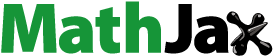
ABSTRACT
Recurring hot head-out water immersion (HOWI) enhances peripheral vascular function and cerebral blood velocity during non-immersion conditions. However, it is unknown if an acute bout of hot HOWI alters cerebrovascular function. Using two experimental studies, we tested the hypotheses that dynamic cerebral autoregulation (dCA) and cerebrovascular reactivity (CVR) are improved during an acute bout of hot (HOT; 39 °C) vs. thermoneutral (TN; 35 °C) HOWI. Eighteen healthy participants (eight females) completed the dCA study, and 14 participants (6 females) completed the CVR study. Both studies consisted of two randomized (TNdCA vs. HOTdCA; TNCVR vs. HOTCVR) 45minute HOWI visits. Middle cerebral artery blood velocity (MCAvmean) was continuously recorded. dCA was assessed using a respiratory impedance device and analyzed via transfer gain and phase in the low-frequency band. CVR was assessed using stepped hypercapnia. Assessments were completed PRE and 30 minutes into HOWI. Values are reported as a change (Δ) from PRE (mean ± SD). There were no differences at PRE for either study. ΔMCAvmean was greater in TNdCA (TNdCA: 4 ± 4 vs. HOTdCA: -3 ± 5 cm/s; P < 0.01) and TNCVR (TNCVR: 5 ± 4 vs. HOTCVR: -1 ± 6 cm/s; P < 0.01) during HOWI. ΔGain was greater in HOTdCA during HOWI (TNdCA: -0.09 ± 0.15 vs. HOTdCA: 0.10 ± 0.17 cm/s/mmHg; P = 0.04). ΔPhase (P > 0.84) and ΔCVR (P > 0.94) were not different between conditions. These data indicate that hot and thermoneutral water immersion do not acutely alter cerebrovascular function in healthy, young adults.
Introduction
Reductions in regional and/or global cerebral blood flow (CBF), which occur during aging [Citation1,Citation2], are associated with cognitive decline [Citation3–5] and an increased risk of dementia [Citation2,Citation6–9]. Reductions in CBF [Citation10–14] and cerebrovascular function [Citation15–18] also occur following head trauma, which likely impacts the duration of symptom resolution. Acute and chronic traumatic brain injuries impair cerebral autoregulation [Citation16–18] and cerebrovascular reactivity [Citation15,Citation19,Citation20]. Therefore, identifying effective interventions that preserve or improve CBF and cerebrovascular function is important. Repetitive increases in CBF and shear stress, such as what occurs during aerobic exercise, augment endothelial-derived nitric oxide bioavailability[Citation21], which is beneficial for cerebrovascular health [Citation22,Citation23]. However, individuals with exercise limitations (e.g., orthopedic injuries, older adults, Parkinson’s disease, etc.) may not be able to safely engage in aerobic exercise training. Thus, these populations are likely at a higher risk for reductions in resting CBF that could alter the ability of the cerebral circulation to buffer changes in blood pressure (i.e., cerebral autoregulation) and arterial CO2 (i.e., cerebrovascular reactivity). Therefore, it is important to identify alternative methods that augment CBF, cerebrovascular shear stress, and cerebrovascular function.
Recently, frequent sauna bathing has been reported to reduce the risk of ischemic stroke [Citation24] and dementia[Citation25]. Additionally, evidence indicates that head-out water immersion (HOWI) in hot water increases peripheral conduit artery and microvascular endothelial function following acute [Citation26] and chronic exposures [Citation27,Citation28] likely due to shear stress mediated upregulation of nitric oxide production. However, internal carotid artery and vertebral artery blood flow are reduced during supine passive heat stress largely due to acute hypocapnia [Citation8] as well as decreases in blood pressure [Citation8,Citation29,Citation30]. Despite these acute reductions in CBF, cerebrovascular function remains largely unchanged [Citation31,Citation32] or is improved [Citation33,Citation34] with heat stress in the absence of water immersion. Thermoneutral HOWI increases CBF and cerebral blood velocity via augmented mean arterial pressure [Citation35,Citation36] and PETCO2 [Citation36,Citation37]. Thus, hot water immersion might attenuate the reductions in CBF that are observed during non-immersed passive heating conditions due to hydrostatic pressure and increases in PETCO2. In this context, little is known regarding whether the benefits of hot HOWI that have been observed to improve peripheral vascular function also occur in the cerebral vasculature. Bailey et al. demonstrated that 8-weeks of hot HOWI augmented mean resting middle cerebral artery blood velocity (MCAvmean) and attenuated reductions in MCAvmean during supine passive heat stress in young healthy females[Citation38]. These findings provide some evidence that repetitive hot HOWI might improve cerebrovascular function. To this end, the repetitive acute effects of hot HOWI might lead to beneficial adaptations that are associated with a hot HOWI intervention. The purpose of our study was to determine if an acute session of hot HOWI improves indices of cerebrovascular function. We utilized two separate studies to test the hypotheses that hot HOWI acutely improves 1) dynamic cerebral autoregulation and 2) cerebrovascular reactivity to hypercapnia in healthy young adults compared to thermoneutral HOWI.
Methods
Participants
Eighteen healthy adults (age: 23 ± 2 y; height: 174 ± 11 cm; weight: 71 ± 14 kg; 8 females) completed the Dynamic Cerebral Autoregulation study that tested the hypothesis that hot HOWI acutely improves dynamic cerebral autoregulation. Fourteen healthy adults (age: 22 ± 3 y; height: 173 ± 10 cm; weight: 70 ± 13 kg; 6 females) completed the Cerebrovascular Reactivity to Hypercapnia study that tested the hypothesis that hot HOWI acutely increases cerebrovascular reactivity using hypercapnia. Based on feasibility data, we estimate that we would achieve an effect size (f) of 0.14 for the interaction term for the main outcome variables of both studies. Thus, using an alpha level of 0.05 and 80% power, our a priori sample size calculation determined we would need 14 participants to detect an interaction effect. Both studies consisted of three visits: a screening visit and two experimental visits. Both studies utilized identical methods and experimental approaches with the exception of the cerebrovascular function tests. All participants were fully informed of the experimental procedures prior to providing informed and written consent. Participants were excluded for self-reporting beta-blocker, alpha-blocker, angiotensin converting enzyme inhibitor, angiotensin receptor blocker use, or the use of any other medication that affects blood pressure or the autonomic nervous system; current or history of digestive tract disorders (irritable bowel syndrome, hemorrhoids, diarrhea, constipation, cancer, surgery); preexisting autonomic, cardiovascular, metabolic, respiratory, or endocrine disorder; major depression; pregnancy; or breastfeeding. Female participants were tested within the first 10 days from onset of menses and self-reported to use contraceptives (Dynamic Cerebral Autoregulation study: n = 3; 1 implant and 2 intrauterine devices; Cerebrovascular Reactivity to Hypercapnia study: n = 1; intrauterine device) or to not use contraceptives (Dynamic Cerebral Autoregulation study: n = 5; Cerebrovascular Reactivity to Hypercapnia study: n = 5). The study was approved by the Institutional Review Board at the University at Buffalo and was performed in accordance with the standards set by the latest revision of the Declaration of Helsinki.
