Abstract
We investigated the effectiveness of artificial silk-based nerve graft in nerve recovery and in helping regain motor functionality in rats. The purpose of this study is to test two questions: (1) Can nerve growth after transection be improved using artificial nerve guides? (2) Does nerve recovery have an impact on the walking patterns of the rat during the recovery period? Rats underwent sciatic nerve sectioning followed by reconstructive surgery. In these experiments, the sciatic nerve was substituted with an artificial silk guide that was biofunctionalized with growth factors and tested in vitro, as described previously. Three-dimensional motion capture and histological analyses were performed. After recovery periods of three and six months, the rats’ kinematics were analyzed during an over-ground walking task to evaluate the nerve recovery progress. The functional nerve recovery showed significant differences between the operated and non-operated sides of the rat, but no differences in their walking patterns during the recovery period. The histological analysis showed the reorganization of nerve tissue into the nerve as well as the presence of multiple neuronal extensions. Here, we have shown histological improvement in the absence of functional restoration after sciatic nerve transection and reconstruction at six months.
Introduction
Locomotion and posture are based on muscular actions that are controlled by the nervous system (Canu et al. Citation2005). Once the peripheral nervous system (PNS) is damaged, the control of the movement is affected and injuries, e.g. nerve liaison, result in a loss of motor function (Nakamura et al. Citation1996; Bareyre et al. Citation2009). Sciatic nerve injuries may cause long-term health problems with restricted activity and life-long disabilities (Campbell Citation2008).
Unfortunately, the repair of peripheral nerve lesions, especially after the loss of a full segment of the nerve, remains a challenge in reconstructive surgery (Biazar, Khorasani, Montazeri et al. Citation2010; Biazar, Khorasani, Zaeifi Citation2010). When a peripheral nerve is sectioned, the axonal segment distal to the lesion site undergoes Wallerian degeneration (Waller Citation1850; Stoll et al. Citation2002), while the proximal segment is able to regenerate axonal sprouts that can eventually re-establish motor and sensory nerve function. Improvements in nerve injury treatments have been reported, and the treatments range from drug-induced therapies to biological tissue engineering (Rajaram et al. Citation2012). One of the major challenges in regenerative neurobiology is to design suitable biomimetic nerve guides and multiple techniques have been developed to design fibrous scaffolds as tissue substitutes (Marquardt & Sakiyama-Elbert Citation2013).
In recent years, we have witnessed the development of increasingly sophisticated bioengineered nerve graft substitutes composed of synthetic or natural polymer-based nerve guides. Silk is a well-known material used by physicians as sutures for decades (Biazar et al. Citation2010). The sericin-free silk fibroin fiber exhibits excellent biocompatibility both in vitro and in vivo (Campbell Citation2008). Electrospinning has emerged as a useful technique due to the ability to generate fibers similar to the scale of fibrous structures seen in the native extracellular matrix (Lewin Citation2006). Silk fibroin electrospun nanofibers have shown good biocompatibility and circularity to enhance peripheral nerve regeneration (Dinis, Elia, Vidal et al. Citation2014; Duval et al. Citation2014). Different types of bioactive molecules, such as growth factors and adhesive molecules, have been incorporated into scaffolds of electrospun nanofibers to promote peripheral nerve regeneration (Altman et al. Citation2003). Using the electrospinning technique, we developed biofunctional silk nanofibers with Nerve Growth Factor (NGF) and Ciliary NeuroTrophic Factor (CNTF) to stimulate nerve outgrowth by artificial nerve grafting (Dinis, Elia, Vidal et al. Citation2014). In the case of artificial nerve replacements, the variety of surrogates is wide and the histological results are not consistent. A variety of regenerative solutions has been proposed over the last decade; however, the efficiency of the treatment is usually shown histologically and rarely combined with measurements of motor functional.
Objectively quantifying motor functionality through measurement of locomotion is common and well-documented in human and animal research areas (Karakostas & Granholm Citation2014). Locomotion is generally analyzed using temporal–spatial parameters and joint angles to evaluate pathological gait (Marin et al. Citation2003). Gait patterns are analyzed by normalizing and averaging data from a number of isolated and independent strides (Pereira et al. Citation2006). In rat models, both over ground (Kunkel-Bagden et al. Citation1993; Kloos et al. Citation2005) and treadmill walking (Bouët et al. Citation2004) have been studied to analyze hindlimb joint kinematics, temporal and spatial measurements (Pereira et al. Citation2006), inverse dynamics (Webb et al. Citation2011; Johnson et al. Citation2012) and more currently pressure distribution of the footprints (Mendes et al. Citation2015).
