ABSTRACT
This review focuses on the properties of the light fields that are more useful in applications. We review recent means of generating shaped-pulse light field, by which matters can be steered toward the desired products, thereby allowing the coherent control in terms of effectiveness, selectivity and manipulation. Applications of these light fields are discussed, including bioscience, laser machining, novel material fabrication, trace material detection and military.
Graphical Abstract
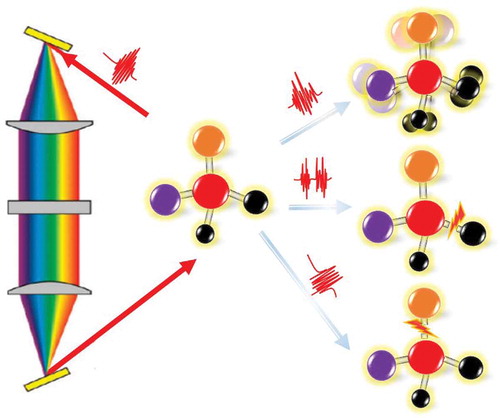
1. Introduction
Because of the complex molecular level structures, molecule reactions can have multiple pathways and multiple sets of products. We need some of the products, but we do not want other byproducts. Controlling the outcome of chemical reactions has been a long-lasting goal of scientists. Conventional methods change the yield of products statistically, for example, by adjusting the temperature, pressure and concentration of reactants. The addition of the catalyst microscopically intervenes the reaction process. With the advent of the laser, people started to use laser light to control molecule reactions. By simply changing the intensity, wavelength, duration of the laser, a certain degree of controllability and selectivity can be achieved. For example, by selectively exciting the O-H bond vibration in the HOD molecule, the OD+H2 product channel can be selected over the OH+HD channel in the H+ HOD reaction [Citation1]. However, for larger molecules, because of intramolecular vibrational redistribution (IVR), the energy pumped into one molecule bond may soon be distributed into several bonds in the molecule, making it difficult to control the product distribution. The problem can be circumvented by utilizing the coherence property of laser light interaction with matter.
In 1986, Brumer and Shapiro proposed the phase coherent control theory [Citation2]. In this method, a molecule is excited simultaneously via two pathways from an initial state to a common excited state. The transition amplitudes induced by the light waves interfere with one another so that variation of the relative phase of these probability amplitudes modulates the excited state’s population. The control method was firstly used by Gordon et al. on HCl molecule [Citation3]. Then it has been successfully demonstrated on many molecule systems, for example, selectivity between molecule ionization and dissociation [Citation4] or different dissociation channels [Citation5]. In the time domain approach, a pump pulse and a dump pulse are used. When the wave packet produced by the pump pulse moves to a certain point on the potential energy surface, a dump pulse (or probe pulse) is applied, and desired products are obtained. The method was also successfully used on many molecule systems [Citation6,Citation7]. The phase between the double-pulse can also be locked to control the molecule dynamics [Citation8,Citation9]. The methods mentioned above are so-called single parameter methods, which means that only one parameter (the phase between two-colored lasers or the delay between double pulses) can be adjusted to control the molecule [Citation10].
In 1985, Tannor and Rice proposed theoretically to use a shaped pulse train to control the product distribution of molecule reaction [Citation11,Citation12]. The shaped pulse train is a series of time and phase correlated pulses with time separations in the femtosecond to picosecond range. This method is a multiple parameter control method. One can adjust the number of sub-pulses, the time, intensity, phase, polarization of each sub-pulse to achieve the optimal control of molecule dynamics.
The biggest problem in controlling molecule reactions is that people know little about the energy structure information for larger molecules (more than a few atoms). Furthermore, the potential energy surface will be distorted in a high-intensity laser field, and the energy levels will be broadened. Theoretically, it is difficult to calculate the best pulse shape to control the molecule reaction. In 1992, Rabitz et al. proposed a closed-loop learning (CLL) method to let the experimental system search by itself the best pulse shape [Citation13]. In this method, the experimenter first put a guess pulse shape or even a random pulse shape into the loop. The results from this pulse shape will be analyzed, and a better shape will be proposed. The loop repeats until the best pulse shape is found. The first application of the CLL method in the molecule system was the control of dissociation of CpFe(CO)2Cl [Citation14]. It was successfully used on many other molecule systems [Citation15–17]. Implementation of the CLL method is not limited to the control of gas-phase molecule reactions. It can also be used in the adaptive compression of the femtosecond laser pulse [Citation18], which is the commonly used method in the lab to test the CLL method algorithm. Other uses of the CLL method include the optimization of the excited state population of dye molecule in the solvent [Citation19], the high harmonic generation [Citation20], the double ionization of Ca [Citation21], the control of ionization and dissociation of the cluster [Citation22,Citation23], selective excitation of one of two very close energy levels [Citation24]. Theoretical works have been done to design the control field and algorithm, and analyze the result [Citation25], which has explained theoretically why effective quantum control is not difficult to realize. It was predicted that as long as the control field is versatile enough, it can control complex systems [Citation26]. The CLL method’s advantage is that the experimenter can start the experiment without any prior knowledge of the molecule system studied and let the experimental system find the optimum pulse shape by itself. However, this is also the drawback of this approach, in the sense that while one has achieved control of the molecule, one does not know how it did it. Studies have focused on simplifying the control pulse by removing extraneous structure from it and trying to understand the mechanisms involved corresponding to the optimum pulse shape [Citation27–29].
Speaking of applications, the effective control of the dynamics of molecules in the condensed phase is essential since a lot of molecules that are vital to our daily life are in the condensed phase, for example, those in biological organisms. Molecules in such a complex environment are subject to energy redistribution to the surrounding molecules, posing an additional challenge to coherent control. Nevertheless, successful control has been demonstrated in many such systems [Citation30–34].
This review focuses on controlling matter with light pulses with intensity, phase, polarization variation in femtoseconds to picoseconds time scales. There are other categories of shaped-pulse light fields. For example, spatially manipulated beams may have exotic focus shapes and find their applications [Citation35,Citation36]. Those topics will not be covered. In the second section, we will discuss methods to generate different kinds of light fields. The third section will look into how such light fields manipulate fundamental molecule dynamics. The fourth section will give a few examples in different areas of applications. The last section is for conclusions and prospects. This is not a comprehensive review but rather a summary of the concept of this field. This review is intended to show the potential of the shaped-pulse light field as a tool and promote applications that may come into reality soon.
2. Generation of shaped-pulse light field
2.1. Evolution of pulse shaping technique
The first step towards controlling molecular reactions is to generate the tool needed, i.e. the shaped femtosecond laser pulse train. In earlier times, dye pulses have been elongated through a fiber. The long pulse can be modulated in the time domain and then recompressed into a shaped femtosecond pulse [Citation37]. Most current works use a spatial light modulator at the Fourier plane (frequency plane) and construct a shaped pulse by an inverse Fourier transformation [Citation38]. As shown in , the optical setup consists of two gratings, two lenses, and the spatial light modulator, which is sometimes called a 4 f setup because the optical path is four times the lens’s focal length.
Figure 1. (a) the conventional pulse-shaping apparatus using SLM, (b) the pulse-shaping apparatus using acousto-optic modulators (Ref. 39) [Citation45]
![Figure 1. (a) the conventional pulse-shaping apparatus using SLM, (b) the pulse-shaping apparatus using acousto-optic modulators (Ref. 39) [Citation45]](/cms/asset/e10dd140-b10a-4225-907f-85038818fa40/tapx_a_1949390_f0001_oc.jpg)
In earlier times, spatial light modulators were substrates with fixed patterns. For example, fused silica etched to a certain depth has been used at the Fourier plane to alter the phase. The amplitude was modulated by metal films evaporated on the substrate [Citation39]. The modulation of amplitude can only be 0 or 1. This was the first pulse shaping realized in the femtosecond time regime. In 1990, Weiner et al. used a spatial light modulator with 128 liquid crystal pixels [Citation40,Citation41]. Each pixel in a liquid crystal spatial light modulator is individually changeable and computer controllable, enabling switching pulse shapes quickly, which is a necessary condition for the closed-loop learning method. To achieve simultaneously the change of the phase and amplitude, two liquid crystal spatial light modulators were used [Citation42,Citation43]. Later, the two spatial light modulators were put together [Citation44], which is the commonly used setup in labs now. They also studied the differences between the 4 f and 8 f setup, the single mask and dual mask, and the inter-pixel gap’s influences. Instead of a liquid crystal modulator, an acousto-optic modulator can be used shown in [Citation45]. Acousto-optic modulators feature some advantages, for example, no inter-pixel gap and hence a smooth modulation curve. A single modulator can modulate phase and amplitude. However, there are also disadvantages, such as the acoustic pattern propagating across the modulator is not fixed. Hence, it is not suitable for high repetition lasers because the pattern will be distorted between pulses. A digital micromirror device can also be used in light modulation [Citation46]. It has many individually controllable tiny mirrors, reflecting light at a particular wavelength or spatial location.