Instrumentation and measurements
Height and nude body weight were measured with a stadiometer and scale (Sartorius, Bohemia, NY) and urine-specific gravity was measured via refractometry (Atago USA, Bellevue, WA) before each experimental visit. An intestinal temperature telemetry pill (HQ Inc., Palmetto, FL, USA) was ingested 1 hour prior to baseline measurements [Citation39] and intestinal temperature was recorded every 5 minutes during the studies. Heart rate was continuously measured using a 3-lead electrocardiogram (ECG; DA100C, Biopac Systems, Goleta, CA). Blood pressure was continuously measured using finger photoplethysmography on the right hand that was supported out of the water (Finometer Pro, FMS, Amsterdam, Netherlands). Blood pressure was corrected to the heart level using a height correction sensor. End-tidal CO2 tension (PETCO2) was continuously measured using capnography (Nonin Medical, Inc., Plymouth, MN). Arterial oxygen saturation was continuously measured using a pulse oximeter on the right index finger (Nonin Medical, Inc., Plymouth, MN). Right MCAvmean was continuously measured as an index of blood flow to the anterior cerebral circulation via transcranial Doppler sonography via a 2 MHz probe (DWL USA, Inc., Germany, Europe) using insonation techniques described in detail by Willie et al. [Citation40]. The depth, gain, and location of the right MCA were recorded during the first experimental visit and used in the second experimental visit. Images of the right common carotid artery (CCA) were taken prior to each cerebrovascular function test using a 10 MHz linear transducer and a Doppler ultrasound (Vivid 7 Dimension, GE, 2010). We chose the CCA as our vessel of interest as changes within this major artery (e.g., diameter and intima-media thickness) are associated with an increased risk of stroke[Citation41]. Brightness mode (B-mode) and pulse-wave mode (PW mode) were simultaneously used to determine vessel diameter and blood velocity, respectively. The insonation angle was <60° and the sample volume was fixed in the center of the vessel and covered approximately 2/3 of the vessel diameter. The insonation angle and sample volume of the right CCA were recorded during the first experimental visit and used in the second experimental visit. All CCA images were obtained by the same sonographer (MLW). The within-subject test–retest coefficient variation for CCA diameter was 1.2 ± 1.0% and CCA blood flow was 3.2 ± 2.8% (n = 8).
Experimental approach
The two randomized experimental visits consisted of: (i) a thermoneutral HOWI visit (35 °C water temperature) and (ii) a hot HOWI visit (39 °C water temperature). Experimental visits were completed at least 7 days apart and at the same time of day. Participants reported to the laboratory after abstaining from strenuous exercise, alcohol, and caffeine for 12 hours, and food for 2 hours. Upon arrival, participants ingested the temperature telemetry pill and provided a urine sample to assess hydration status via urine-specific gravity. At this point, participants were not allowed to consume fluids throughout the duration of the study visit. We assessed the pregnancy status (i.e., confirmed negative) in women of childbearing potential via a urine pregnancy test, and they reported the start date of their last menstrual cycle. After the urine sample was obtained, participants provided a nude body weight in a private room. Men wore shorts and women wore shorts and a sports bra during both experimental visits. ) and 1 C illustrates the experimental set-up for both studies. After the urine sample was collected, participants then entered the empty water immersion tank and assumed a seated position with their right arm supported on a platform at heart level, and were instrumented for the measurements of heart rate, blood pressure, arterial oxygen saturation, PETCO2, and MCAvmean. After ≥10 minutes of quiet seated rest, a 5-minute baseline period commenced, followed by a cerebrovascular function test (PRE). Following PRE, the water immersion tank was rapidly filled with water to the level of the sternal notch, which occurred over ~4 minutes. Cerebrovascular function tests were initiated 30 minutes into the 45 minutes of HOWI, immediately post-HOWI (POST-1), and 45 minutes post-HOWI (POST-2). Following the cerebrovascular function test performed during HOWI, the water immersion tank was rapidly emptied within ~4 minutes. We chose to test at 30 minutes of HOWI based on prior work by Bailey et al. [Citation38] that investigated the effects of repetitive 30 minute hot water immersion sessions on cerebral blood velocity. Thus, we aimed to determine the acute effects of water immersion on cerebrovascular function while in the water (at 30 minutes) and out of the water.
Figure 1. (a)Test of dynamic cerebral autoregulation using a respiratory impedance device. (b) Typical tracing of arterial blood pressure (top; blue), PETCO2 (middle; red) and right MCAv (bottom; pink) while breathing through the respiratory impedance device. (c) Test of cerebrovascular reactivity using a stepped hypercapnia protocol. (d) Typical tracing of the PETCO2 waveform (top; blue) and right MCAv (bottom; pink) at baseline breathing room air and throughout the stepped hypercapnia protocol
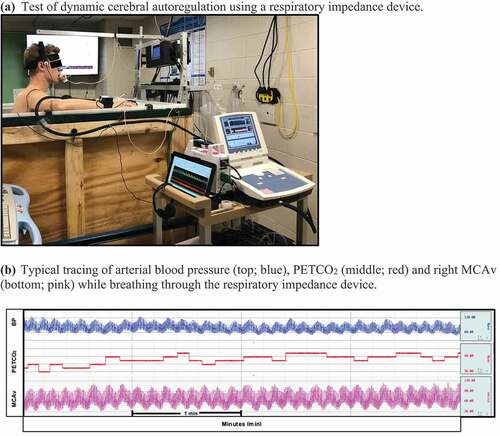
Dynamic cerebral autoregulation
Dynamic cerebral autoregulation (dCA) was assessed using a respiratory impedance device set to a pressure of 8–10 cm H2O to induce oscillatory changes in blood pressure. Based on feasibility data, we expected blood pressure to increase by ~8 mm Hg during inspiration and decrease by ~6 mm Hg during expiration. Participants breathed through the device while wearing a nose clip for 5 minutes and were instructed to maintain baseline PETCO2 (±1 mm Hg). Continuous visual feedback using the capnograph and verbal feedback was provided (i.e., “speed up your breathing” if PETCO2 increased; “slow down your breathing” if PETCO2 decreased).
Cerebrovascular reactivity to hypercapnia
Cerebrovascular reactivity (CVR) was assessed using a stepped hypercapnia protocol [Citation42–44]. Participants inhaled 3%, 5%, and 7% CO2 for 3 minutes each through a two-way valve (Hans Rudolph, Inc., Shawnee, KS, USA). The hypercapnic gases contained 21% O2 and were balanced with nitrogen.
Data analyses
Heart rate (1000 Hz), blood pressure (62.5 Hz), stroke volume (62.5 Hz), PETCO2 (62.5 Hz), respiratory rate (62.5 Hz), and MCAvmean (1000 Hz) were continuously recorded using a data acquisition system (Biopac MP150, AcqKnowledge, 4.2.0, Goleta, CA). Stroke volume was estimated using Modelflow [Citation45] and cardiac output was calculated as the product of heart rate and stroke volume. MCA conductance was calculated as the quotient of the MCAmean and mean arterial pressure. Total peripheral resistance was calculated as the quotient of mean arterial pressure and cardiac output. Offline ultrasound CCA diameter (cm) and mean blood velocity (cm/s) values were obtained from a longitudinal section in B-mode and PW-mode, respectively. The mean values obtained were then used to calculate blood flow (ml/min) and shear rate (s−1)[Citation46].
Blood flow was calculated as:
Shear rate was calculated as:
Dynamic cerebral autoregulation
Mean values were calculated for each five-minute baseline period prior to breathing through the respiratory impedance device. Mean values of PETCO2 were calculated for each minute of the five-minute testing period due to the potential influence that arterial CO2 may have on cerebral autoregulation. Raw data files of beat-to-beat blood pressure (input signal) and MCAv (output signal or response) were used to assess dynamic cerebral autoregulation (WinCPRS, Absolute Aliens Oy, Turku, Finland). These analog signals were integrated with the R-R intervals of the ECG, linearly interpolated, and re-sampled at 5 Hz to align the beat-to-beat time series for transfer function analysis. Data were analyzed by fast Fourier transformation using a Hanning window with sliding bandwidth of 180 s and 50% overlap to reduce cross-spectral leakage. Coherence and transfer function gain and phase were calculated in the very-low frequency (VLF; 0.02–0.07 Hz), low-frequency (LF; 0.07–0.20 Hz), and high-frequency band (HF; 0.20–0.50 Hz) based on Welch’s method. Transfer gain and phase reflect the magnitude and time delay between alterations in blood pressure and MCAv within a given frequency. A decrease in transfer gain and an increase in transfer phase are indicative of an “improvement” in cerebral autoregulation as this reflects a greater ability of the cerebral vasculature to buffer changes in arterial blood pressure. Negative phase values were excluded from averaging in the VLF and LF bands due to the “wrap-around” effect[Citation47]. Additionally, raw data files of blood pressure and MCAv were visually inspected for artifact. Participants were excluded from the transfer function analysis if excessive artifact persisted beyond 3 beats (n = 4). [Citation47] An additional participant was excluded from MCAvmean and MCA conductance calculations due to excessive head movement. CCA variables were analyzed from n = 13 due to alterations in the insonation angle between measurements.