Most studies in rodent locomotion after spinal cord injury have used 3D motion capture (Madete et al. Citation2010; Karakostas & Granholm Citation2014) but implementing biomechanical models that take 3D angular displacements of each body segment (Karakostas & Granholm Citation2014) into account are rare. Due to the size of the rodent, the extracted kinematic information is often motion in only two of the three planes of movement (Thota et al. Citation2005). A solution could be either small marker clusters or inertia-based measurement units (Venkatraman et al. Citation2010) to objectively quantify the kinematics of rodents during rehabilitation (Taborri et al. Citation2016).
We developed a new multifunctional silk-based material to support and promote PNS nerve regeneration in a rat model which has already satisfied in vitro biocompatibility test. The aim of this study is to focus on the demonstration of the nerve tissue in vivo regeneration after a nerve injury on an animal model by motor functionality tests of analysis of gait patterns and histological observations. We hypothesize differences in the control of posture and locomotion according to control group and healing time.
Material and method
Animals
Fourteen two month-old Sprague Dawley male rats (Harlan, Compiègne, France) weighing approximately 200–250 g were used in this study. All animals were kept in ventilated, humidity and temperature-controlled rooms with a 12/12-h light/dark cycle. They were housed on sawdust and received food pellets and water ad lib. All procedures were performed with the approval of the Veterinary Authorities of France in accordance with the European Communities Council Directive of 22 September 2010:63/UE. This study was specifically approved by the Université de Technology de Compiègne ethics committee. The animals were observed and examined daily by a veterinarian to avoid unnecessary suffering. The animals were divided into two groups, one control group (six rats) and one group with a NGF/CNTF-functionalized silk implant as nerve prosthesis (eight rats). At the end of the experiments, the animals were euthanized by injection of a lethal dose of sodium pentobarbital. The nerves were surgically dissected immediately after the animal’s death and immersed in 10% buffered formalin awaiting histology.
Silk implant
The full design procedure of NGF/CNTF-functionalized silk implant, including electrospinning, was recently presented by Denis et al. (for detailed information see (Dinis, Vidal, José et al. Citation2014)). Briefly, silk fibroin solution (functionalized) was delivered through a 16G stainless-steel capillary at a constant flow rate of 15 μL/min with a syringe pump (Thermo Scientific, Waltham, MA, USA). The syringe needle was set at 12 kV voltage with a high voltage power supply (Gamma High Voltage Research ES-30P, Ormond Beach, FL, USA) and mounted at the center of a 10 cm diameter aluminum plate. The distance between the needle and the ground collector was set to 15 cm. Fibroin functionalized silk was spun during 90 min on a rotating mandrel (rotation speed: 9.5 m/s). After 90 min of electrospinning, the electrospun fibers were removed from the mandrel and rolled up at 360° about 50 times using Teflon sticks (0.3 mm diameter) in order to get a final tube of about 3 mm diameter. All of the electrospun fibroin with sticks were treated by water vapor annealing overnight to obtain a water insoluble silk conduit (Liu et al. Citation2012). Teflon sticks were removed after the water vapor annealing process using sterile PBS solution. All tubes were standardized at a length of 1 cm with 1.1 mm average diameter in accordance with the size of a rat sciatic nerve (Dinis, Elia, Dermingy et al. Citation2014).
Surgical procedure
In this experiment, we examined a sciatic nerve injury/repair model. Specifically, the NGF/CNTF-functionalized silk implant was wetted overnight at 4 °C in sterile PBS. The animals were deeply anesthetized with a combination of ketamine (60 mg/kg) and xylazin (6 mg/kg). Surgery was performed on the rat’s right leg under aseptic condition and with the aid of an operating microscope (Stemi SV8, Zeiss). A 5 mm defect in the sciatic nerve created by surgical removal of the nerve tissue with micro scissors after local application of lidocain (2 mg/mL) was repaired with the nerve conduits (Figure (A)). The NGF/CNTF-functionalized silk implant was interposed into the 5 mm nerve defects. The distal and proximal nerve ends were then anchored to the conduit by 10-0 nylon micro sutures (Ethicon, Cincinnati, Ohio, USA) (Figure (D)). The skin was sutured with absorbable 4-0 nylon sutures (Ethicon, Cincinnati, Ohio, USA). Postoperative care included intraperitoneal injection of xylazin (10 mg/kg) once a day for up to three days. Animals were weighed daily for the first seven days post-surgery and then weighed weekly to document the postoperative recovery.
Table 1. Mean and standard deviation of the range of motion of the hip, knee, and ankle joints.
Table 2. Mean and standard deviation of the ankle-hip, ankle-knee, and knee-hip cyclogram perimeters.