In pulse shaping, we add phase and amplitude modulations to the femtosecond laser pulse, so it is crucial to ensure that the pulse before shaping is transform-limited. Thus, a properly shaped pulse with precisely the same phase as we added can be obtained at the pulse shaper’s output. Many methods exist for measuring the amplitude and phase of a femtosecond laser pulse, for example, the Frequency Resolved Optical Gating (FROG) [Citation47], the Spectral Phase Interferometry for Direct Electric-field Reconstruction (SPIDER) [Citation48]. The pulse shaping and pulse characterization can be combined into one setup using the Multiphoton Intrapulse Interference Phase Scan (MIIPS) method [Citation49]. The method takes advantage of the multiphoton intrapulse interference effect. During a multiphoton process, because the ultrashort excitation pulse has a certain bandwidth, different combinations of photon energies can add up to the same final energy. The final multiphoton excitation probability is determined by the interference of the different excitation pathways. The interference can be constructive or destructive.
The liquid crystal material used in the spatial light modulators cannot withstand high fluence laser irradiation. A pre-amplification pulse shaping scheme can be used to avoid this problem, in which the pulse shaper is put in between the oscillator and the amplifier [Citation50]. Another advantage of this scheme is that the amplifier’s output intensity is not affected by the pulse shaper since the amplifier runs in a saturated regime. A second pulse shaper was put after the amplifier to verify that the accurate phase has been transferred to the output pulse. The technique was also used in pulse shaping using an acousto-optic modulator [Citation51].
2.2. Ultraviolet and other wavelengths
According to the system studied, we may need shaped laser pulses at different wavelengths in our research works. Different kinds of spatial light modulators work in different wavelength regions. Traditional Ti:sapphire laser pulses around 800 nm can be shaped using liquid crystal spatial light modulators, which work best in the visible to near-infrared. Ultraviolet laser pulses can be shaped using acousto-optic modulators [Citation52]. Shaped pulses in the near-infrared can also be converted to the ultraviolet using frequency doubling or other frequency conversion methods [Citation53,Citation54]. However, special care must be taken because the conversion efficiency will be strongly affected by the spectral phase. Thus, the product yield will depend on not only laser-controlled molecule dynamics but also the generated ultraviolet pulse intensity.
Other methods were also used to generate ultraviolet shaped pulse trains. For example, instead of doing the frequency doubling on the already shaped pulse train, the frequency doubling can be carried out at the Fourier plane of the 4 f setup [Citation55]. This way, each frequency component is mixed only with itself, and the problem of crossing the frequency components is eliminated. In Ref [Citation56]., the LC-SLM (liquid crystal spatial modulator) was placed in the chirped pulse amplification system. The shaped spectral phase of Ti:sapphire laser was then transferred to that in a deep UV pulse at 256 nm.
In Ref [Citation57]., an extreme ultraviolet pulse shaper has been built, which uses grazing-incidence reflective optics and operates under ultra-high vacuum, as shown in . The instrument can shape femtosecond pulses with a wavelength as short as 15 nm, and a proof-of-principle experiment with 266 nm laser has been performed. The study overcomes conventional transmissive pulse shapers’ main wavelength obstacles: the limited transparency and laser-induced modulator medium damage at shorter wavelengths.
Figure 2. (a) Sketch of XUV pulse shaper optics in 4 f-geometry (side view) with the cylindrical mirrors CM1 to CM4, the gratings G1 and G2 as well as the shaping mask. A phase/amplitude mask located in the fourier plane of the zero-dispersion compressor allows tailoring the time-frequency spectrum (shape) of femtosecond pulse indicated in purple. A lamellar mirror assembly acting as a phase/amplitude mask and operating under grazing incidence is highlighted. (b) Comparison of the measured UV spectrum averaged over 251 pulses at the shaper exit (orange) with the simulated spectral distribution (blue). (c) part of the lamellar mirror modulating the spectral amplitudes in the fourier plane is shown in a false color representation. The reflective stripes of 100 μm width are separated by 150 μm gaps. surface damage (‘chipping’) is indicated in purple.(Ref. 57) [Citation57]
![Figure 2. (a) Sketch of XUV pulse shaper optics in 4 f-geometry (side view) with the cylindrical mirrors CM1 to CM4, the gratings G1 and G2 as well as the shaping mask. A phase/amplitude mask located in the fourier plane of the zero-dispersion compressor allows tailoring the time-frequency spectrum (shape) of femtosecond pulse indicated in purple. A lamellar mirror assembly acting as a phase/amplitude mask and operating under grazing incidence is highlighted. (b) Comparison of the measured UV spectrum averaged over 251 pulses at the shaper exit (orange) with the simulated spectral distribution (blue). (c) part of the lamellar mirror modulating the spectral amplitudes in the fourier plane is shown in a false color representation. The reflective stripes of 100 μm width are separated by 150 μm gaps. surface damage (‘chipping’) is indicated in purple.(Ref. 57) [Citation57]](/cms/asset/67e40348-1902-4df6-a9ac-99e958f7cacf/tapx_a_1949390_f0002_oc.jpg)
The adiabatic difference frequency generation method was used to convert near-IR pulse to mid-IR pulse [Citation58]. By changing phase functions on the near-IR pulse shaper, a mid-IR pulse pair was generated, and the delay and phase between the pulses can be controlled. The direct amplitude and phase shaping of mid-infrared (MIR) femtosecond laser pulses at 4.9 μm has been reported [Citation59] by using an acousto-optic programmable dispersive filter, as shown in . Calomel crystal extends the device’s wavelength region to mid-infrared. In another work, germanium was used [Citation60]. Terahertz laser pulses can also be shaped, by shaping the femtosecond laser pulse which generates the terahertz pulse, by using shaped-pulse terahertz devices or materials, or by shaping the terahertz pulse directly [Citation61].
Figure 3. (a) Experimental setup of mid-infrared femtosecond pulse shaping with a calomel acousto-optic programmable dispersive filter. (b) Examples of amplitude shaping: spectra of the transmitted MIR pulse with acoustic wave on (dotted curve, Ion) and off (dashed curve, Ioff) and the corresponding relative difference (solid curve, (Ioff − Ion)/Ioff). (c) Examples of phase modulations induced by the acoustooptic programmable dispersive filter (AOPDF). From left to right: quadratic, cubic, and fourth-order applied (dashed lines) and measured (solid lines) phases. phase error ε is displayed for each phase function. the spectra of the diffracted pulses are shown in the background. (Ref.59) [Citation59]
![Figure 3. (a) Experimental setup of mid-infrared femtosecond pulse shaping with a calomel acousto-optic programmable dispersive filter. (b) Examples of amplitude shaping: spectra of the transmitted MIR pulse with acoustic wave on (dotted curve, Ion) and off (dashed curve, Ioff) and the corresponding relative difference (solid curve, (Ioff − Ion)/Ioff). (c) Examples of phase modulations induced by the acoustooptic programmable dispersive filter (AOPDF). From left to right: quadratic, cubic, and fourth-order applied (dashed lines) and measured (solid lines) phases. phase error ε is displayed for each phase function. the spectra of the diffracted pulses are shown in the background. (Ref.59) [Citation59]](/cms/asset/736cb85b-3762-46e5-ad8d-913b6636cffb/tapx_a_1949390_f0003_b.gif)
By shaping the electron bunch, X-ray pulse pairs have been generated, and the pulse separations were controlled on a femtosecond time scale [Citation62]. The shaped X-ray pulses can be characterized by time-resolved photoelectron streaking spectroscopy. X-ray pulse pairs have potential applications in x-ray pump-probe experiments. Deeply bound core states or highly excited conduction band states can be studied.
2.3. Polarization pulse shaping
Most molecules are three-dimensional. During ionization, dissociation, and isomerization processes, the molecular structure changes with time. To achieve better control over the molecular dynamics, a pulse with polarization state changing with time is preferred. A good review detailed the development and application of polarization shaping [Citation63], here we just give a brief introduction. The simplest way of generating polarization-shaped femtosecond pulses is to combine two pulses with different polarization [Citation64,Citation65], or two color field with orthogonal polarization [Citation66,Citation67]. Liquid crystal spatial light modulators are then used to generate polarization-shaped pulses [Citation68,Citation69]. To ensure the control of the phase, the amplitude, and the polarization independently, the optical setup needs to be carefully designed, including the direction of liquid crystal, the number of liquid crystal mask, the position of waveplates and polarizers [Citation70–73], in which a typical strategy using two SLMs (four-layer liquid crystal mask) has been developed. Then, three SLMs can achieve the shaping pulse with arbitrary polarization [Citation72], as shown in .
