% for various shear rates at the normal stress of 200 kPa. This increase in comparison with the slow residual strength occurs at the maximum shear rate (i.e. 200 mm/min) and step 4 (i.e. 50 mm/min). The increase to the slow residual strength decreases with a decreasing shear rate, from 50 mm/min to the minimum shear rate of 1 mm/min. However, the fast residual strengths are greater than the slow residual strengths under all normal stresses and at the shear rates.
Figure 4. Residual shear strength vs. time at various shear rates. (a) = 50 kPa, (b)
= 100 kPa and (c)
=200 kPa.
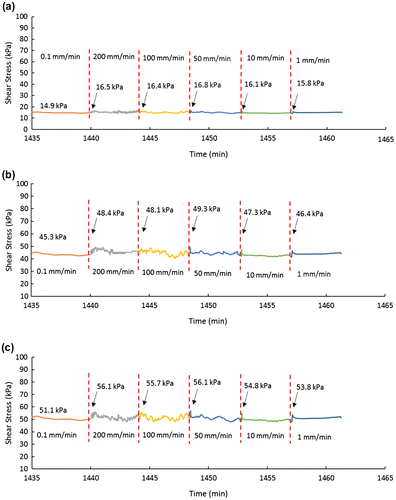
The fast residual strengths of the clay studied vs. time were replotted in Figure to illustrate the effect of the applied normal stress on the results. As expected, the residual strength increases with increasing applied normal strength. In fact, under the normal stress of 200 kPa, the slow residual strength of the soil studied is equal to 51.1 kPa, which is 342 and 112% of those under the normal stresses of 50 and 100 kPa, respectively. Similarly, the same trend is nearly identical for the peak residual strength at faster rates. The fast residual strength under 100 kPa is nearly 10% higher than that under 50 kPa. Furthermore, the fast residual strength increases to three times the relative strength when the normal stress increases to 200 kPa.
Figure presents the relationship between the fast residual strengths and time for various shear rates under the normal stress of 200 kPa. As illustrated, the fast residual strength increases immediately to a value greater than the slow residual stress (i.e. 54.1 kPa in comparison with 51.1 kPa) when the shear rate increases. The fast residual strength at the rate of 200 mm/min then continues to increase with time and reaches the peak fast residual strength (56.1 kPa). Tatsuoka, Di Benedetto, Enomoto, Kawabe, and Kongkitkul (Citation2008) called this positive impact of fast shear rates on the residual strength of the clayey soils, “Isotach behaviour”. This positive effect is likely followed by a negative effect with a further displacement. In fact, the fast residual strength decreases from 51.1 to 47.9 kPa (i.e. 6% drop). Such an increase and decrease in the residual strength was also observed by other researchers for clayey soils (Tika et al., Citation1996). Likewise, such similar behaviour is also observed in this study for other rates of shear displacement. However, for the last two steps (steps 5 and 6), there is a sharp increase in the fast residual strength when the rate changes, which is followed by a drop in the fast residual strength. The positive and negative effect of shear rate is virtually the same for other normal stresses. However, only the results of the normal stress of 200 kPa are presented herein for the sake of brevity and simplicity.
Figure 6. Residual shear behaviour under = 200 kPa at the various shear rates of (a) 200 mm/min, (b) 100 mm/min, (c) 50 mm/min, (d) 10 mm/min and (e) 1 mm/min.
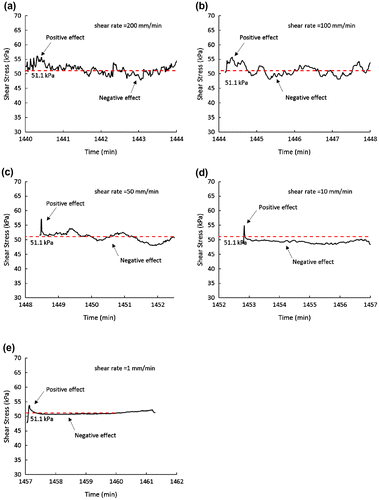
Overall, when a pre-sheared surface developed at residual shear strength by slow shearing was subjected to more rapid shear displacement rate, the Isotach behaviour was observed. The observed behaviour includes an increase in shear strength (positive effect) at a negligibly small displacement followed by a drop reaches the minimum value (negative effect). The studies on the mechanism of the negative effect suggest that the main reason for the observed loss of strength is the penetration of water along the shear surface. Fast shearing results in an increase in the void ratio and thus the water content. The water is then free to penetrate along the shear surface and reduces the shear strength (Tika et al., Citation1996). On the other hand, the increase in the shear strength at small displacement may be related to volume and structural changes within the shear surface during the fast shearing stage. Fast shearing can cause disordering the structure of the shear surface and result in an increase in the strength (Figure ).