Motion capture
To evaluate the nerve regeneration of the animal, motion analysis was performed after a recovery period of three and six months postoperatively. The operated rats underwent surgery at two months and the motion capture was conducted at five and eight months (three and six months post-operatively). The control group was examined two months after the experiment commenced at time of the motion capture session for the implant group. The operated rats were weighed weekly showing an increase from 221 ± 15 to 458 ± 43 g. Two weeks prior to the data collection, each animal was trained weekly to walk consistently on a wooden runway (150 × 10 × 1 cm) (Madete et al. Citation2010) at its usual walking speed. Ten minute-long training sessions were given once a week with nutrition used as positive reinforcement to improve performance. This training procedure was achieved on the control and the implant group. Over ground walking was chosen to study natural and spontaneous walking patterns without imposing speed constraints and inflicting possible pain for the rats. For the motion capture session, a seventeen camera motion capture system (T160, Vicon motion systems Inc., Oxford, UK) was used to record the locomotion of the rat at a rate of 100 Hz. Hair was clipped around the hindlimbs to improve the marker placement for analysis. The same operator performed all marker placements to avoid inter-tester variability. Rats were equipped with 10 hemispheric markers (Ø = 3 mm) covered by adhesive infrared-reflective paper (3 M Scotchlite, Minnesota, USA) according to the following anatomic landmarks: fifth lumbar vertebra, the tail origin, the trochanter major, (hip), the knee, the lateral malleolus of the ankle and fifth metatarsophalangeal joint (MTP) (Figure (a) and (b)). The back is defined by the 3D marker positions of the fifth lumbar vertebra and the tail origin and the thigh is defined by the trochanter major and the knee. The shank is defined by the 3D marker positions of the knee and the lateral malleolus while the foot is defined by the lateral malleolus of the ankle and the fifth metatarsophalangeal joint (Figure ). The global reference frame was chosen such that the x axis pointed in the anterior direction, the y axis in the upward direction, and the z axis laterally to the right. The 3D positions of theses markers were recorded when the animal walked on the wooden runway (Figure (c) and (d)) that allowed the recording of 10 consecutive steps at regular speed. The analysis was performed on at least 10 steps per rat. As post processing, the stride length, foot clearance, walking speed (König et al. Citation2014), flexion extension angles of the hip (α), knee (β), and ankle (γ) (Figure (a)) (Canu et al. Citation2005; Perrot et al. Citation2009, Citation2011); and cyclograms (Pereira et al. Citation2006; Kutilek et al. Citation2013) were computed with a custom written Matlab’s routine (MathWorks, Inc., Natick, MA). The hip, knee, and ankle angles were defined as relative angles between the particular segments. The stride length was defined as the distance between the fifth metatarsal head markers of the hindlimb in two consecutive steps and normalized to body height. Stride clearance was defined as the maximal height of the fifth metatarsal head marker of the hindlimb during each step and normalized to body height. Walking speed was defined as step length divided by the time of each step. This equals to an instantaneous walking speed which was then averaged for each group. Based on the joint angles, the range of motion was calculated for each joint as the maximum value between the flexed and the extended position during the walking trials. Cyclograms were generated to inspect the interjoint coordination (Pereira et al. Citation2006). The cyclogram perimeters were calculated as the sum of the length of two successive connecting points of the cyclogram.
Figure 1. In vivo implantation of the NGF/CNTF-functionalized silk implant for sciatic nerve reconstruction. A 5 mm sciatic nerve section is removed on the right leg of the rat (a), the implant is first sutured to the proximal end of the nerve (b), the scaffold is carefully placed in front of the distal extremity of the sectioned sciatic nerve (c) and then sutured without turning the nerve (d).
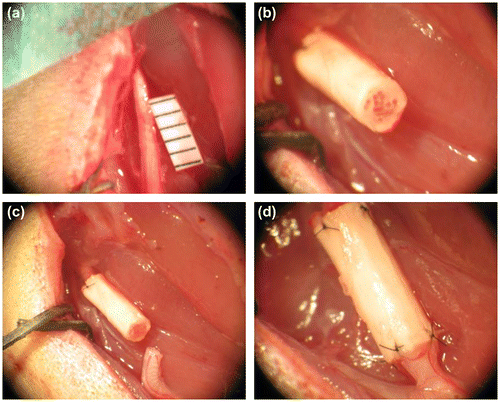
Figure 2. Diagram (a) and picture (b) showing the position of joint markers during the experiment. Ten 3 mm diameter reflective markers positioned on the 5th lumbar vertebra, the tail origin, the trochanter major, (hip), the knee, the lateral malleolus of the ankle and fifth metatarsophalangeal joint (MTP) were used to identify the rat’s right hindlimbs to calculate the hip (α), knee (β) and ankle angle (γ) (c) 3D positions of the cameras and the rat in the motion capture environment (d) superposition of the 3d marker positions and the optical cameras during the motion capture sessions.