Figure 4. Pulse shaper setup for full control of phase, amplitude, and polarization of femtosecond laser pulses. left. for the modulation of the pulses, three standard double liquid crystal array modulators, a polarizer, and a pair of half-wave plates are used. they are placed in the fourier plane of a 4-f line in the order shown in the inset on the upper. the inset on the right shows a polarization ellipse with its major (Amajor) and minor (Aminor) axes. the orientation is described by the angle Ref.72) [Citation72]
![Figure 4. Pulse shaper setup for full control of phase, amplitude, and polarization of femtosecond laser pulses. left. for the modulation of the pulses, three standard double liquid crystal array modulators, a polarizer, and a pair of half-wave plates are used. they are placed in the fourier plane of a 4-f line in the order shown in the inset on the upper. the inset on the right shows a polarization ellipse with its major (Amajor) and minor (Aminor) axes. the orientation is described by the angle Ref.72) [Citation72]](/cms/asset/757faf43-ce88-4f7b-a88f-94d8a885a86f/tapx_a_1949390_f0004_oc.jpg)
Recently, the vortex optical field with different structured states of polarization (SoP) has been investigated. The SoP can be manipulated spatially in the cross section of field by changing the orbital angular momentum (OAM) caused by the helical wave front of a light beam. Various vortex fields are generated by imposing the various spatial phase function in a vector vortex optical field [Citation74–76], for example helix phase plate [Citation77]. A novel optical OAM control named ‘mode-splitting’ can produce an arbitrary OAM state with vector polarization [Citation78], as shown in . Furthermore, some advances in the photonic platform have brought in many new phenomena and advantages to manipulate the light fields [Citation79–81].
Figure 5. The mode-splitting concept of optical OAM states, where m and l are the azimuthal index of polarization state and the topological charge of vortex phase, respectively. (b) poincare sphere representation of polarization states for plane waves. the poles represent circular polarization, the equator linear polarization and the intermediate points elliptical polarization. the northern and southern hemispheres stand for the right-handed (RH) and left-handed (LH) elliptical polarization. the polarization states at antipodal points are orthogonal, and any other state of polarization is given as their linear combination. (c) Polarization distribution of three kinds of polarized beams in terms of m = − 1, 0, and 1, respectively. (d) The weight of radial and azimuthal polarization component in the beam cross section of the three beams in (c). (Ref.78) [Citation78]
![Figure 5. The mode-splitting concept of optical OAM states, where m and l are the azimuthal index of polarization state and the topological charge of vortex phase, respectively. (b) poincare sphere representation of polarization states for plane waves. the poles represent circular polarization, the equator linear polarization and the intermediate points elliptical polarization. the northern and southern hemispheres stand for the right-handed (RH) and left-handed (LH) elliptical polarization. the polarization states at antipodal points are orthogonal, and any other state of polarization is given as their linear combination. (c) Polarization distribution of three kinds of polarized beams in terms of m = − 1, 0, and 1, respectively. (d) The weight of radial and azimuthal polarization component in the beam cross section of the three beams in (c). (Ref.78) [Citation78]](/cms/asset/ed669923-e935-4053-b0f5-a6f1183518cf/tapx_a_1949390_f0005_oc.jpg)
2.4. Other techniques
Many other works have been done to improve the quality of generated pulse shapes, overcome current techniques’ limitations, and meet special requirements. The following are just a few examples.
In Ref [Citation82]., the phase instability due to the thermally activated libration of nematic liquid crystal molecules and its effect on the output laser shape are studied. Cooling of the LC-SLM and signal averaging methods are proposed to minimize this effect.
As mentioned before, acousto-optic modulators are not suitable for high repetition lasers. However, some applications, for example, biology imaging with ultrashort laser pulses, need high repetition laser sources. An effective way of pulse selection and calibration has been demonstrated. With an input laser repetition rate of 76 MHz, ~10 MHz repetition rate pulse shaping has been realized [Citation83].
Satellite pulses are common in shaped pulse trains, and they might influence the dynamic behavior of the system we want to control. A randomized multiple independent comb shaping algorithm was developed to generate satellite-free pulse sequences [Citation84]. Compared to periodic transmission comb phases, the aperiodic and random permutated comb phases can suppress undesired satellite pulses by the factor of 8 and extend the usable delay range.
Usually, femtosecond pulse pairs are generated with a Michelson Interferometer setup. However, the achievable delay precision is limited by mechanical stability and travel range adjustment resolution. Femtosecond laser pulse pairs can also be generated by using a pulse shaper. The delay between the sub-pulses can be controlled with zeptosecond precision [Citation85]. The precisely timed pulse pairs were used in the quantum control experiment of potassium.
3. Matter manipulation
In this section, we discuss the benefits we can get when we use a shaped-pulse light field to interact with matter. Shaped femtosecond laser pulses interact with atoms and molecules directly on femtosecond to picosecond time scales, which results in many advantages, such as effective reaction, selective identification, and structure manipulation.
3.1. Effectiveness
By effectiveness, we mean that the shaped pulse can induce an effective reaction (such as higher signal intensity, higher yield of products) than induced by a transform-limited pulse. Effectiveness is the result of the fully matching of the laser field with the microscopic mechanisms, such as the resonance of energy levels, the matching of laser sub-pulse arrival time with wavepacket motion on the potential energy surfaces, the matching of pulse train interval with the coherent motion of the lattice.
For example, compared to the shortest, transform-limited pulse, the D→A fluorescence signal induced by a positively chirped pulse increased by a factor of 3 [Citation86]. By using a pulse train, at some sub-pulse separation, the product yield from the ionization/dissociation of cyclopentanone can be enhanced by seven-folds, compared to transform-limited pulse, and the yield ratios between different products can be changed by up to 18 folds [Citation87]. By simply dividing the laser pulse into two sub-pulses, the laser-induced breakdown spectral emission during ablation can be enhanced by many folds [Citation88]. As shown in , the fluorescence from Si atoms and ions was observed to increase by an order of magnitude as the delay between the pulses was increased [Citation89].
Figure 6. Atomic fluorescence produced by the double pulse ablation of Si<111 > . (a) fluorescence vs delay time at a combined fluence of 48.7 J/cm2. each point is the average of 40 pulses, with each pulse ablating a fresh surface area. The error bars are a single standard deviation. the smooth curve is a least squares fit of an exponential function. (b) enhancement ratio as a function of delay time and fluence. the three-dimensional representation is based on delay scans taken at seven different fluences. (Ref.89) [Citation89]
![Figure 6. Atomic fluorescence produced by the double pulse ablation of Si<111 > . (a) fluorescence vs delay time at a combined fluence of 48.7 J/cm2. each point is the average of 40 pulses, with each pulse ablating a fresh surface area. The error bars are a single standard deviation. the smooth curve is a least squares fit of an exponential function. (b) enhancement ratio as a function of delay time and fluence. the three-dimensional representation is based on delay scans taken at seven different fluences. (Ref.89) [Citation89]](/cms/asset/4eda15e6-47a9-43ee-8cc1-a46e770c8c0f/tapx_a_1949390_f0006_oc.jpg)
In the classical linear regime, the rate of absorption of photons by an atom or molecule is proportional to the number density of the absorbers and the irradiance of the light source. By introducing multiple coherent excitation paths, it is possible to alter the absorption cross-section, thereby creating ‘dark pulses’ of light with the same spectral content as a conventional pulse but are not absorbed by the target species. Induced transparency is achieved over the prespecified period via a coherent mechanism that relies on controlling the wavepacket dynamics in the excited manifold by using the optimally designed laser pulse [Citation90,Citation91]. For example, using shaped femtosecond pulses with a genetic algorithm, the ionization of isolated pyrazine molecules at a prespecified time is suppressed shown in [Citation90]. The induced transparency may find applications where reduced absorption of laser light is required, for example, deep penetration in biological tissues.