Cerebrovascular reactivity to hypercapnia
Mean values were calculated over the final 2 minutes of baseline and every 30 seconds during the hypercapnia protocol. These values were then plotted and cerebrovascular reactivity to hypercapnia was calculated as the slope of the regression line between MCAv and PETCO2 using linear regression analysis [Citation42–44]. CCA variables were analyzed from n = 12 due to alterations in insonation angle between measurements.
Statistical analyses
Data were analyzed using PRISM software (Version 8, GraphPad Software, La Jolla, CA). No comparisons were made between the Dynamic Cerebral Autoregulation study and the Cerebrovascular Reactivity to Hypercapnia study. We used two-tailed paired t-tests to determine if PRE values were different between experimental visits within a study. For both studies, we used mixed-effects ANOVAs to compare the change (∆) from PRE between and within trials. If an ANOVA revealed an interaction or main effect [Citation48], we used Holm–Sidak post hoc analyses to determine where differences occurred. Exact P-values are reported where appropriate. Data are reported as the change from PRE (mean ± SD).
Results
Effects of head-out water immersion on dynamic cerebral autoregulation
Participants arrived hydrated for both thermoneutral (urine-specific gravity: 1.012 ± 0.009) and hot HOWI visits (urine-specific gravity: 1.014 ± 0.008).
Intestinal temperature
Intestinal temperature was not different between conditions at PRE (). In TNdCA, the intestinal temperature slightly decreased from PRE during HOWI (-0.2 ± 0.2 °C; P = 0.004). In HOTdCA, intestinal temperature increased from PRE during HOWI (0.5 ± 0.2 °C; P < 0.001), at POST-1 (0.6 ± 0.3 °C; P < 0.001), and at POST-2 (0.4 ± 0.2 °C; P < 0.001) ().
Table 1. Dynamic cerebral autoregulation pre-immersion baseline values
Figure 2. Dynamic cerebral autoregulation study change in intestinal temperature from PRE to 30 min of head-out water immersion, immediately post-immersion, and 45 min post-immersion in thermoneutral (35 °C) and hot (39 °C) water. B = different from PRE (P ≤ 0.05), * = different between conditions (P ≤ 0.05). n = 17
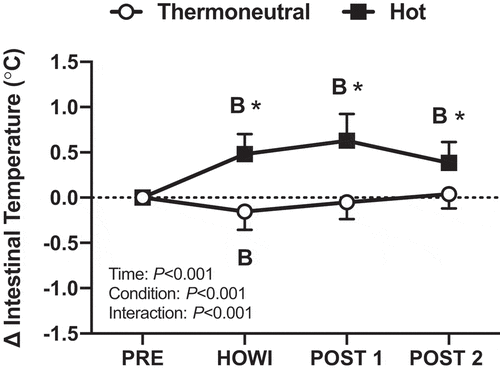
Cardiovascular responses
Stroke volume was lower in TNdCA at PRE, but no other cardiovascular variables were different at PRE between conditions (). In TNdCA, heart rate decreased from PRE during HOWI (P < 0.001) and remained attenuated at POST-1 (P < 0.001) and POST-2 (P = 0.011). The change in heart rate was greater in HOTdCA versus TNdCA throughout the study (all P < 0.001) ()). The change in mean arterial pressure was greater in TNdCA (all P < 0.001) ()). In TNCA, stroke volume increased from PRE during HOWI (P < 0.001). In HOTdCA, stroke volume decreased from PRE at POST-2 (P < 0.001) ()). In TNdCA, cardiac output did not change (P ≥ 0.076), whereas cardiac output increased from PRE during HOWI in HOTdCA (P < 0.001) (). In TNdCA, total peripheral resistance increased from PRE at POST-1 and POST-2 (P < 0.001). In HOTdCA, total peripheral resistance decreased from PRE during HOWI (P < 0.001) (). In TNdCA, arterial oxygen saturation increased from PRE during HOWI (P = 0.015) ()).
Figure 3. Dynamic cerebral autoregulation study changes in heart rate (a), mean arterial pressure (b), stroke volume (c), cardiac output (d), total peripheral resistance (e), and arterial oxygen saturation (f) from PRE to 30 min of head-out water immersion, immediately post-immersion, and 45 min post-immersion in thermoneutral (35 °C) and hot (39 °C) water. B = different from PRE (P ≤ 0.05), * = different between conditions (P ≤ 0.05). n = 18
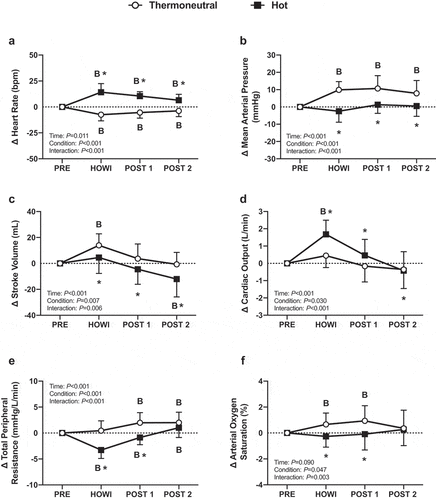
Respiratory rate and PETCO2
Respiratory rate and PETCO2 were not different between conditions at PRE (). Respiratory rate did not change from PRE (P = 0.343) and was not different between conditions (P = 0.068) (). In TNdCA, PETCO2 increased from PRE during HOWI (P < 0.001) but decreased at POST-1 (P = 0.018) and POST-2 (P = 0.045). In HOTdCA, PETCO2 decreased from PRE at POST-1 and POST-2 (both P < 0.001). The change in PETCO2 was greater in TNdCA during HOWI (P = 0.025) but was greater in HOTdCA at POST-1 (P = 0.060) and POST-2 (P = 0.060) (). Additionally, the change in PETCO2 during the dCA tests was not different between conditions at any time point ().
Figure 4. Dynamic cerebral autoregulation study change in respiratory rate (a) and PETCO2 (b) from PRE to 30 min of head-out water immersion, immediately post-immersion, and 45 min post-immersion in thermoneutral (35 °C) and hot (39 °C) water. B = different from PRE (P ≤ 0.05), * = different between conditions (P ≤ 0.05). n = 18
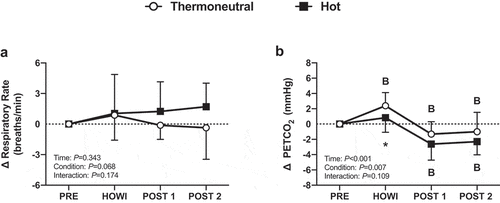
Figure 5. Changes in PETCO2 from baseline (BL) immediately prior to breathing on the respiratory impedance device (BL) to each minute of the dynamic cerebral autoregulation testing at PRE (a), 30 min of head-out water immersion (b), immediately post-immersion (c), and 45 min post-immersion (d) in thermoneutral (35 °C) and hot (39 °C) water during the dynamic cerebral autoregulation study. B = different from BL (P ≤ 0.05). n = 15
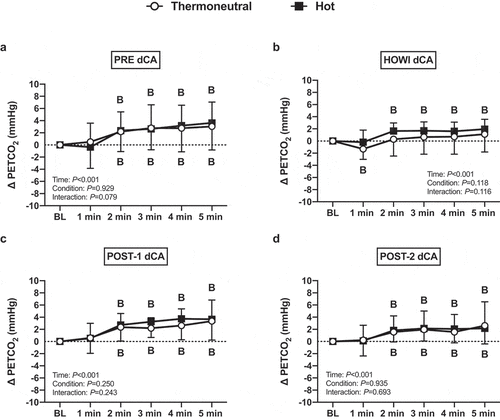
Cerebrovascular responses
( is a typical tracing of the blood pressure, MCAv, and PETCO2 signals during the dCA assessment. Cerebrovascular variables were not different at PRE (). In HOTdCA, MCAvmean decreased from PRE during HOWI (P = 0.036) and remained lower at POST-1 (P = 0.002) and POST-2 (P < 0.001). The magnitude of change for MCAvmean was lower in HOTdCA during HOWI (P = 0.007), at POST-1 (P = 0.026), and POST-2 (P = 0.026) compared to TNdCA (). In TNdCA, MCA conductance decreased from PRE throughout the study (all P ≤ 0.002). In HOTdCA, MCA conductance decreased from PRE at POST-1 (P = 0.003) and POST-2 (P < 0.001) (). In TNdCA and HOTdCA, VLF coherence decreased from PRE during HOWI (TNCA: P = 0.027; HOTCA: P = 0.002) (). The change in VLF coherence was greater in TNdCA at POST-2 (P = 0.044). The ANOVA did not reveal an interaction or main effect of LF and HF coherence, or VLF gain (). The change in LF gain was different between conditions during HOWI (P = 0.038) (). In HOTdCA, HF gain increased from PRE during HOWI (P = 0.037). The change in HF gain was greater than TNdCA during HOWI (P < 0.001) and at POST-1 (P = 0.016) ()). The ANOVA did not reveal a significant interaction or main effect for phase in any frequency band (). In TNdCA and HOTdCA, CCA diameter increased from PRE during HOWI (TNCA: P = 0.052; HOTCA: P = 0.006) ()). In HOTdCA, CCA blood velocity increased from PRE during HOWI (P = 0.002) ()). The ANOVA did not reveal an interaction or main effect of CCA blood flow ()). In HOTdCA, the CCA shear rate increased from PRE during HOWI (P = 0.038). In TNdCA, the CCA shear rate decreased from PRE during HOWI (P = 0.054) ()). The CCA shear rate was greater during HOTdCA HOWI (P < 0.001).