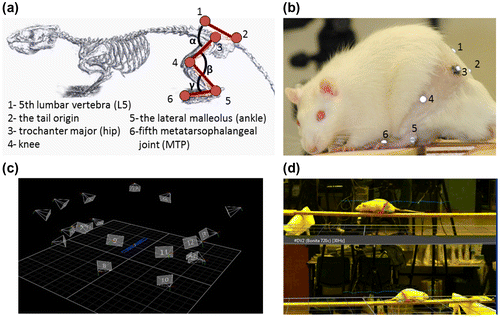
Histological analysis
Once the motion capture sessions were achieved (six months after grafting), histology was performed to observe the nerve regeneration inside the implants. The implants were dissected from the animals, including the surrounding soft tissue, and fixed in 10% buffered formalin solution for two days. Each dissected implant was embedded in a paraffin block and sectioned into 5 μm-thick slices with a microtome (Lemardeley, Paris, France). Then, the sections from the middle of the implant were stained with Hematoxylin and Eosin (H&E) and visualized with a microscope (Leica Microsystems, Jena, Germany). This was done for six samples per implant in an attempt to understand what cellular events may be occurring within and around the tubes in vivo.
Statistical analysis
For statistical analysis, an univariate measures analysis of variance (ANOVA) and Student t-tests were used to compare the differences in the temporal/spatial and kinematic parameters (Mean ± standard deviation (S.D.) values for all of the measured variables are reported) with the STATISTICA software package (StatSoft, Inc. Maisons-Alfort, France). This analysis was followed by Tukey HSD post hoc comparisons (Moore & McCabe Citation2006). The level of statistical significance was a p < 0.05.
Results
Functional results
All eight operated rats were able to perform the motion capture sessions, and the kinematics of hind limbs during the walking task were recorded at three months and six months after surgery. Even though the operated group had a slightly asymmetrical walking pattern, the animals demonstrated well-coordinated and reproducible walking sequences during the experiment without any sign of discomfort or motor impairments.
For walking speed, significant differences between both sessions and the control group (p < 0.05) were seen (Figure (a)). Differences between the operated and non-operated side were shown for the stride length and clearance (p < 0.05), except during the six-month session for clearance (t = 2.2; p > 0.05) (Figure (b)). The average stride length of the walking trials did not differ during the two experimental sessions (p > 0.05), but significant differences between both sessions and the control group (p < 0.05) were seen (Figure (c)). For the range of motion parameters, an ANOVA combining three groups (three months group vs. six months group vs. Control group) on two sides (operated vs. non-operated) for the three joints (Hip vs. Knee vs. Ankle) was performed.
Figure 3. Representative walking speed (a), stride length (b), and stride clearance (c) during walking for the operated (o) and the non-operated limbs (no).
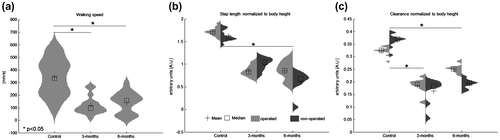
The average range of motion values of the walking trials did not differ during the two experimental sessions, nor did they differ from the control group (F(2,14) = 2.87, p > 0.05). Differences were found between the operated and non-operated side (F(1,7) = 16.09, p < 0.05), as well as among the range of motion of the angles (F(2,14) = 176.31, p < 0.05). Post hoc comparisons only unveiled statistically significant differences (p < 0.05) for the ankle joints between the operated and the non-operated side (see Table ).
For the inter-joint coordination parameter (cyclogram), an ANOVA combining three groups (three months group vs. six months group vs. control) on the two sides (operated vs. non-operated) and three cyclograms (hip-knee vs. hip-ankle vs. knee-ankle) was then applied on the perimeter of the cylcograms. The averaged cyclogram perimeter of the walking trials neither differed among the three-month and six-month groups and the control group (F(1,7) = 0.09, p > 0.05), nor between the operated and non-operated side (F(2.14) = 0.87, p > 0.05) (see Table ).
Histological results
Regeneration from silk-based conduit grafts was confirmed by surgical analysis after six months (Figure ). The morphology of the rat paw was compared with the control group. Paws with the nerve implant were identical, presenting only slightly less separated toes (Figure (a) and (b)). After six months, the nerves regenerated through the native nerve or silk conduit were visualized and prepared for histological assessment (Figure (c) and (d)). The observation of the grafted material revealed no rupture between the sectioned part of the nerve and the biomaterial. A thin membrane of well-vascularized fibrous tissue grew and covered the implanted artificial nerve grafts. After histological preparation, the organization of nerve tissue into the nerve or the graft nerve was noticed. The nerve was successfully connected and bridged (Figure (a) and (b)). Native nerves presented at least three separated fascicular-like areas, whereas the grafts showed more fascicular-like regions. At a higher resolution examination of these pores, a collagen membrane colonizing the silk fibroin material was detected, as wall fibroblasts-like cells which are also found in native nerves (Figure (c) and (d)). Nerve cells were also observed and identified by H&E staining based on their morphologies (Figure (e) and (f)). Schwann cells and axons surrounded by myelin are present inside the grafts, demonstrating neural regeneration extensions inside the silk conduit grafts. A double staining (axonal specialty stain and a Schwann cell stain), could not be performed as the auto fluorescence of the silk proteins attracts the various secondary antibodies tested. The histological results rely on the observation made by two experienced anatomo-pathologists trained to detect the two types of cells (neurons and Schwann cells) on histological sections. As such the histological observations demonstrated the axonal growth and nerve tissue advancement through the entire conduit and the restoration of the integrity of the nerve structure.