Figure 7. Pyrazine parent ion growth curves for the feedback-generated pulse with early target time T. the arrows at t = 1.24 ps indicate the genetic algorithm (GA) target time. (a) comparison of the raw data for the genetic algorithm optimized pulse and a Transform-Limited (TL) pulse measured back-to-back. (b) comparison of the ion growth curve for the feedback-controlled experiment with the solution to the rate equations. (c) comparison of the ion growth curve with the optimized pulse envelope. (Ref.90) [Citation90]
![Figure 7. Pyrazine parent ion growth curves for the feedback-generated pulse with early target time T. the arrows at t = 1.24 ps indicate the genetic algorithm (GA) target time. (a) comparison of the raw data for the genetic algorithm optimized pulse and a Transform-Limited (TL) pulse measured back-to-back. (b) comparison of the ion growth curve for the feedback-controlled experiment with the solution to the rate equations. (c) comparison of the ion growth curve with the optimized pulse envelope. (Ref.90) [Citation90]](/cms/asset/2313a27c-1f69-49cf-9abc-0c8771471ff8/tapx_a_1949390_f0007_oc.jpg)
3.2. Selectivity
Some molecules have similar structures, such as the homologous molecule series and isomers. As an extreme case of isomer, chiral molecules have almost identical structures, except that one is the other’s mirror image [Citation92]. The similarities of these molecules, both in spatial structures and energy level structures, pose significant challenges to scientists in distinguishing them and controlling the reaction between them. In this review, we mainly summarize the selectivities in these area, including identification, isomerization and reaction control. Many research works have been done in this area, showing the selectivity achieved using shaped-pulse light fields.
The selectivity can be implemented by manipulating the rotational processes using shaped-pulse laser fields. A theoretical scheme for enantiomer identification of chiral molecules is proposed using a pair of skewed mutual linearly polarized laser pulses [Citation93], as shown in . The approach can excite a coherent unidirectional rotation of two enantiomers, and show that the off-diagonal anisotropic contributions to the electric polarizability tensor for two enantiomers have different signs. It is very suitable for selective chiral analysis of mixtures. The same optical method for enantiomer identification is presented using the laser fields with twisted polarization [Citation94,Citation95]. Milner and Averbukh first achieve the experimental enantioselective rotational control of chiral molecules with an optical centrifuge [Citation96].
Figure 8. Left: one-dimensional cut through the potential energy surface of HSOH calculated along the torsional coordinate rHSOH together with the definition of the molecule fixed axes system for two enantiomeric molecular configurations. right: calculated time evolution of the electric dipole expectation value (μZ) in the laboratory fixed frame, induced by the double-pulse excitation of the enantiomeric states φL and φR (blue and red colors) initially in the ground rotational state. The time delay between two pulses is set to 6 ps and the pulse mutual polarization angle is 45°.(Ref.93) [Citation93]
![Figure 8. Left: one-dimensional cut through the potential energy surface of HSOH calculated along the torsional coordinate rHSOH together with the definition of the molecule fixed axes system for two enantiomeric molecular configurations. right: calculated time evolution of the electric dipole expectation value (μZ) in the laboratory fixed frame, induced by the double-pulse excitation of the enantiomeric states φL and φR (blue and red colors) initially in the ground rotational state. The time delay between two pulses is set to 6 ps and the pulse mutual polarization angle is 45°.(Ref.93) [Citation93]](/cms/asset/46160caf-0c5d-4dc4-b99a-adbf83cf623d/tapx_a_1949390_f0008_oc.jpg)
The selectivity can also be accomplished by manipulating the ionization and dissociation processes using shaped-pulse laser fields. For example, the ionization processes of a series of similar methyl halide molecules have been studied using two-colored femtosecond laser fields with a controlled phase between them [Citation97]. A series of different polarized femtosecond laser pulses can be used to excite the left- and right-handed chiral molecules into different states, and further ionization or excitation can distinguish them, as proposed theoretically [Citation98,Citation99]. Under the irradiation of shaped femtosecond laser pulses, the control mechanisms of a series of molecules, with an acetone structure in which H atoms in one methyl radical are substituted by different atoms, are studied [Citation100]. By using shaped femtosecond laser pulses with binary phase, different mass spectrum fingerprints have been found for two xylene isomers, which will yield identical spectra under the irradiation of a transform-limited laser pulse [Citation101], as shown in . A dual-mass-spectrometer scheme has been proposed, combined with the closed-loop learning method, to identify isomers and control their reactions [Citation102]. A shaped excitation pulse in the deep ultraviolet has been used to control the ring-opening isomerization from 1,3-cyclohexadiene to 1,3,5-hexatriene [Citation103].
Figure 9. Coherent laser control mass spectra obtained for o- (open squares) and p-xylene (filled circles). The top panel shows results obtained with BP0 corresponding to TL laser pulses; the flat phase across the spectrum of the laser pulse is shown in the inset. The bottom panel shows data obtained with laser pulses with binary phase BP858, which is shown together with the spectrum of the pulse in the bottom inset. The mass spectra were normalized so that the molecular ion (M+) intensity equals unity. In the top and bottom panels, the region for m/z near 91, corresponding to the tropylium ion (T+), was amplified to highlight the observed differences. For the BP858 shaped pulses, the relative yield of T+ for p-xylene is 2.1 times greater than that observed for o-xylene.(Ref.101) [Citation101]
![Figure 9. Coherent laser control mass spectra obtained for o- (open squares) and p-xylene (filled circles). The top panel shows results obtained with BP0 corresponding to TL laser pulses; the flat phase across the spectrum of the laser pulse is shown in the inset. The bottom panel shows data obtained with laser pulses with binary phase BP858, which is shown together with the spectrum of the pulse in the bottom inset. The mass spectra were normalized so that the molecular ion (M+) intensity equals unity. In the top and bottom panels, the region for m/z near 91, corresponding to the tropylium ion (T+), was amplified to highlight the observed differences. For the BP858 shaped pulses, the relative yield of T+ for p-xylene is 2.1 times greater than that observed for o-xylene.(Ref.101) [Citation101]](/cms/asset/d7abc41c-d154-4ef8-b71f-2bb5182c6ba7/tapx_a_1949390_f0009_b.gif)
3.3. Manipulation
Molecules may have random orientations relative to the laser polarization when interacting with the laser, such as those in the gas phase molecule beam. The experimental results are averaged values without considering the spatial direction and spatial structure of molecules. However, spatial alignment and orientation of molecules have an essential effect on the preparation of the excited states and the following ionization and dissociation processes. Scientists want to study the alignment mechanisms to achieve the evolution, control, and application of the aligned molecule system [Citation104,Citation105].
The alignment degree that can be achieved with a transform-limited pulse is limited. Furthermore, one cannot use very high intensity since that will result in the ionization and dissociation of molecules. Theoretically, it has been proposed to use a shaped pulse train with two or more sub-pulses with fixed linear polarization to improve the alignment degree [Citation106–110]. The genetic algorithm has been used to search for the optimum pulse shape to achieve a high alignment degree [Citation111,Citation112]. Experimentally, double-pulse has been used to optimize molecule alignment [Citation109,Citation113,Citation114], periodic phase structures have been used to control the rotation dynamics and optimize the alignment of molecules [Citation110,Citation111], and the genetic algorithm has also been used to search for the optimum pulse [Citation112]. One axis of a three-dimensional polyatomic molecule can be aligned along the laser polarization direction using a linearly polarized laser. However, the alignments of the other two axes of the molecule are not affected. Experimentally, two pulses with different polarizations or pulse trains with rotating polarization have been used to control three-dimensional spatial alignment or movement of the molecule [Citation115–119]. For example, the directionality of molecular rotation is controlled by two experimental approaches (named as ‘double-kick’ and ‘chiral-pulse-train’ schemes). As shown in , using the technique of polarization-sensitive resonance-enhanced multiphoton ionization, the directionality control is achieved by changing the polarization and the time delay of shaped pulses [Citation119]. Complex polarization-shaped pulse trains are also proposed theoretically to control the spatial state of molecules [Citation120,Citation121].