Figure 6. Dynamic cerebral autoregulation study changes in MCAvmean (a) and MCA conductance (b) from PRE to 30 min of head-out water immersion, immediately post-immersion, and 45 min post-immersion in thermoneutral (35 °C) and hot (39 °C) water. B = different from PRE (P ≤ 0.05), * = different between conditions (P ≤ 0.05). n = 14
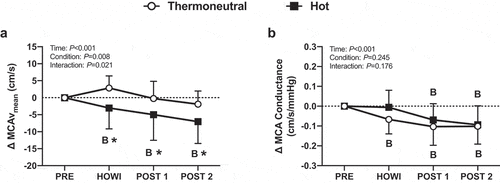
Figure 7. Dynamic cerebral autoregulation study changes in coherence (a-c), phase (d-f), and gain (g-i) in the very-low frequency (VLF; 0.02–0.07 Hz), low-frequency (LF; 0.07–0.20 Hz), and high-frequency (HF; 0.20–0.50 Hz) band from PRE to 30 min of head-out water immersion, immediately post-immersion, and 45 min post-immersion in thermoneutral (35 °C) and hot (39 °C) water. B = different from PRE (P ≤ 0.05). * = different between conditions (P ≤ 0.05). n = 15
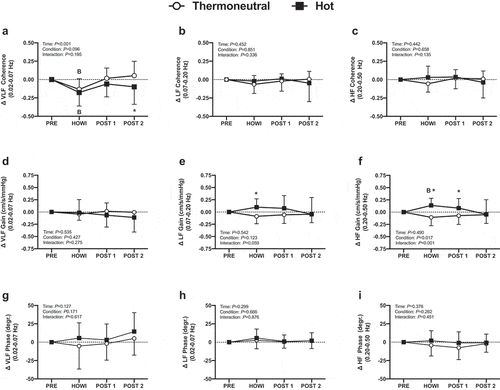
Figure 8. Dynamic cerebral autoregulation study changes in common carotid artery diameter (a), mean blood velocity (b), mean blood flow (c), and shear rate (d) from PRE to 30 min of head-out water immersion, immediately post-immersion, and 45 minutes post-immersion in thermoneutral (35 °C) and hot (39 °C) water. B = different from PRE (P ≤ 0.05). * = different between conditions (P ≤ 0.05). n = 13
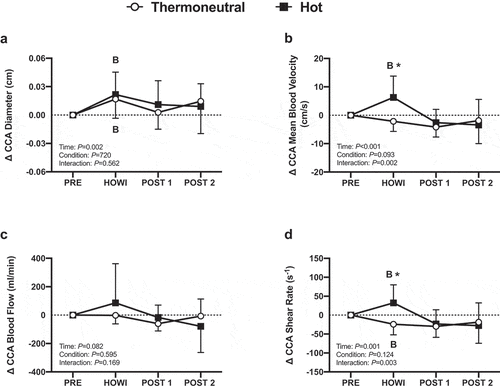
Effects of head-out water immersion on cerebrovascular reactivity
Participants arrived hydrated for both thermoneutral (urine-specific gravity: 1.017 ± 0.009) and hot HOWI visits (urine-specific gravity: 1.015 ± 0.008).
Intestinal temperature
Intestinal temperature was not different between conditions at PRE (). The change in intestinal temperature was greater in HOTCVR ().
Table 2. Cerebrovascular reactivity to hypercapnia pre-immersion baseline values
Figure 9. Cerebrovascular reactivity to hypercapnia study change in intestinal temperature from PRE to 30 min of head-out water immersion, immediately post-immersion, and 45 min post-immersion in thermoneutral (35 °C) and hot (39 °C) water. B = different from PRE (P ≤ 0.05), * = different between conditions (P ≤ 0.05). n = 14
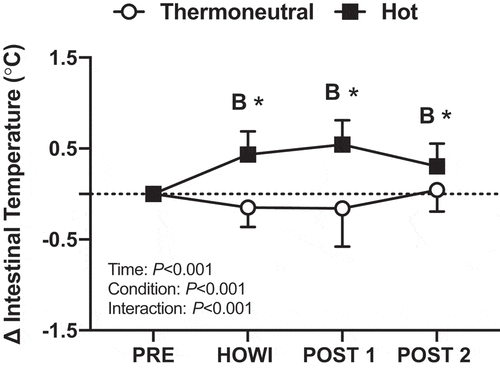
Cardiovascular responses
Cardiovascular variables were not different between conditions at PRE (). The change from PRE was different between TNCVR and HOTCVR at every time point for heart rate (all P < 0.001), mean arterial pressure (all P ≤ 0.005), stroke volume (all P < 0.001), and arterial oxygen saturation (all P ≤ 0.039) ( (a–c, f)). The change from PRE in cardiac output was not different between conditions (). The change from PRE in total peripheral resistance was different between conditions at HOWI and POST-1 (both P < 0.001) ().
Figure 10. Cerebrovascular reactivity to hypercapnia study changes in heart rate (a), mean arterial pressure (b), stroke volume (c), and cardiac output (d), total peripheral resistance (e), and arterial oxygen saturation (f) from PRE to 30 min of head-out water immersion, immediately post-immersion, and 45 min post-immersion in thermoneutral (35 °C) and hot (39 °C) water. B = different from PRE (P ≤ 0.05), * = different between conditions (P ≤ 0.05). n = 14
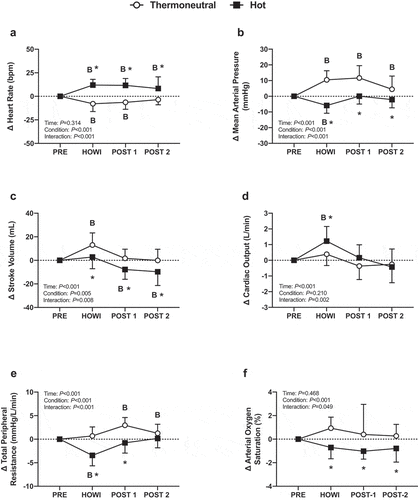
Respiratory rate and PETCO2
Respiratory rate and PETCO2 were not different between conditions at PRE (). In TNCVR, respiratory rate increased from PRE during HOWI (P = 0.034) and POST-2 (P = 0.021). In HOTCVR, respiratory rate increased from PRE during HOWI (P = 0.025) and at POST-2 (P = 0.056) ()). In HOTCVR, PETCO2 increased from PRE during HOWI (P = 0.041), but the change was not different between conditions ()).