Figure 4. Observation of post-operative rats after 6 months. Evaluation of native sciatic nerve (left) and nerve silk graft (right). (a,b) rat paw pictures, (c,d: scale bar 1 cm) sciatic nerve pictures. No visual differences can be observed between the control and the grafted animal.
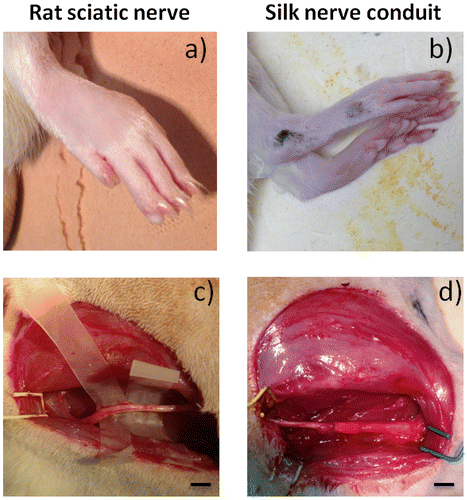
Figure 5. Histological analysis of sciatic nerve (a) and silk conduits (b) following implantation into rats after 6 months. Observation with Hematoxylin and Eosin staining of native sciatic nerve (left) and nerve silk graft (right) by optic microscopy. (a,b, scale bar 150 μm) Overall observation of the full section of the reconstructed nerves. (c, d, scale bar 20 μm) Note inside reconstructed sciatic nerve the presence of blood vessels (circled). (e, f, scale bar 5 μm) Specific cell populations can be observed inside the nerves, specifically Schwann cells (Sc) and axons surrounded by myelin (Ax).
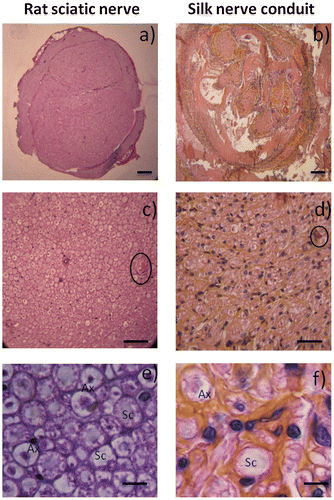
Discussion
This work has demonstrated in vivo nerve regeneration by NGF/CNTF-functionalized silk implant not only from a structural point of view with histological observation, but also from a functional point of view with a locomotion motion analysis. The examinations at three and six months showed contrasting results. First, the locomotion motion analysis showed that the group with implants never achieved walking patterns identical to those of the control group. Moreover, there were no significant differences between results at three months and six months postoperatively, suggesting that the healing process had plateaued after three months. Conversely, histological observations showed the presence of nerve cells which demonstrates that our silk artificial substitutes graft facilitates nerve tissue regeneration.
Regarding the influence of the healing time, our experimental outcomes do not demonstrate a significant change in kinematic parameters or differences in the angular variability of the joints during the walking experiments for the group with implants. The ankle ROM data showed differences between the operated and the non-operated side and rats showed larger plantar flexion during the swing phase of the stride. Thus, the increased ROM may be the result of a motor control deficit of the ankle, and the decreased ROM of the hip could be an overcompensation to avoid limping.
With human experimentation, three-dimensional kinematic gait analysis is used as a performance indicator of a deficit or a rehabilitation process of the neuro-musculoskeletal system (Rezgui et al. Citation2013). In this study, we performed an investigation with rats, and it is well known that motion capture on animals with neuromuscular deficits is difficult (Johnson et al. Citation2012). Global motor control strategies are known to have large individual differences (Latash et al. Citation2002); rats may heal differently and therefore various sensori-motor strategies can exist among different rats (Garnier et al. Citation2008). In our case, we also clearly observed a compensation strategy with unconventional walking patterns. Nevertheless, the evaluation of rat locomotion by a 3D motion analysis system has been proven to provide maximal precision and accuracy of joint angles (Karakostas & Granholm Citation2014), and our results on the control group are in accordance with the literature (Garnier et al. Citation2008; Perrot et al. Citation2011). Longer follow-up may show different results, and it cannot be ruled out that after six months further functional improvement is seen; however, these data suggest that the regenerative nerve did not reach the control group from a functional point of view in the period of this study.