Figure 10. (Color online) directionality of molecular rotation for (a), (c) J’’ = 3 and (b), (d) J’’ = 4 rotational states of 14N2. (a) and (b) show the double-kick excitation scheme, and (c) and (d) show the excitation with the chiral pulse train. note the differences in color scales: the maximum and minimum directionality achieved are ε = ±0.7 with the double-kick scheme and ε = ±0.4 with the chiral-train technique. (Ref.119) [Citation119]
![Figure 10. (Color online) directionality of molecular rotation for (a), (c) J’’ = 3 and (b), (d) J’’ = 4 rotational states of 14N2. (a) and (b) show the double-kick excitation scheme, and (c) and (d) show the excitation with the chiral pulse train. note the differences in color scales: the maximum and minimum directionality achieved are ε = ±0.7 with the double-kick scheme and ε = ±0.4 with the chiral-train technique. (Ref.119) [Citation119]](/cms/asset/25975451-0109-49e6-941d-838e13a2e7f7/tapx_a_1949390_f0010_oc.jpg)
The ionization and dissociation processes can be manipulated using shaped-pulse laser fields. The chemical bonds of molecules can be broken and rearranged irradiated by shaped laser fields, which enables us to further manipulate chemical reactions [Citation122,Citation123]. The ionization processes, such as above-threshold ionization [Citation124,Citation125], nonsequential double ionization [Citation126,Citation127], high-harmonic spectroscopy [Citation128], are also manipulated by variety of shaped-pulse laser fields. Because of the three-dimensional spatial distribution of molecule structure and quantum states, which also changes with time after excitation during ionization, dissociation, and isomerization, a light field with changing polarization can control the molecule dynamics more effectively. For example, when already aligned molecules are irradiated by subsequent different polarized laser pulses, the ionization efficiency will be different [Citation129,Citation130], or the molecule will be excited to different excited states, and different products will be generated [Citation131]. With polarization-shaped femtosecond laser pulses, research works have been done to optimize the ionization efficiency of molecules [Citation132,Citation133], the production ratio of oddly and evenly charged ions [Citation134], the transition between states [Citation135,Citation136], and the control of the photoelectron angular distributions [Citation137]. Depicted in , the Multiphoton ionization of potassium atoms can be manipulated by a sequence of two counter-rotating circularly polarized femtosecond laser pulses, which produces vortex-shaped photoelectron momentum distributions in the polarization plane [Citation138].Molecule rotation was controlled using a laser pulse with rotating polarization, and the rotation finally leads to its dissociation [Citation65]. Theoretically and experimentally, molecule torsional motion has been controlled [Citation139–142], and nuclear spin isomers can be selectively manipulated [Citation119,Citation141]. Vibrational states can be selectively excited, while shaped pulse trains with fixed polarization can not achieve such a high degree of selectivity [Citation143].
Figure 11. Sections through the reconstructed 3D electron distribution (ED) for (a) right-handed circularly polarized pulse, followed by the second right-handed circularly polarized pulse (RRCP), (b) right-handed circularly polarized pulse, followed by the second left-handed circularly polarized pulse (RLCP), and (c) left-handed circularly polarized pulse, followed by the second right-handed circularly polarized pulse (LRCP). Upper row: sections in the polarization plane at z = 0 compared to simulation results shown in the insets. Lower row: sections in the detector plane at x = 0. counter-rotating circularity pulse pairs generate vortex shaped EDs in contrast to RRCP pulse pairs, which create circularly symmetric EDs. (Ref.138) [Citation138]
![Figure 11. Sections through the reconstructed 3D electron distribution (ED) for (a) right-handed circularly polarized pulse, followed by the second right-handed circularly polarized pulse (RRCP), (b) right-handed circularly polarized pulse, followed by the second left-handed circularly polarized pulse (RLCP), and (c) left-handed circularly polarized pulse, followed by the second right-handed circularly polarized pulse (LRCP). Upper row: sections in the polarization plane at z = 0 compared to simulation results shown in the insets. Lower row: sections in the detector plane at x = 0. counter-rotating circularity pulse pairs generate vortex shaped EDs in contrast to RRCP pulse pairs, which create circularly symmetric EDs. (Ref.138) [Citation138]](/cms/asset/1c8c2b8f-499e-49a3-a119-b57f8654a929/tapx_a_1949390_f0011_oc.jpg)
4. Applications
4.1. Bioscience
Biological systems contain large molecules are complex. These biomolecules’ functions are the results of fundamental processes, such as energy and electron transfer, isomerization, proton transfer, which happens in femtosecond and picosecond time scales [Citation144]. It is hard to model such large systems theoretically. However, quantum effects can still be exploited. It has been demonstrated that coherence in single molecules can be controlled in disordered environments like encountered in biologically relevant systems [Citation145]. Fluorescence proteins are widely used in biological imaging. Chirped pulses were used to control the pump-dump dynamics of yellow fluorescence protein [Citation146]. It was demonstrated that quantum control techniques can be applied to large biomolecules in complicated environments [Citation33,Citation147,Citation148].
Raman spectroscopy has found applications in life sciences, including quantification of biomolecules and hyperspectral molecular imaging of cells and tissue [Citation149–151]. Raman microscopy has been used to detect DNA methylation status changes on a single cell level [Citation152]. Stimulated Raman Scattering (SRS) microscopy has been used to map nucleic acid distribution inside a cell, and cellular processes such as cell division can also be visualized [Citation153]. It has also been used to differentiate the tumor from the normal brain [Citation154]. Tumor margins can be clearly revealed, and hence safety and accuracy of surgeries are improved. An etalon filter generated asymmetric pulse was used as a pump pulse in SRS [Citation155]. It was found that the asymmetric pulse profile was responsible for doubled Raman signal intensity and reduced backgrounds. A sinusoidal spectral phase was applied to the broadband femtosecond pulse, and this single pulse was used to perform a coherent anti-Stokes Raman spectroscopy (CARS) measurement [Citation156]. The same pulse supplies the three photons required for the process. Moreover, because the shaped pulses’ peak intensity is weaker than the transform-limited pulse, the non-resonant Raman background is reduced. A FAST-CARS (Femtosecond Adaptive Spectroscopic Techniques applied to CARS) has been proposed to prepare the coherence between two vibrational states of the molecule and enhance the sensibility of Raman spectroscopy [Citation157]. Both preparation and reading pulses can be adaptively shaped to maximize the signal. It was also proposed that by maximizing differences of the spectral patterns, particular species can be identified. The spectra of the SRS excitation laser are tailored according to the Raman signatures of particular chemical species [Citation158]. Thus, the targeted species can be selectively excited, even in the presence of interfering species, which have overlapping Raman bands. The method has been successfully applied to the imaging of the distribution of protein, oleic acid and stearic acid, in Caenorhabditis elegans.
When imaging samples, shaped pulses can be used to improve the imaging capability. For example, under the irradiation of two phase-only shaped pulses, different chromophores in drosophila embryos labeled with enhanced green fluorescent protein can be selectively excited, enabling distinction of different parts in 2-Photon Excited Fluorescence (2PEF) images [Citation159], as illustrated in . However, current histopathology techniques require preprocessing of the samples, such as fixation, embedding, sectioning, and staining. These processes are time-consuming. They also increase patients’ costs. Most importantly, they have a destructive nature, which is attributed to the macroscopic force applied to the sample or the microscopic labeling molecules added. Multiphoton microscopy has been used for the molecular and cellular examination of unlabelled samples. For example, a supercontinuum laser was used in label-free multimodal multiphoton microscopy, including two-photon auto-fluorescence (2PAF), three-photon auto-fluorescence (3PAF), second-harmonic generation (SHG), third-harmonic generation (THG), and CARS or SRS [Citation160]. By modifying the laser spectrum using a pulse shaper, fast switching between different modalities is achieved while ensuring co-localized imaging. The pulses with different shapes now act as the marker molecules added to the sample. By manipulating light, maximal information can be obtained from a single sample, and the correlation between the markers can also be obtained. As the simplest form of shaped femtosecond pulses, pump-probe or pump-dump pulses can be used in microscopy imaging, especially for those molecules that do not fluoresce. These techniques are based on excited-state absorption, stimulated emission, ground-state depletion, and the photothermal effect, as reviewed in [Citation161]. Pump-probe imaging has been successfully applied to many systems, for example, the multi-color pump-probe imaging of heme proteins and red blood cells [Citation162].