Cerebrovascular responses
) is a typical tracing of the PETCO2 and MCAv signals during the cerebrovascular reactivity to hypercapnia test. Cerebrovascular variables were not different between conditions at PRE (). In TNCVR, MCAvmean increased from PRE during HOWI (P < 0.001) and remained elevated at POST-1 (P = 0.005). The change in MCAvmean was greater in TNCVR during HOWI (P = 0.002) ()). The ANOVA did not reveal a significant interaction for MCA conductance ()) or for cerebrovascular reactivity to hypercapnia. However, cerebrovascular reactivity exhibited a time main effect, but the Holm–Sidak multiple comparisons tests were unable to detect any differences from PRE in either condition (P ≥ 0.294) ()). The change from PRE in CCA diameter, mean blood velocity, and shear rate were similar to those observed in the Dynamic Cerebral Autoregulation study (). In HOTCVR, CCA blood flow increased from PRE during HOWI (all P < 0.001) ()).
Figure 12. Cerebrovascular reactivity to hypercapnia study changes in MCAvmean (a), MCA conductance (b) and MCA cerebrovascular reactivity to hypercapnia (c) from PRE to 30 min of head-out water immersion, immediately post-immersion, and 45 min post-immersion in thermoneutral (35 °C) and hot (39 °C) water. B = different from baseline (P ≤ 0.05), *different between conditions (P ≤ 0.05). n = 14
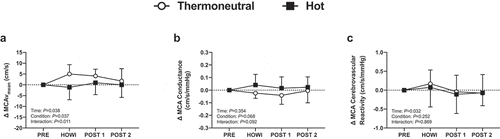
Figure 13. Cerebrovascular reactivity to hypercapnia study changes in common carotid artery diameter (a), mean blood velocity (b), mean blood flow (d), and shear rate (f) from PRE to 30 min of head-out water immersion, immediately post-immersion, and 45 minutes post-immersion in thermoneutral (35 °C) and hot (39 °C) water. B = different from PRE (P ≤ 0.05). * = different between conditions (P ≤ 0.05). n = 12
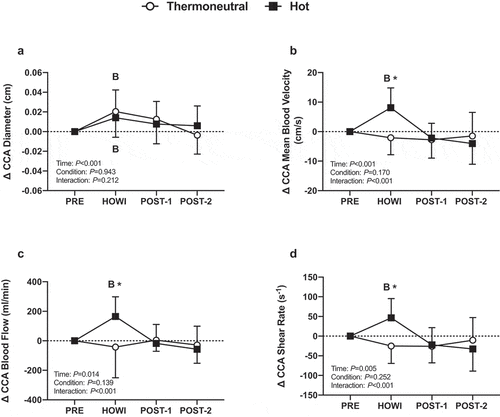
Discussion
We investigated the cerebrovascular responses during and following 45 minutes of thermoneutral and hot HOWI in healthy, young adults. Our major novel findings are that hot water immersion does not significantly alter dynamic cerebral autoregulation or cerebrovascular reactivity to hypercapnia in the MCA.
Thermoneutral HOWI augmented MCAvmean ~4–8% in both studies. We observed increases in stroke volume and mean arterial pressure during thermoneutral HOWI, which indicates a rise in thoracic blood volume. Additionally, we observed similar increases in PETCO2 during thermoneutral HOWI as we have previously (~2 mm Hg)[Citation37]. Thus, the increases in MCAvmean are likely due to the combined effects of the rise in mean arterial pressure and PETCO2 during thermoneutral HOWI [Citation36,Citation37].
Hot HOWI decreased MCAvmean by ~2–3% in both studies. During hot HOWI, we observed a small decrease in mean arterial pressure in study 1 (-3 mm Hg) and study 2 (-6 mm Hg). Reductions in blood pressure have been reported in healthy individuals during hot HOWI [Citation49] and during lower limb immersion in hot water[Citation50]. Unlike investigations that have used water perfused suits to induce passive heat stress [Citation29,Citation33], we did not observe a decrease in PETCO2 during hot HOWI. The increase in PETCO2 that occurs during the thermoneutral HOWI [Citation35–37,Citation51] potentially offsets a hyperventilation-induced decrease in PETCO2 during hot HOWI that would otherwise occur during passive heat stress. Additionally, the intestinal temperature that was reached during our studies was below the core body temperature threshold (~38.5 °C) at which ventilation typically increases[Citation52]. Although skin blood flow likely increased during hot HOWI, we speculate that hydrostatic pressure during hot HOWI attenuated the increase in skin blood flow and/or augmented venous return. This response along with the increased heart rate and subsequent rise in cardiac output during hot HOWI mitigated the potential decrease in mean arterial pressure. Collectively, the PETCO2 and mean arterial pressure responses during hot HOWI likely attenuated the decrease in MCAvmean (combined mean decrease of 3.4% from PRE) when compared to other passive heat stress models. For instance, decreases of ~11% in MCAvmean have been observed during seated passive heat stress (~0.5°C increase in esophageal temperature) induced by the combination of an upper body water-perfused suit and hot water immersion to the iliac crest [Citation53,Citation54]. Although MCAvmean increased during thermoneutral HOWI, we found that CCA blood flow did not change and CCA shear rate decreased. However, CCA blood flow and shear rate increased during hot HOWI. These differences between thermoneutral and hot HOWI are partially due to the decrease in total peripheral resistance (combined mean for both studies: TN: 0.6% and HOT: -3.4%) during hot HOWI. Most of the increases in blood flow and shear rate that we observed in the CCA were probably directed to the external carotid artery for thermoregulation during hot HOWI. In support of this, Sato et al. [Citation55] observed an increase in external carotid artery blood flow while internal carotid artery blood flow decreased.
Nevertheless, the increases in CCA blood flow and shear rate during hot HOWI could be important modulators for improving cerebral artery endothelial function[Citation56]. For instance, microvascular and macrovascular endothelial functions are improved following acute [Citation26] and chronic heat therapy[Citation27], likely due to increases in micro- and macrovascular blood flow and shear rate. Future work should determine if extra-cranial conduit artery vasomotor function improves following acute and chronic hot HOWI.
Dynamic cerebral autoregulation
We found that dynamic cerebral autoregulation was largely unaltered during and following thermoneutral HOWI. However, we observed an increase from baseline in HF gain as well as a difference between conditions in HF and LF gain during hot HOWI. Additionally, coherence decreased in the VLF band during thermoneutral and hot HOWI but there were no changes in phase within any frequency band for either condition. To the best of our knowledge, there are no published studies investigating the effects of acute thermoneutral water immersion on cerebral autoregulation. However, Kermorgant et al. investigated spontaneous cerebral autoregulation following 3 d of dry immersion in temperate conditions[Citation57]. These investigators reported an increase in low-frequency phase and no change in gain (all frequency bands) at rest[Citation57]. Thus, our results likely differ due to assessing different components of cerebral autoregulation (i.e., spontaneous versus dynamic) and the duration of immersion.