4. Conclusion
The shear behaviour of a pre-sheared clay was investigated in this study to examine the effect of shear rate on residual shear strength. Based on the results of the ring shear tests, the following has been concluded:
• | There is an immediate increase of residual strength in comparison with the slow residual strength when the shear rates increase. In fact, there is a positive rate effect on the residual strength at a small displacement. | ||||
• | The fast residual strength of pre-sheared clays increases with an increasing applied normal stress. | ||||
• | The peak residual strengths of the pre-sheared clay in the fast shear steps are dependent on the rates of shear displacement. The fast residual strengths increase the slow residual strength when the rates increase. Moreover, the maximum peak residual strength of the fast steps occurs at the displacement rates of 50 and 200 mm/min. | ||||
• | There is a drop in the fast residual strength on top of the increase in the displacement for all various shear rates and the applied normal stresses. | ||||
• | There is a positive and negative effect of displacement rate on the residual strength for the rates greater than 50 kPa, which requires more research on the shear behaviour of pre-sheared clayey soils at fast shearing conditions. |
Funding
This work was supported by the Australian Government Research Training Program.
Additional information
Notes on contributors
Farzad Habibbeygi
Farzad Habibbeygi is a PhD student at Department of Civil Engineering, Faculty of Science and Engineering, Curtin University and a member of Engineers of Australia. His research area of interest is the mechanical and compression behaviour of expansive clays, numerical modelling and artificial neural network. He has also published several research papers in international journals in his area of interest.
References
- American Society for Testing and Materials (2015). Specifications. West Conshohocken, PA: Author.
- Casacrande, A., & Wilson, S. (1951). Effect of rate of loading on the strength of clays and shales at constant water content. Géotechnique, 2(3), 251–263.10.1680/geot.1951.2.3.251
- Gratchev, I. B., & Sassa, K. (2015). Shear strength of clay at different shear rates. Journal of Geotechnical and Geoenvironmental Engineering, 141(5), 06015002. doi:10.1061/(asce)gt.1943-5606.0001297
- Habibbeygi, F., Nikraz, H., & Chegenizadeh, A. (2017). Intrinsic compression characteristics of an expansive clay from Western Australia. International Journal of GEOMATE, 12(29), 140–147. doi:10.21660/2017.29.20455
- Habibbeygi, F., Nikraz, H., & Verheyde, F. (2017). Determination of the compression index of reconstituted clays using intrinsic concept and normalized void ratio. International Journal of Geomate, 13(39), 54–60. doi:10.21660/2017.39.98271
- Head, K. H. (1986). Manual of soil laboratory testing (Vol. 2). London: Pentech Press.
- Li, Y. R., Wen, B. P., Aydin, A., & Ju, N. P. (2013). Ring shear tests on slip zone soils of three giant landslides in the Three Gorges Project area. Engineering Geology, 154, 106–115. doi:10.1016/j.enggeo.2012.12.015
- Mesri, G., & Olson, R. (1970). Shear strength of montmorillonite. Géotechnique, 20(3), 261–270.10.1680/geot.1970.20.3.261
- Richardson, A. M., & Whitman, R. V. (1963). Effect of strain-rate upon undrained shear resistance of a saturated remoulded fat clay. Géotechnique, 13(4), 310–324.10.1680/geot.1963.13.4.310
- Scaringi, G., & Di Maio, C. (2016). Influence of displacement rate on residual shear strength of clays. Procedia Earth and Planetary Science, 16, 137–145. doi:10.1016/j.proeps.2016.10.015
- Skempton, A. W. (1985). Residual strength of clays in landslides, folded strata and the laboratory. Géotechnique, 35(1), 3–18.10.1680/geot.1985.35.1.3
- Tatsuoka, F., Di Benedetto, H., Enomoto, T., Kawabe, S., & Kongkitkul, W. (2008). Various viscosity types of geomaterials in shear and their mathematical expression. Soils and Foundations, 48(1), 41–60.10.3208/sandf.48.41
- Tika, T. E., & Hutchinson, J. (1999). Ring shear tests on soil from the Vaiont landslide slip surface. Géotechnique, 49(1), 59–74.10.1680/geot.1999.49.1.59
- Tika, T. E., Vaughan, P., & Lemos, L. (1996). Fast shearing of pre-existing shear zones in soil. Géotechnique, 46(2), 197–233.10.1680/geot.1996.46.2.197
- Vulliet, L., & Hutter, K. (1988). Viscous-type sliding laws for landslides. Canadian Geotechnical Journal, 25(3), 467–477.10.1139/t88-052