Figure 12. 2PEF images of an eGFP (enhanced green fluorescence protein) labeled drosophila embryo. (a) blue-tuned excitation. (b)Red-tuned excitation. (c) Transform limited pulse. these three images are normalized to the fluorescence signal of the vitelline membrane. (d) Linear combination of A and B to isolate the eGFP fluorescence. (e) Linear combination of A and B to isolate the yolk fluorescence. (f) Composite image of C and D to illustrate the good separation between eGFP and yolk fluorescence. (Ref.159) [Citation159]
![Figure 12. 2PEF images of an eGFP (enhanced green fluorescence protein) labeled drosophila embryo. (a) blue-tuned excitation. (b)Red-tuned excitation. (c) Transform limited pulse. these three images are normalized to the fluorescence signal of the vitelline membrane. (d) Linear combination of A and B to isolate the eGFP fluorescence. (e) Linear combination of A and B to isolate the yolk fluorescence. (f) Composite image of C and D to illustrate the good separation between eGFP and yolk fluorescence. (Ref.159) [Citation159]](/cms/asset/ba434b4e-1ce6-4892-b1a8-737f30db5a07/tapx_a_1949390_f0012_oc.jpg)
It is common that biological systems have similar or identical absorption or emission spectrum. The Optimal Dynamic Discrimination (ODD) method was proposed to distinguish quantum systems, with minimal structural differences, through their dynamics [Citation163]. The ODD method was used to discriminate between two nearly identical biomolecules, riboflavin and flavin mononucleotide, which have almost identical absorption and fluorescence spectra [Citation164–166]. The fluorescence induced by a UV pulse can be depleted by the following IR pulse. The depletion signals are the same for the two molecules if the UV pulse and the IR pulse are both transform-limited. However, specially shaped UV pulses can be found to maximize or minimize the depletion ratios between the two molecules and can be used to identify them. The method was also used in discrimination between bio- and non-bio- aerosols with almost identical absorption/emission spectra [Citation167]. Pure NADH (reduced nicotinamide adenine dinucleotide) and enzyme-bound NADH have similar fluorescence spectrum shapes. By applying a π-shaped step phase across the spectrum, the fluorescence intensity as a function of step position can be measured. The curves’ shapes will be different for different enzyme concentrations and can be used to distinguish the unbound and bound molecules [Citation168]. The fluorescence depletion of tryptophan (Trp) and tyrosine (Tyr) molecules is controlled [Citation169]. When irradiated with the found optimum pulse shape, a signal change of 35% is obtained on Trp selectively, while Tyr depletion remains unchanged.
Pulse shapers can be used not only to generated shaped pulses but also to obtain transform-limited pulses. This is important, especially for bioscience applications of short pulses. MIIPS method can measure and compensate the dispersion of the output pulses from a fiber laser oscillator at the microscope objective’s focus. By ensuring that the short pulses are transform-limited, it was found that signal intensity of Third Harmonic Generation (THG) was ten times larger when 30 fs pulses were used compared to 100 fs pulse [Citation170]. The sub-40 fs, 1060 nm pulses from a fiber laser can provide ~80% increase of penetration depth in human skin samples [Citation171]. Using the compensated sub 10 fs pulses, greater signal, increased depth, and less photobleaching were obtained in two-photon microscopy with samples like HeLa cells and mouse kidney tissue [Citation172,Citation173]. The broad spectrum of this short laser pulse also enables selective excitation of subcellular components. The effect of different pulse shapes on photobleaching was also studied [Citation174]. The fundamental laser spectrum’s shape does not change by applying the spectral phase to the laser pulse. However, because of multiphoton Intrapulse interference [Citation175], the multiphoton spectra may change. Acidic and basic regions of the sample have been selectively excited with the shaped pulse, and suppression of multiphoton induced damage was achieved [Citation176].
In Bioscience applications, the laser may need to pass through a turbid scattering medium, and its coherence may be lost. However, it was demonstrated that the phase information used to excite the samples selectively could survive after passing through tissue, and decoherence and optical resolution were not affected by pulse shaping [Citation177]. Shaped laser with a π-phase step is transmitted through optically dense Indocyanine Green sample, and an enhancement of stimulated emission up to 14 times, compared to that from the transform-limited pulse, was observed [Citation178]. Potential applications were proposed, like the transmission of signals through absorbing media or controlling photodynamic therapy (PDT).
The closed-loop learning method has been successfully used in controlling single molecule reactions. It has been demonstrated that the method is also capable of searching for the optimal solution in complex biological systems. CLL method has been used to improve the fluorescence intensities per unit pump intensity from complex bio-related molecules, such as GFP or BFP (Blue Fluorescence Protein), and decrease photobleaching simultaneously [Citation179–182], as well as selective excitation and discrimination of different dyes [Citation183]. It has also been used to find pulse shapes to control the branching ratio between two energy flow pathways of a bioinspired dyad molecule [Citation184]. PDT uses light to excite photosensitizer molecules. The molecules then produce singlet oxygen which is toxic to cells. PDT is used in the treatment of cancer and other diseases. Closed-loop and open-loop methods were used to control the ratio between singlet and triplet states of zinc phthalocyanine, a model photosensitizer [Citation185]. Using the shaped broadband pump and probe pulses in CARS, it was demonstrated that the evolutionary algorithm could find optimal pulse shapes, which will enhance the desired molecule’s response and suppress the contribution from the other four molecules with overlapping resonance [Citation186]. The method can be used in chemically-selective microscopy.
Matrix-Assisted Laser Desorption Ionization Time-Of-Flight Mass Spectrometry (MALDI TOF MS) is an important tool for DNA sequencing [Citation187,Citation188]. It has also been used to identify different respiratory viruses by proteome profiling of virus-infected and uninfected cell cultures [Citation189]. Using femtosecond Laser Ablation Time-Of-Flight Mass Spectroscopy (fsLA-TOF-MS), by comparing spectra of different isotope-labeled RNAs, the number of nucleotide contents was reproduced [Citation190]. Shaped femtosecond laser pulses might be used as the desorption laser in MALDI TOF MS to control the shape of mass spectra and increase the sensitivity. Identification of different biomolecules is possible without the need to map the complete structure.
4.2. Laser machining
Laser material interaction is a complex phenomenon involving several phases and multiple lengths and time scales [Citation191]. To improve the quality, resolution, and efficiency of micromachining, temporal, spatial, and spatiotemporal laser pulse shaping techniques have been developed [Citation192]. The sub-pulses can fit the many stages in the ablation process.
Double-pulse has been widely used in the study of laser-material interaction. The ablation rate can be calculated by measuring the ions generated during the ablation or the crater morphology after ablation. Compared to a single pulse with the same total fluence, using a double pulse, it was found that the ablation rate may be higher for some materials (usually semiconductors) [Citation193–195], while lower for some other materials (usually dielectrics) [Citation196,Citation197]. This general rule may not always be valid because the ablation dynamics will differ depending on laser fluence, pulse width, and many other experimental parameters, and the ablation rate will change (increase and decrease in different double-pulse delay regions). Some works studied the damage threshold or breakdown threshold change under the irradiation of double-pulse [Citation198,Citation199]. It was found that under some conditions, with each sub-pulse below the damage threshold of material, the double-pulse can still initiate the ablation. The plume generated by the first pulse may shield the second pulse partially or completely from reaching the sample surface, and it might be reheated by the second pulse [Citation200–202]. The laser-induced breakdown spectral emission can be enhanced with double-pulse irradiation compared to a single pulse, which can be explained by the plasma reheating effect or by better coupling of the second pulse to the liquid phase generated by the first one [Citation88,Citation203]. Some works have studied the crater’s morphology under the irradiation of double-pulse and the influence of double-pulse on the nanostructure on the surface [Citation204,Citation205]. It was also reported that the particle size in the ejected material from the ablation of copper and gold changes with delay, which was explained as the atomization of the nanoparticles in the plume by the second pulse [Citation206,Citation207]. The same effect was also observed in the hole drilling study of metal films [Citation208].
Works have been done to compare the crater morphology after multiple-pulse (with pulse number greater than two) irradiation and single pulse irradiation [Citation209–211]. On dielectrics, cleaner and smoother craters were obtained with multiple-pulse, while cracks were seen using single-pulse. Using the genetic algorithm, pulse shapes have been found to enhance the silicon ion emission from the ablation [Citation194,Citation212] and control the generated nanoparticle size [Citation213]. The coupling of laser energy with the material has been studied under the irradiation of shaped pulse and single pulse [Citation214]. The effect of laser pulse bandwidth and phase on the plasma optical emission intensity has been studied [Citation215]. Significant photoluminescence intensity enhancement has been observed in the shaped pulse ablation of GaAs [Citation216,Citation217]. It was proposed that the control was achieved by coherent manipulation of plasma electrons, which in turn excite lattice phonons. The effect of temporal pulse shaping on the plasma composition and kinetics from aluminum and carbon ablation was studied [Citation218]. Theoretically, many research works also analyzed and simulated the ablation processes under the irradiation of femtosecond laser pulse train [Citation211,Citation219–221]. Femtosecond laser structuring can have very high precision. Using asymmetric pulse train, crater sizes much smaller than the diffraction limit have been obtained [Citation222,Citation223]. Short 12 fs pulses, with picojoule pulse energy and sub-milliwatt mean powers, are used to cut lines in cell nuclei and drill holes in chromosomes [Citation224].