Doering et al. measured cerebral autoregulation during 30 minutes of a progressive hot HOWI challenge (36–42 °C) that increased core temperature from 36.8 to 37.2 °C [Citation49]. Using the thigh cuff deflation technique, Doering et al. found that the cerebral autoregulatory index, which reflects the time course of the change in cerebral blood velocity to a rapid decrease in blood pressure, was improved during hot HOWI[Citation49]. The decrease in mean arterial pressure following thigh cuff release was not reported; thus, the stimulus for autoregulation (i.e., decrease in mean arterial pressure) in Doering et al. is unclear. We used a respiratory impedance device to induce oscillatory increases and decreases in mean arterial pressure (). This allowed us to assess cerebral autoregulation throughout a broad range of mean arterial pressures, whereas the cuff deflation technique only assesses cerebral autoregulation in response to falling blood pressures. Several other investigators have examined cerebral autoregulation in healthy adults during passive heat stress via water perfused suits [Citation33,Citation34]. These studies examined cerebral autoregulation in the supine position using oscillatory lower body negative pressure [Citation33] and thigh cuff deflation [Citation34] during greater hyperthermic stress (+1.0 °C) [Citation33,Citation34] versus our hot HOWI conditions (+0.6 °C). These passive heat stress studies demonstrated that the low-frequency gain did not change [Citation33,Citation34] but phase increased in the low-frequency band and gain decreased in the very-low-frequency band [Citation33,Citation34]. Therefore, these findings indicate that cerebral autoregulation during passive heat stress in the supine position is improved. Our results contradict these previous findings as we observed increases in high-frequency gain during hot HOWI but no change in phase was observed across all frequency bands. Thus, it appears that dynamic cerebral autoregulation is altered in the HF band due to a greater magnitude of changes in blood pressure being translated to the cerebral vasculature. However, cerebral autoregulation primarily operates in the VLF and LF band where we observed no differences from pre-immersion in either condition for gain and phase. Therefore, we do not interpret our data to suggest that acute hot HOWI has a robust negative impact on dynamic cerebral autoregulation in healthy adults. The discrepancies between our study and the prior investigations could be due to the method of thermal stress (i.e., hot water perfused suits vs. hot HOWI), the severity and duration of thermal stress, or the assessment of cerebral autoregulation. Nevertheless, more work needs to be done to determine how a greater thermal stress during hot HOWI and/or how repetitive hot HOWI impacts cerebral autoregulation.
Cerebrovascular reactivity to hypercapnia
Contrary to our hypothesis, we did not observe any changes in cerebrovascular reactivity to hypercapnia during and following hot HOWI. To the best of our knowledge, we are the first to examine cerebrovascular reactivity to hypercapnia during and following an acute session of hot HOWI. Nitric oxide (NO) produced in the cerebral vasculature contributes to the increases in MCAvmean and CBF in response to hypercapnia [Citation58] and thus cerebrovascular reactivity might provide an index of nitric oxide bioavailability in the cerebral vasculature. Although we found an increase in CCA shear rate during hot HOWI, it does not appear that shear-induced nitric oxide production translated to an improvement in intra-cranial vascular function (i.e., nitric oxide bioavailability). Likewise, investigations that have used water perfused suits have also found that MCA reactivity to hypercapnia is not changed during passive heat stress [Citation30–32]. However, Ogoh et al. found that the internal carotid artery blood flow response to hypercapnia was attenuated during severe passive heat stress (1.50 °C increase in esophageal temperature)[Citation30]. Collectively, it appears as though heat stress induced by water immersion or by water perfused suits does not alter intracranial vascular reactivity to hypercapnia.
The cerebrovascular responses to passive heat stress in or out of the water appear to be different than the peripheral vascular responses. For instance, peripheral microvascular [Citation27] and conduit artery [Citation28] function following heat stress are both augmented [Citation27,Citation28]. One reason for the differential responses to heat stress between the vascular beds could be the techniques employed to interrogate vascular function. The estimation of nitric oxide bioavailability using cerebrovascular reactivity to hypercapnia is different than how vascular function is assessed in the microcirculation (e.g., microdialysis, local heating, etc.) and peripheral conduit arteries (e.g., flow-mediated dilation, acetylcholine infusion, etc.) thus making a direct comparison between the vascular beds difficult.
We found that MCA reactivity to hypercapnia did not change during or following thermoneutral HOWI. Our findings differ from Sackett et al. who demonstrated a decrease in cerebrovascular reactivity during and following 1 h of head-out immersion in 35 °C water[Citation37]. Several factors might have contributed to the different responses. We previously observed increases in PETCO2 during thermoneutral HOWI [Citation37,Citation59,Citation60]. From those studies, we speculated that the increase in PETCO2 might have contributed to the decrease in cerebrovascular reactivity to hypercapnia during thermoneutral HOWI because we also found that breathing hypercapnic gas to raise PETCO2 also reduced MCA reactivity to hypercapnia[Citation37]. Although we did not observe a statistically significant rise in PETCO2 during thermoneutral HOWI, the increase we observed (~2 mm Hg) is in line with our previous studies (~2 mm Hg) [Citation37,Citation59,Citation60]. Therefore, the discrepancy might be due to differences in cerebrovascular reactivity protocols that were employed. In the current study, we used a stepped hypercapnia protocol whereas we previously used a rebreathe protocol [Citation37]. That being said, increasing PETCO2 by 5 or 10 mm Hg above baseline for 5 minutes elicits similar cerebrovascular reactivity results as a rebreathing protocol[Citation61]. Thus, it is currently unclear why our results differ from our previous investigation.
Experimental considerations
There are multiple experimental considerations that pertain to the interpretation of our data. First, we did not directly measure intracranial artery blood flow. Transcranial Doppler ultrasound is a noninvasive tool used to measure beat-to-beat cerebral blood velocity of intracranial arteries (e.g., MCA and PCA). This tool allows us to assess rapid and dynamic changes in cerebral artery blood velocity. However, it does not provide a measure of vessel diameter. Serrador et al. reported a constant MCA diameter during elevations in PETCO2 (~8 mm Hg) using MRI[Citation62]. However, Giller et al. observed small changes in the MCA diameter (~2–2.5%) during hypo- and hyper-capnia (±10 mm Hg) as well as blood pressure (± 18 mm Hg) during craniotomy[Citation63]. We cannot confirm if the changes observed in MCAvmean are due to alterations in artery diameter, blood flow, or a combination of both. Additionally, we cannot measure blood pressure within the MCA, and thus we assume that changes in blood pressure measured via finger photo plethysmography are reflective of changes in intracranial pressure [Citation64–66]. Second, we only measured the cerebrovascular response to HOWI in the MCA and CCA and thus it is unclear how other intra- or extra-cranial arteries respond to thermoneutral and hot HOWI. Carter et al. [Citation36] reported an increase in both MCAvmean and posterior cerebral artery blood velocity during 10 minutes of thermoneutral water immersion. Additionally, it is likely that the rise in CCA blood flow observed during hot water immersion was primarily driven by blood going to the external carotid artery for heat dissipation. Third, we tested female participants within 10 days of the onset of menses when progesterone and estrogen are low. The menstrual cycle does not alter dynamic cerebral autoregulation [Citation67]; however, it is unknown if there is an interaction among cerebrovascular function, menstrual cycle phase, and hot head-out water immersion. Fourth, we did not measure circulating factors, such as heat shock proteins, that have been shown to increase with hot water immersion [Citation68] which might contribute to improved cerebrovascular health[Citation69]. Lastly, we chose to test cerebrovascular function at 30 minutes of water immersion based on the work of Bailey et al. [Citation38]. It is possible that 1) we did not increase core temperature sufficiently and/or 2) a repetitive stimulus is needed to elicit improvements in cerebrovascular function, as previously reported in the peripheral circulation [Citation26,Citation28,Citation70,Citation71].
Perspectives and significance
Our data indicate that acute hot water immersion alters dynamic cerebral autoregulation in the high-frequency band via augmented transfer gain, but it is likely that these findings are not indicative of a “worsening” in dynamic cerebral autoregulation since phase was not altered in any frequency band and coherence was only altered in the VLF. Additionally, cerebrovascular reactivity to hypercapnia was unaltered during and following acute hot water immersion. Collectively, these findings provide evidence that 30 minutes of hot head-out water immersion does not acutely alter indices of cerebrovascular function in healthy, young adults. However, increases in CCA shear rate during hot HOWI are likely beneficial for improving extracranial vascular function when repeatedly performed. For instance, repeated increases in shear stress improve endothelial function via augmented FMD[Citation72]. Future work should aim to determine if cerebrovascular function is altered during acute hot water immersion with a greater thermal stress or if a repetitive stimulus is needed to elicit beneficial improvements in cerebrovascular function.
Conclusion
We found that acute head-out immersion in hot water resulted in lower middle cerebral artery blood velocity compared to thermoneutral water, but neither condition altered cerebrovascular reactivity to hypercapnia in the middle cerebral artery. Acute hot head-out water immersion influenced transfer gain in the high-frequency band; however, phase was not changed within this band or others. Thus, dynamic cerebral autoregulation does not appear to be altered during or following hot head-out water immersion. Finally, we observed an increase in the common carotid artery shear rate, which likely promotes beneficial vascular adaptations in this extracranial artery, during head out water immersion in hot water.