Conversely, the histological observations are very positive from a cellular point of view. Six months after the implantation, the silk nerve conduit was stable- an important factor for axon regeneration and growth. The nerve bundles surrounded by the myelin sheath are immersed in a matrix composed of capillaries and connective tissue. Furthermore, Schwann cells were observed around the axons forming dark spots. These cells wrap around the axon and were found in all parts of the PNS. These microscopic findings were confirmed with H&E staining examination. The entire nerve graft was completely colonized by extensions of neurons and glial cells. These observations confirm previous research showing Fibroin silk as a promising biomaterial to design nerve prosthesis due to its biocompatibility, ability to hold sutures, interesting mechanical properties, and ability to biodegrade into naturally metabolized products (Dinis et al. Citation2013, Dinis, Elia, Vidal et al. Citation2014; Belanger et al. Citation2016).
In addition, some studies reported that functionalized biomaterials with growth factors enhance neurite extension due to migration and growth of Schwann cells on the biomaterials (Fine et al. Citation2002; Ahmed et al. Citation2003; Bryan et al. Citation2004). However, our observations at six months showed an initiation of nerve recovery inside the NGF/CNTF-functionalized silk implant. NGF is well known to stimulate sensory neurons, but its action against motor neuron is unknown (Elliott & Snider Citation1996). This may explain the lack of functional recovery that was seen in the motion capture trials. Functional recovery is directly related to the histological results and six months recovery period may have been too short to fully regain functional movement patterns.
The present study has some limitations and an explanation of the insignificant difference between the three-month and six-month sessions could be the growth of the rats. A lack of physical activity results in an increase of body weight and an increased fat tissue percentage (Filipe et al. Citation2006). Consequently, these physical modifications may have modified the motor strategies (Garnier et al. Citation2008) and also increased the soft tissue artifacts (Filipe et al. Citation2006). This could account for the modifications in ROM during to the healing process. A general change in kinematics based on body composition and growth remains speculative as the control group was measured only at two months. Not only the confounder of body size/composition with reduced activity but also the six-month follow-up time may have been incomplete to see restoration of function. Also the inter-individual variability could have masked some group level effects which may be present with larger samples, or longer observations. Finally, future research including pre-operatively motion capture sessions, an age-matched control group, a cluster-based marker set with a 6-degrees-of-freedom biomechanical model of the rodent, additional kinetic and electromyographic information would contribute to a better understanding of the rehabilitation process.
Conclusion
This study has demonstrated histological improvement in the absence of functional (gait) restoration three and six months after sciatic transection and repair using an NGF/CNTF-functionalized silk implant conduit scaffold. These results highlight the complex relationship between the nerve regeneration process and motor functional recovery. Future studies will focus on how to tune the function of the silk implant to target motor neuron, as well as how the rehabilitation will influence motor functionality, and examine these parameters over longer time periods.
Disclosure statement
No potential conflict of interest was reported by the authors.
Funding
This work was supported by Region Picardie; The Tissue Engineering Resource Center (TERC) from Tufts University (NIH Center – [P41 EB002520]).
Acknowledgments
The authors thank the Picardie Region for its financial support, the Tissue Engineering Resource Center (TERC) from Tufts University (NIH Center – P41 EB002520). Tony M. Dinis received a fellowship from the French Ministry of Science and Technology.
References
- Ahmed Z, Underwood S, Brown RA. 2003. Nerve guide material made from fibronectin: assessment of in vitro properties. Tissue Eng. 9:219–231. doi:10.1089/107632703764664693
- Altman GH, Diaz F, Jakuba C, Calabro T, Horan RL, Chen J, Lu H, Richmond J, Kaplan DL. 2003. Silk-based biomaterials. Biomaterials. 24:401–416.10.1016/S0142-9612(02)00353-8
- Bareyre FM, Kerschensteiner M, Raineteau O, Mettenleiter TC, Weinmann O, Schwalb ME. 2009. The injured spinal cord spontaneously forms a new intraspinal circuit in adult rats. Nat Neurosci. 7:269–277.
- Belanger K, Dinis TM, Taourirt S, Vidal G, Kaplan DL, Egles C. 2016. Recent strategies in tissue engineering for guided peripheral nerve regeneration. Macromol Biosci. 16:472–481. doi:10.1002/mabi.201500367
- Biazar E, Khorasani MT, Montazeri N, Pourshamsian K, Daliri M, Rezaei R, Jabarvand M, Khoshzaban A, Heidari S, Jafarpour M, Roviemiab Z. 2010. Types of neural guides and using nanotechnology for peripheral nerve reconstruction. Int J Nanomed. 5:839–852. doi:10.2147/IJN.S11883
- Biazar E, Khorasani MT, Zaeifi D. 2010. Nanotechnology for peripheral nerve regeneration. Int J Nano Dimens. 1:1–23.