Methods have been developed to control laser-matter interactions to produce particular nano-/micro- structures on the surface, which shows novel properties and potential applications [Citation225]. Various laser surface structures can be formed, including periodic structures, nanoholes, controlled irregular nanostructures, and nanostructure-textured microstructures. With these structures, materials can show different colors or be completely black; the surface’s wettability can be controlled; the biomedical properties like cell adhesion and growth can be modified. We can expect more inspiring applications for matter with particular surface. For example, the black metal has high absorptivity, making it an excellent candidate for solar energy collectors or advanced stealth technology. Sharp conical spikes have been produced on the silicon surface irradiated by femtosecond laser pulses [Citation226]. The spikes only formed in SF6 or Cl2 environment, not in the vacuum, N2, or He, suggesting that chemical reaction was involved during the laser ablation process.
4.3. Novel material fabrication
Clusters (from the point of view of atoms and molecules), or nanoparticle (from the point of view of macroscopic materials), is a special kind of matter. Because of the large surface area to size ratio, the size and structure have important influences on the physical and chemical properties of the cluster itself and the macroscopic material formed by it [Citation227]. Various methods have been developed to control the clusters’ size distribution using lasers [Citation228–233].
Ionization processes of C60 have been studied by using lasers with different wavelengths. The cluster tends to dissociate under resonance conditions, while it tends to form larger clusters under non-resonance conditions [Citation234]. Double-pulse has been used to control the Coulomb explosion of Ag clusters [Citation235]. Double-pulse and triple-pulse have been used to control the ionization processes of Xe clusters [Citation236]. The electrons can be ionized from the atom but still confined in the cluster, or they can be removed from the cluster permanently. The two ionization mechanisms can be selected by varying the double-pulse delay. The nanoparticle’s shape can also be changed by the irradiation of the laser, from nanorod to nanodot, and femtosecond laser was shown to be more effective [Citation237]. The genetic algorithm has been used to control the generated cluster size during laser ablation of semiconductors [Citation213]. Optimal pulse shape can also be found to enhance the ionic emission selectively with respect to the neutral emission lines during the laser ablation of aluminum [Citation238].
Silicon clusters have been generated by the femtosecond laser ablation method [Citation239], and the influence of non-thermal melting has been studied. Under the normal melting mechanism, electrons absorb energy from laser photons and reach a high temperature. Then electrons collide with phonons and heat the lattice to induce melting. Under the irradiation of ultrashort laser pulses, non-thermal melting can happen [Citation240–243]. The electrons will then be excited to antibonding orbital, and the bond between atoms will break. This kind of melting is non-thermal and can happen very fast, even within 1 ps. The system is in a low lattice temperature metastable state. Next, phase explosion will happen, and more clusters will be generated instead of single atoms. During this non-thermal melting stage, femtosecond laser control can play a significant role.
Laser ablating bulk materials into clusters is an important cluster generation method. With shaped femtosecond laser pulses, we can control different stages of the whole experiment. Firstly, we can use shaped pulses to control and optimize the ablation process. During the transportation period, for example, in the gas flow from the laser-target interaction region to the ionization region in a mass spectrometer, another shaped femtosecond laser can be used to change the size distribution and control the isomerization from one structure to another. A third shaped laser can be used in the detection stage to identify clusters with similar structures, which will show identical spectrum under the irradiation of the transform-limited pulse. This laser can also be used in the collection stage if our goal is to produce clusters with specific structures. For example, it may be used to dissociate clusters with other structures and leave the one we want.
4.4. Trace material detection
In various fields, there are needs to detect trace amounts of materials. High sensitivity and selectivity are the two fundamental requirements because of the weak and usually overlapped signals from conventional methods. For some applications, for example, explosive detection in a large public area, non-destructive, eye- and skin-safe are required. The shaped-pulse light field may find its applications in this area.
The CARS method with a single phase-shaped femtosecond broadband pulse as the pump, stokes, and probe beams, was used to detect and identify various materials, including trace amount of explosives, at a distance [Citation244]. Using a broadband laser as the pump and stokes beam, the CARS method was used to detect trace quantities of explosives [Citation245]. A fiber laser operating in the 1550 nm-1800 nm eye-safe wavelength range was used, making the system a promising tool for future implementation in public places. Single Beam SRS (SB-SRS), or Single Ultrafast Pulse Excited Remote SRS (SUPER-SRS), was used in standoff detection of trace amounts of explosives [Citation246,Citation247]. The phase was applied to the laser spectrum, enabling selective excitation of a particular vibrational mode (of a particular substance). A complete spectrum can be obtained by scanning the phase. Simultaneous detection of multiple explosives can also be realized by applying a more complex spectral phase that will selectively excite two or more Raman modes or by adding time-delayed shaped pulses.
Due to the balanced effect of self-focusing and diffraction at the focus, an intense femtosecond laser will form filaments when propagating in the air [Citation248]. The light detection and ranging (LIDAR) method can detect trace material in the atmosphere remotely. The backscatter signals from gas molecules and aerosols are collected and analyzed to get the composition, abundance, distance, size, and phase information. The mobile femtosecond-terawatt laser system, has been constructed to study these applications, and pulse shaping was expected to benefit in terms of phase correction of the laser pulse after long-range (up to a few tens of km) propagations in air, as well as identifying bioagents that have identical linear fluorescence spectrum [Citation249–251]. Along the filament path, the molecules will be aligned. The existence of the aligned molecules and the recurrence of the alignment states will affect the propagation of subsequent laser pulses. For example, when propagating at different delays relative to the leading pulse, the subsequent pulse will experience focusing, diverging or spectral broadening [Citation252]. The white light generation can be enhanced [Citation253]. The filament length can be elongated [Citation254,Citation255]. The repulsion and attraction of adjacent filament can be controlled [Citation256,Citation257]. Biological warfare agents, like bacteria, viruses, in airborne aerosol form, have been detected using ultraviolet laser-induced fluorescence LIDAR, with the laser pulse energy to be 100 mJ or more [Citation258].
4.5. Military and defense
Lasers have potential military applications, such as range-finders, target designation, data relay devices, directed energy weapons, weather modifiers, and more [Citation259]. Lasers are more favorite directed-energy weapons because they can deliver energy at the speed of light, and they have low shot-to-shot operational costs [Citation260]. Conventional High-Energy Laser (HEL) weapons use lasers with the power of 100 kW or more, though some 10 kW, 50 kW models are also being developed [Citation260]. Higher power means weight increase. Efforts are being made to develop a lighter system, for example, less than five kg/kW, for tactical aircraft integration [Citation260]. Powerful femtosecond lasers are also being built. For example, the High Repetition Rate Advanced Petawatt Laser System (HAPLS) from Lawrence Livermore National Laboratories (LLNL; Livermore, California, USA) will deliver 30 fs pulses with more than 30 J per pulse [Citation260].
Shaped ultrafast laser pulses can be used to increase the sensitivity of laser trace material detection, to detect disguised vehicles and weapons, warfare agents. Shaped pulse material ablation and micromachining can be used to treat the surfaces of warships and planes, to achieve corrosion protection, or strong radar wave absorption. Nanometer and micrometer sized structures on the surface can be tuned to increase the absorption of light and radar wave [Citation225,Citation261,Citation262]. Changing the shape of the laser field has a big influence on the morphology of the irradiated area [Citation208,Citation263–266]. Charge Coupled Devices (CCDs) and other sensitive electronics can be destroyed by intense light. They need to be protected by window that has a high optical density at the laser wavelength or optical limiting materials that turns opaque at high intensity [Citation267]. Such laser protection shield can be penetrated with shaped pulses by manipulating the wave packet on the excited states such that the following laser photon does not have enough energy to reach higher state, and hence the induced transparency is achieved [Citation90,Citation91]. High intensity laser weapons will benefit from using a shaped laser pulse in two ways. The first is that aligned atmosphere molecules by a pre- pulse-train will increase the effective distance of the main laser [Citation255]. The second is that shaped pulse will increase the ablation efficiency [Citation89,Citation217,Citation268]. Hence, lasers with the same intensity will have larger destroying ability. Or, lower intensity lasers will be needed to achieve the same destroying ability, which means less weight and size.
5. Conclusions
A femtosecond laser is a common tool in scientific research laboratories. However, it is rarely used in other applied fields, except in some eye surgeries [Citation269,Citation270]. The situation results from the lack of understanding or control of the microscopic dynamics and the lack of compatible laser systems. For example, some applications, such as laser weapons, mass production, require lasers to have very high pulse energy, peak power, or average power. Such requirements cannot be met by current laboratory lasers, but these lasers can be built if applications need them. Projects like International Coherent Amplification Network (ICAN) have led to powerful femtosecond laser systems with pulse energies above tens of joules, repetition rates above tens of kilohertz, and peak powers above hundreds of terawatts [Citation271,Citation272]. Depending on the application, the femtosecond laser system’s size, weight, and pulse characteristics will need to be considered. Smaller mobile systems can be fitted to a truck or even possible to an earth-orbiting satellite [Citation273,Citation274].