Abbreviations
CBF = cerebral blood flow
CCA = common carotid artery
cm H2O = centimeters of water
CO2 = carbon dioxide
CVR = cerebrovascular reactivity to hypercapnia
dCA = dynamic cerebral autoregulation
HF = high frequency
HOWI = head-out water immersion
LF = low frequency
MAP = mean arterial pressure
MCA = middle cerebral artery
MCAvmean = mean middle cerebral artery blood velocity
mm Hg = millimeters of mercury
NO = nitric oxide
PETCO2 = end-tidal carbon dioxide tension
USG = urine-specific gravity
VLF = very low frequency
Acknowledgments
We thank the participants for their time and commitment to complete the study.
Disclosure statement
No conflicts of interest, financial or otherwise, are declared by the authors.
Additional information
Funding
References
- Chen JJ, Rosas HD, Salat DH. Age-associated reductions in cerebral blood flow are independent from regional atrophy. Neuroimage. 2011;55(2):468–478.
- Lu H, Xu F, Rodrigue KM, et al. Alterations in cerebral metabolic rate and blood supply across the adult lifespan. Cereb Cortex. 2011;21(6):1426–1434.
- Leeuwis AE, Benedictus MR, Kuijer JPA, et al. Lower cerebral blood flow is associated with impairment in multiple cognitive domains in Alzheimer’s disease. Alzheimers Dement. 2017;13(5):531–540.
- Leeuwis AE, Smith LA, Melbourne A, et al. Cerebral blood flow and cognitive functioning in a community-based, multi-ethnic cohort: the SABRE study. Front Aging Neurosci. 2018;10:279.
- Bangen KJ, Werhane ML, Weigand AJ, et al. Reduced regional cerebral blood flow relates to poorer cognition in older adults with type 2 diabetes. Front Aging Neurosci. 2018;10:270.
- Austin BP, Nair VA, Meier TB, et al. Effects of hypoperfusion in Alzheimer’s disease. J Alzheimers Dis. 2011;26(Suppl 3):123–133.
- Roher AE, Debbins JP, Malek-Ahmadi M, et al. Cerebral blood flow in Alzheimer’s disease. Vasc Health Risk Manag. 2012;8:599–611.
- Bain AR, Smith KJ, Lewis NC, et al. Regional changes in brain blood flow during severe passive hyperthermia: effects of PaCO2 and extracranial blood flow. J Appl Physiol. 2013;115(5):653–659. ( 1985).
- Wolters FJ, Zonneveld HI, Hofman A, et al. Cerebral perfusion and the risk of Dementia: a population-based study. Circulation. 2017;136(8):719–728.
- Meier TB, Bellgowan PS, Singh R, et al. Recovery of cerebral blood flow following sports-related concussion. JAMA Neurol. 2015;72(5):530–538.
- Wang Y, Nelson LD, LaRoche AA, et al. Cerebral blood flow alterations in acute sport-related concussion. J Neurotrauma. 2016;33(13):1227–1236.
- Martin NA, Patwardhan RV, Alexander MJ, et al. Characterization of cerebral hemodynamic phases following severe head trauma: hypoperfusion, hyperemia, and vasospasm. J Neurosurg. 1997;87(1):9–19.
- Kim J, Whyte J, Patel S, et al. Resting cerebral blood flow alterations in chronic traumatic brain injury: an arterial spin labeling perfusion FMRI study. J Neurotrauma. 2010;27(8):1399–1411.
- Shin YB, Kim SJ, Kim IJ, et al. Voxel-based statistical analysis of cerebral blood flow using Tc-99m ECD brain SPECT in patients with traumatic brain injury: group and individual analyses. Brain Inj. 2006;20(6):661–667.
- Tan CO, Meehan WP 3rd, Iverson GL, et al. Cerebrovascular regulation, exercise, and mild traumatic brain injury. Neurology. 2014;83(18):1665–1672.
- Junger EC, Newell DW, Grant GA, et al. Cerebral autoregulation following minor head injury. J Neurosurg. 1997;86(3):425–432.
- Wright AD, Smirl JD, Bryk K, et al. Sport-related concussion alters indices of dynamic cerebral autoregulation. Front Neurol. 2018;9:196.
- Bailey DM, Jones DW, Sinnott A, et al. Impaired cerebral haemodynamic function associated with chronic traumatic brain injury in professional boxers. Clin Sci (Lond). 2013;124(3):177–189.
- Lynch CE, Eisenbaum M, Algamal M, et al. Impairment of cerebrovascular reactivity in response to hypercapnic challenge in a mouse model of repetitive mild traumatic brain injury. J Cereb Blood Flow Metab. 2020;271678X20954015. DOI:https://doi.org/10.1177/0271678X20954015.
- Len TK, Neary JP, Asmundson GJ, et al. Cerebrovascular reactivity impairment after sport-induced concussion. Med Sci Sports Exerc. 2011;43(12):2241–2248.
- Mashour GA, Boock RJ. Effects of shear stress on nitric oxide levels of human cerebral endothelial cells cultured in an artificial capillary system. Brain Res. 1999;842(1):233–238.
- Bliss ES, Wong RH, Howe PR, et al. Benefits of exercise training on cerebrovascular and cognitive function in ageing. J Cereb Blood Flow Metab. 2020;271678X20957807.
- Bolduc V, Thorin-Trescases N, Thorin E. Endothelium-dependent control of cerebrovascular functions through age: exercise for healthy cerebrovascular aging. Am J Physiol Heart Circ Physiol. 2013;305(5):H620–633.
- Kunutsor SK, Khan H, Zaccardi F, et al. Sauna bathing reduces the risk of stroke in Finnish men and women: a prospective cohort study. Neurology. 2018;90(22):e1937–e1944.
- Knekt P, Järvinen R, Rissanen H, et al. Does sauna bathing protect against dementia? Preventive Med Rep. 2020;20(101221).
- Brunt VE, Jeckell AT, Ely BR, et al. Acute hot water immersion is protective against impaired vascular function following forearm ischemia-reperfusion in young healthy humans. Am J Physiol Regul Integr Comp Physiol. 2016;311(6):R1060–R1067.
- Brunt VE, Eymann TM, Francisco MA, et al. Passive heat therapy improves cutaneous microvascular function in sedentary humans via improved nitric oxide-dependent dilation. J Appl Physiol. 2016;121(3):716–723. ( 1985).
- Brunt VE, Howard MJ, Francisco MA, et al. Passive heat therapy improves endothelial function, arterial stiffness and blood pressure in sedentary humans. J Physiol. 2016;594(18):5329–5342.
- Ogoh S, Sato K, Okazaki K, et al. Blood flow distribution during heat stress: cerebral and systemic blood flow. J Cereb Blood Flow Metab. 2013;33(12):1915–1920.
- Ogoh S, Sato K, Okazaki K, et al. Hyperthermia modulates regional differences in cerebral blood flow to changes in CO2. J Appl Physiol. 2014;117(1):46–52. ( 1985).
- Lee JF, Christmas KM, Harrison ML, et al. Cerebral vasoreactivity: impact of heat stress and lower body negative pressure. Clin Auton Res. 2014;24(3):135–141.
- Fan JL, Cotter JD, Lucas RA, et al. Human cardiorespiratory and cerebrovascular function during severe passive hyperthermia: effects of mild hypohydration. J Appl Physiol. 2008;105(2):433–445. ( 1985).
- Brothers RM, Zhang R, Wingo JE, et al. Effects of heat stress on dynamic cerebral autoregulation during large fluctuations in arterial blood pressure. J Appl Physiol. 2009;107(6):1722–1729. ( 1985).
- Low DA, Wingo JE, Keller DM, et al. Dynamic cerebral autoregulation during passive heat stress in humans. Am J Physiol Regul Integr Comp Physiol. 2009;296(5):R1598–1605.