- Bouët V, Borel L, Harlay F, Gahéry Y, Lacour M. 2004. Kinematics of treadmill locomotion in rats conceived, born, and reared in a hypergravity field (2 g). Adaptation to 1 g. Behav Brain Res. 150:207–216.10.1016/S0166-4328(03)00258-4
- Bryan DJ, Tang JB, Doherty SA, Hile DD, Trantolo DJ, Wise DL, Summerhayes IC. 2004. Enhanced peripheral nerve regeneration through a poled bioresorbable poly(lactic-co-glycolic acid) guidance channel. J Neural Eng. 1:91–98. doi:10.1088/1741-2560/1/2/004
- Campbell WW. 2008. Evaluation and management of peripheral nerve injury. Clin Neurophysiol. 119:1951–1965. doi:10.1016/j.clinph.2008.03.018
- Canu M-H, Garnier C, Lepoutre F-X, Falempin M. 2005. A 3D analysis of hindlimb motion during treadmill locomotion in rats after a 14-day episode of simulated microgravity. Behav Brain Res. 157:309–321.10.1016/j.bbr.2004.07.009
- Dinis T, Vidal G, Marin F, Kaplan D, Eglès C. 2013. Silk nerve: bioactive implant for peripheral nerve regeneration. Comput Methods Biomech Biomed Engin. 16:253–254.
- Dinis T, Elia R, Vidal G, Dermingy Q, Denoeud C, Kaplan D, Egles C, Marin F. 2014. 3D multi-channel bi-functionalized silk electrospun conduits for peripheral nerve regeneration. J Mech Behav Biomed Mater (accepted).
- Dinis TM, Elia R, Vidal G, Auffret A, Kaplan DL, Egles C. 2014. Method to form a fiber/growth factor dual-gradient along electrospun silk for nerve regeneration. J Mech Behav Biomed Mater. 41:43–55. doi:10.1016/j.jmbbm.2014.09.029
- Dinis T, Vidal G, José R, Fitzpatrick C, Marin F. 2014. Complementary effects of two growth factors in multifunctionalized silk nanofibers for nerve reconstruction. PlosOne. 9:e109770. doi:10.1371/journal.pone.0109770
- Duval J-L, Dinis T, Vidal G, Vigneron P, Kaplan DL, Egles C. 2014. Organotypic culture to assess cell adhesion, growth and alignment of different organs on silk fibroin. J Tissue Eng Regen Med. doi:10.1002/term.1916
- Elliott JL, Snider WD. 1996. Motor neuron growth factors. Neurology. 47:47S–53S. doi:10.1212/WNL.47.4_Suppl_2.47S
- Filipe VM, Pereira JE, Costa LM, Maurício AC, Couto PA, Melo-Pinto P, Varejão ASP. 2006. Effect of skin movement on the analysis of hindlimb kinematics during treadmill locomotion in rats. J Neurosci Methods. 153:55–61.10.1016/j.jneumeth.2005.10.006
- Fine EG, Decosterd I, Papaloïzos M, Zurn AD, Aebischer P. 2002. GDNF and NGF released by synthetic guidance channels support sciatic nerve regeneration across a long gap. Eur J Neurosci. 15:589–601. doi:10.1046/j.1460-9568.2002.01892.x
- Garnier C, Falempin M, Canu M-H. 2008. A 3D analysis of fore- and hindlimb motion during locomotion: comparison of overground and ladder walking in rats. Behav Brain Res. 186:57–65.10.1016/j.bbr.2007.07.023
- Johnson WL, Jindrich DL, Roy RR, Edgerton VR. 2012. Quantitative metrics of spinal cord injury recovery in the rat using motion capture, electromyography and ground reaction force measurement. J Neurosci Methods. 206:65–72.10.1016/j.jneumeth.2012.02.008
- Karakostas T, Granholm A-C. 2014. Motion capture and associated novel measurement devices for movement function in humans and animal models. J Neurosci Methods. 231:1–2.10.1016/j.jneumeth.2014.05.028
- Kloos AD, Fisher LC, Detloff MR, Hassenzahl DL, Basso DM. 2005. Stepwise motor and all-or-none sensory recovery is associated with nonlinear sparing after incremental spinal cord injury in rats. Exp Neurol. 191:251–265.10.1016/j.expneurol.2004.09.016
- König N, Singh NB, von Beckerath J, Janke L, Taylor WR. 2014. Is gait variability reliable? An assessment of spatio-temporal parameters of gait variability during continuous overground walking. Gait Posture. 39:615–617.10.1016/j.gaitpost.2013.06.014
- Kunkel-Bagden E, Dai HN, Bregman BS. 1993. Methods to assess the development and recovery of locomotor function after spinal cord injury in rats. Exp Neurol. 119:153–164.10.1006/exnr.1993.1017
- Kutilek P, Viteckova S, Svoboda Z, Smrcka P. 2013. Kinematic quantification of gait asymmetry in patients with peroneal nerve palsy based on bilateral cyclograms. J Musculoskelet Neuronal Interact. 13:244–250.
- Latash ML, Scholz JP, Schöner G. 2002. Motor control strategies revealed in the structure of motor variability. Exerc Sport Sci Rev. 30:26–31.10.1097/00003677-200201000-00006
- Lewin M. 2006. Handbook of fiber chemistry. 3rd ed. Boca Raton, FL: CRC Press.
- Liu W, Thomopoulos S, Xia Y. 2012. Electrospun nanofibers for regenerative medicine. Adv Healthc Mater. 1:10–25. doi:10.1002/adhm.201100021
- Madete JK, Klein A, Fuller A, Trueman RC, Rosser AE, Dunnett SB, Holt CA. 2010. Challenges facing quantification of rat locomotion along beams of varying widths. Proc Inst Mech Eng [H]. 224:1257–1265.10.1243/09544119JEIM779
- Marin F, Allain J, Diop A, Maurel N, Simondi M, Lavaste F. 2003. On the estimation of knee joint kinematics. Hum Mov Sci. 18:613–622.
- Marquardt LM, Sakiyama-Elbert SE. 2013. Engineering peripheral nerve repair. Curr Opin Biotechnol. 24:887–892.10.1016/j.copbio.2013.05.006
- Mendes CS, Bartos I, Márka Z, Akay T, Márka S, Mann RS. 2015. Quantification of gait parameters in freely walking rodents. BMC Biol. 13:50. doi:10.1186/s12915-015-0154-0
- Moore DS, McCabe G. 2006. Introduction to the practice of statistics. New York, NY: W. H. Freeman and Company.
- Nakamura M, Fujimura Y, Yato Y, Watanbe M. 1996. Muscle reorganization following incomplete cervical spinal cord injury in rats. Spinal Cord. 34:752–756.
- Pereira JE, Cabrita AM, Filipe VM, Bulas-Cruz J, Couto PA, Melo-Pinto P, Costac LM, Geunad S, Maurícioe AC, Varejão ASP. 2006. A comparison analysis of hindlimb kinematics during overground and treadmill locomotion in rats. Behav Brain Res. 172:212–218.10.1016/j.bbr.2006.04.027
- Perrot O, Laroche D, Pozzo T, Marie C. 2009. Quantitative assessment of stereotyped and challenged locomotion after lesion of the striatum: a 3D kinematic study in rats. PLoS One. 4:e7616. doi:10.1371/journal.pone.0007616
- Perrot O, Laroche D, Pozzo T, Marie C. 2011. Kinematics of obstacle clearance in the rat. Behav Brain Res. 224:241–249.10.1016/j.bbr.2011.05.027
- Rajaram A, Chen X-B, Schreyer DJ. 2012. Strategic design and recent fabrication techniques for bioengineered tissue scaffolds to improve peripheral nerve regeneration. Tissue Eng Part B Rev. 18:454–467.10.1089/ten.teb.2012.0006
- Rezgui T, Megrot F, Marin F. 2013. Musculoskeletal modelling of cerebral palsy children: sensitivity analysis of musculoskeletal model parameter’s values for gait analysis. Comput Methods Biomech Biomed Engin. 16:155–157.10.1080/10255842.2013.815897
- Stoll G, Jander S, Myers RR. 2002. Degeneration and regeneration of the peripheral nervous system: From Augustus Waller’s observations to neuroinflammation. J Peripher Nerv Syst. 7:13–27.10.1046/j.1529-8027.2002.02002.x
- Taborri J, Palermo E, Rossi S, Cappa P. 2016. Gait partitioning methods: a systematic review. Sensors. 16:66. doi:10.3390/s16010066
- Thota AK, Watson SC, Knapp E, Thompson B, Jung R. 2005. Neuromechanical control of locomotion in the rat. J Neurotrauma. 22:442–465. doi:10.1089/neu.2005.22.442
- Venkatraman S, Jin X, Costa RM, Carmena JM. 2010. Investigating neural correlates of behavior in freely behaving rodents using inertial sensors. J Neurophysiol. 104:569–575. doi:10.1152/jn.00121.2010
- Waller A. 1850. Experiments on the section of the glossopharyngeal and hypoglossal nerves of the frog, and observations of the alterations produced thereby in the structure of their primitive fibres. Philos Trans R Soc Lond. 140:423–429.10.1098/rstl.1850.0021
- Webb AA, Kerr B, Neville T, Ngan S, Assem H. 2011. Kinematics and ground reaction force determination: a demonstration quantifying locomotor abilities of young adult, middle-aged, and geriatric rats. J Vis Exp. 48:2138. doi:10.3791/2138