- Shoemaker LN, Wilson LC, Lucas SJE, et al. Swimming-related effects on cerebrovascular and cognitive function. Physiol Rep. 2019;7(20):e14247.
- Carter HH, Spence AL, Pugh CJ, et al. Cardiovascular responses to water immersion in humans: impact on cerebral perfusion. Am J Physiol Regul Integr Comp Physiol. 2014;306(9):R636–640.
- Sackett JR, Schlader ZJ, Cruz C, et al. The effect of water immersion and acute hypercapnia on ventilatory sensitivity and cerebrovascular reactivity. Physiol Rep. 2018;6(20):e13901.
- Bailey TG, Cable NT, Miller GD, et al. Repeated warm water immersion induces similar cerebrovascular adaptations to 8 weeks of moderate-intensity exercise training in females. Int J Sports Med. 2016;37(10):757–765.
- Notley SR, Meade RD, Kenny GP. Time following ingestion does not influence the validity of telemetry pill measurements of core temperature during exercise-heat stress: The journal Temperature Toolbox. Temperature. 2020;8(1):12-20. doi:https://doi.org/10.1080/23328940.2020.1801119
- Willie CK, Colino FL, Bailey DM, et al. Utility of transcranial Doppler ultrasound for the integrative assessment of cerebrovascular function. J Neurosci Methods. 2011;196(2):221–237.
- Sedaghat S, Van Sloten TT, Laurent S, et al. Common carotid artery diameter and risk of cardiovascular events and mortality: pooled analyses of four cohort studies. Hypertension. 2018;72(1):85–92.
- Barnes JN, Schmidt JE, Nicholson WT, et al. Cyclooxygenase inhibition abolishes age-related differences in cerebral vasodilator responses to hypercapnia. J Appl Physiol. 2012;112(11):1884–1890. ( 1985).
- Barnes JN, Taylor JL, Kluck BN, et al. Cerebrovascular reactivity is associated with maximal aerobic capacity in healthy older adults. J Appl Physiol. 2013;114(10):1383–1387. ( 1985).
- Xie A, Skatrud JB, Morgan B, et al. Influence of cerebrovascular function on the hypercapnic ventilatory response in healthy humans. J Physiol. 2006;577(Pt 1):319–329.
- Wesseling KHHJ, Settels JJ, Schreuder JJ. Computation of aortic flow from pressure in humans using a nonlinear, three-element model. J Appl Physiol. 1985;74(5):2566–2573. ( 1985).
- Coolbaugh CL, Bush EC, Caskey CF, et al. FloWave.US: validated, open-source, and flexible software for ultrasound blood flow analysis. J Appl Physiol. 2016;121(4):849–857. ( 1985).
- Claassen JA, Meel-van Den Abeelen AS, Simpson DM, et al. Transfer function analysis of dynamic cerebral autoregulation: a white paper from the international cerebral autoregulation research network. J Cereb Blood Flow Metab. 2016;36(4):665–680.
- Tybout Aas B. Can I test for simple effects in the presence of an insignificant interaction? J Consum Psychol. 2001;10(1–2):9–10.
- Doering TJ, Aaslid R, Steuernagel B, et al. Cerebral autoregulation during whole-body hypothermia and hyperthermia stimulus. Am J Phys Med Rehabil. 1999;78(1):33–38.
- Coombs GB, Vucina D, Caldwell HG, et al. Cerebrovascular function is preserved during mild hyperthermia in cervical spinal cord injury. Spinal Cord. 2019;57(11):979–984.
- Barwood MJ, Burrows H, Cessford J, et al. “Float first and kick for your life”: psychophysiological basis for safety behaviour on accidental short-term cold water immersion. Physiol Behav. 2016;154:83–89.
- Hayashi K. Ventilatory response to increasing body temperature: characteristics and effect on central fatigue. J Phys Fitness Sports Med. 2015;4(2):143–149.
- Tsuji B, Filingeri D, Honda Y, et al. Effect of hypocapnia on the sensitivity of hyperthermic hyperventilation and the cerebrovascular response in resting heated humans. J Appl Physiol. 2018;124(1):225–233. ( 1985).
- Tsuji B, Hoshi Y, Honda Y, et al. Respiratory mechanics and cerebral blood flow during heat-induced hyperventilation and its voluntary suppression in passively heated humans. Physiol Rep. 2019;7(1):e13967.
- Sato K, Oue A, Yoneya M, et al. Heat stress redistributes blood flow in arteries of the brain during dynamic exercise. J Appl Physiol. 2016;120(7):766–773. ( 1985).
- Zhang Y, Liao B, Li M, et al. Shear stress regulates endothelial cell function through SRB1-eNOS signaling pathway. Cardiovasc Ther. 2016;34(5):308–313.
- Kermorgant M, Leca F, Nasr N, et al. Impacts of simulated weightlessness by dry immersion on optic nerve sheath diameter and cerebral autoregulation. Front Physiol. 2017;8:780.
- Iadecola C, Zhang F. Nitric oxide-dependent and -independent components of cerebrovasodilation elicited by hypercapnia. Am J Physiol. 1994;266(2 Pt 2):R546–552.
- Sackett JR, Schlader ZJ, Sarker S, et al. Peripheral chemosensitivity is not blunted during 2 h of thermoneutral head out water immersion in healthy men and women. Physiol Rep. 2017;5(20):e13472.
- Sackett JR, Schlader ZJ, O’Leary MC, et al. Central chemosensitivity is augmented during 2 h of thermoneutral head-out water immersion in healthy men and women. Exp Physiol. 2018;103(5):714–727.
- Brothers RM, Lucas RA, Zhu YS, et al. Cerebral vasomotor reactivity: steady-state versus transient changes in carbon dioxide tension. Exp Physiol. 2014;99(11):1499–1510.
- Serrador JM, Picot PA, Rutt BK, et al. MRI measures of middle cerebral artery diameter in conscious humans during simulated orthostasis. Stroke. 2000;31(7):1672–1678.
- Giller CA, Bowman G, Dyer H, et al. Cerebral arterial diameters during changes in blood pressure and carbon dioxide during craniotomy. Neurosurgery. 1993;32(5):737–741. discussion 741–732.
- Parati G, Casadei R, Groppelli A, et al. Comparison of finger and intra-arterial blood pressure monitoring at rest and during laboratory testing. Hypertension. 1989;13(6 Pt 1):647–655.
- Imholz BP, Wieling W, Van Montfrans GA, et al. Fifteen years experience with finger arterial pressure monitoring: assessment of the technology. Cardiovasc Res. 1998;38(3):605–616.
- Fantini S, Sassaroli A, Tgavalekos KT, et al. Cerebral blood flow and autoregulation: current measurement techniques and prospects for noninvasive optical methods. Neurophotonics. 2016;3(3):031411.
- Favre ME, Serrador JM. Sex differences in cerebral autoregulation are unaffected by menstrual cycle phase in young, healthy women. Am J Physiol Heart Circ Physiol. 2019;316(4):H920–H933.
- Faulkner SH, Jackson S, Fatania G, et al. The effect of passive heating on heat shock protein 70 and interleukin-6: a possible treatment tool for metabolic diseases? Temperature. 2017;4(3):292–304. doi:https://doi.org/10.1080/23328940.2017.1288688.
- Shao A, Zhou Y, Yao Y, et al. The role and therapeutic potential of heat shock proteins in haemorrhagic stroke. J Cell Mol Med. 2019;23(9):5846–5858.
- Brunt VE, Wiedenfeld-Needham K, Comrada LN, et al. Passive heat therapy protects against endothelial cell hypoxia-reoxygenation via effects of elevations in temperature and circulating factors. J Physiol. 2018;596(20):4831–4845.
- Brunt VE, Weidenfeld-Needham KM, Comrada LN, et al. Serum from young, sedentary adults who underwent passive heat therapy improves endothelial cell angiogenesis via improved nitric oxide bioavailability. Temperature. 2019;6(2):169–178. doi:https://doi.org/10.1080/23328940.2019.1614851
- Johnson BD, Mather KJ, Wallace JP. Mechanotransduction of shear in the endothelium: basic studies and clinical implications. Vasc Med. 2011;16(5):365–377.