After enough research and development, a wide range of products utilizing the science and technology of shaped-pulse light fields may become realities. For example, biochemical direct reading equipment can replace biochemical reagents, detect hazardous elements in food or the environment, and quickly detect viruses. Medical equipment can image tissue and cellular samples without labeling, killing tumor cells without affecting healthy cells. Non-lethal anti-terrorism equipment can detect dangerous substances remotely and incapacitate terrorists temporarily. Military equipment can detect stealth planes, vehicles and mines, disable missile seekers or radars, and detect warfare agents on the battlefield. Material manufacturing equipment can make materials with novel clusters as constructing elements, and make materials that have nanostructures. Pharmaceutical manufacturing equipment can make medicines with particular molecular structures, for example, selective production of one of the enantiomers. Results in the field of coherent control using shaped femtosecond laser pulses are thriving in the past years. Now it has come to the time that practical applications are feasible. With dedicated efforts to industrialize these sciences and technologies, a new era will soon come, and our life has changed at the atomic and molecular level.
Disclosure statement
No potential conflict of interest was reported by the author(s).
Additional information
Funding
References
- Sinha A, Hsiao MC, Crim FF. Bond-selected bimolecular chemistry: h+HOD(4νOH)→OD+H2. J Chem Phys. 1990;92(10):6333.
- Shapiro M, Brumer P. Laser control of product quantum state populations in unimolecular reactions. J Chem Phys. 1986;84(7):4103.
- Park SM, Lu S-P, Robert JG. Coherent laser control of the resonance-enhanced multiphoton ionization of HCl. J Chem Phys. 1991;94(12):8622.
- Zhu L, Kleiman V, Li X, et al. Coherent Laser Control of the Product Distribution Obtained in the Photoexcitation of HI. Science. 1995;270(5233):77.
- Nagai H, Ohmura H, Ito F, et al. Coherent phase control of the product branching ratio in the photodissociation of dimethylsulfide. J Chem Phys. 2006;124(3):034304.
- Potter ED, Herek JL, Pedersen S, et al. Femtosecond laser control of a chemical reaction. Nature. 1992;355(6355):66.
- Herek JL, Materny A, Zewail AH. Femtosecond control of an elementary unimolecular reaction from the transition-state region. Chem Phys Lett. 1994;228:15.
- Scherer NF, Ruggiero AJ, Du M, et al. Time resolved dynamics of isolated molecular systems studied with phase‐locked femtosecond pulse pairs. J Chem Phys. 1990;93:856.
- Scherer NF, Carlson RJ, Matro A, et al. Fluorescence-detected wave packet interferometry: time resolved molecular spectroscopy with sequences of femtosecond phase-locked pulses. J Chem Phys. 1991;95(3):1487.
- Brixner T, Gerber G. Quantum Control of Gas-Phase and Liquid-Phase Femtochemistry. Chemphyschem. 2003;4(5):418.
- Tannor DJ, Rice SA. Control of selectivity of chemical reaction via control of wave packet evolution. J Chem Phys. 1985;83(10):5013.
- David RKSAR, Kosloff R, Rice SA. J. Tannor. Coherent pulse sequence induced control of selectivity of reactions: Exact quantum mechanical calculations. J. Chem. Phys. 1986;85:5805.
- Judson RS, Rabitz H. Teaching lasers to control molecules. Phys Rev Lett. 1992;68(10):1500.
- Assion A, Baumert T, Bergt M, et al. Control of Chemical Reactions by Feedback-Optimized Phase-Shaped Femtosecond Laser Pulses. Science. 1998;282:919.
- Levis RJ, Menkir GM, Rabitz H. Selective Bond Dissociation and Rearrangement with Optimally Tailored, Strong-Field Laser Pulses. Science. 2001;292(5517):709.
- Vajdaa S, Rosendo-Francisco P, Kaposta C, et al. Analysis and control of ultrafast photodissociation processes in organometallic molecules. Eur.Phys. J. D. 2001;16(1):161.
- Graham P, Menkir G, Levis RJ. An investigation of the effects of experimental parameters on the closed-loop control of photoionization/dissociation processes in acetophenone. Spectrochimica Acta Part B. 2003;58(6):1097.
- Baumert T, Brixner T, Seyfried V, et al. Femtosecond pulse shaping by an evolutionary algorithm with feedback. Appl. Phys. B-Lasers O. 1997;65:779.
- Bardeen CJ, Yakovlev VV, Wilson KR, et al. Feedback quantum control of molecular electronic population transfer. Chem Phys Lett. 1997;280(1–2):151.
- Bartels R, Backus S, Zeek E, et al. Shaped-pulse optimization of coherent emission of high-harmonic soft X-rays. Nature. 2000;406(6792):164.
- Papastathopoulos E, Strehle M, Gerber G. Optimal control of femtosecond multiphoton double ionization of atomic calcium. Chem Phys Lett. 2005;408(1–3):65.
- Bartelt A, Lindinger A, Lupulescu C, et al. Optimal control of multi-photon dissociation and ionization processes in small NamKnclusters. PhysChem Chem Phys. 2004;6(8):1679.
- Lindinger A, Lupulescu C, Bartelt A, et al. Coherent control of alkali cluster fragmentation dynamics. Spectrochimica Acta Part B. 2003;58(6):1109.
- Yokoyama K, Teranishi Y, Toya Y, et al. Optimal control of ultrafast selection. J Chem Phys. 2004;120(20):9446.
- Rabitz H. The role of theory in the laboratory control of quantum dynamics phenomena. Theory Chem Acc. 2003;109(2):64.
- Ho T-S, Rabitz H. Why do effective quantum controls appear easy to find? J. Photoch. Photobio. A. 2006;180(3):226.
- Geremia JM, Zhu WS, Rabitz H. Incorporating physical implementation concerns into closed loop quantum control experiments. J Chem Phys. 2000;113(24):10841.
- Mitraa A, Rabitz H. Quantum control mechanism analysis through field based Hamiltonian encoding. J Chem Phys. 125(19):194107.
- Vogt G, Nuernberger P, Selle R, et al. Analysis of femtosecond quantum control mechanisms with colored double pulses. Phys Rev A. 2006;74(3):033413.
- Nuernberger P, Vogt G, Brixner T, et al. Femtosecond quantum control of molecular dynamics in the condensed phase. Phys Chem Chem Phys. 2007;9:2470.
- Engel GS, Calhoun TR, Read EL, et al. Evidence for wavelike energy transfer through quantum coherence in photosynthetic systems. Nature. 2007;446(7137):782.
- Herek JL, Wohlleben W, Cogdell RJ, et al. Quantum control of energy flow in light harvesting. Nature. 2002;417(6888):533.
- Prokhorenko VI, Nagy AM, Waschuk SA, et al. Coherent Control of Retinal Isomerization in Bacteriorhodopsin. Science. 2006;313(5791):1257.
- Kuroda DG, Singh CP, Peng ZH, et al. Mapping Excited-State Dynamics by Coherent Control of a Dendrimer’s Photoemission Efficiency. Science. 2009;326:263.
- Wattellier B, Sauteret C, Chanteloup JC, et al. Beam-focus shaping by use of programmable phase-only filters: application to an ultralong focal line. Opt Lett. 27:213.
- Mosk AP, Lagendijk A, Lerosey G, et al. Controlling waves in space and time for imaging and focusing in complex media. Nat Photonics. 2012;6:283.
- Haner M, Warren WS. Synthesis of crafted optical pulses by time domain modulation in a fiber-grating compressor. Appl Phys Lett. 1988;52(18):1458.
- Weiner AM. Femtosecond pulse shaping using spatial light modulators. Rev Sci Instrum. 2000;71(5):1929.
- Weiner AM, Heritage JP, Kirschner EM. High-resolution femtosecond pulse shaping. J Opt Soc Am B. 1988;5:1563.
- Weiner AM, Leaird DE, Patel JS, et al. Programmable femtosecond pulse shaping by use of a multielement liquid-crystal phase modulator. Opt Lett. 1990;15(6):326.
- Weiner AM, Leaird DE, Patel JS, et al. Programmable shaping of femtosecond optical pulses by use of 128-element liquid crystal phase modulator. IEEE J. Quantum Elect. 1992;28(4):908.
- Wefers MM, Nelson KA. Ultrafast Optical Waveforms. Science. 1993;262(5138):1381.
- Marc MW, Keith AN. Programmable phase and amplitude femtosecond pulse shaping. Opt Lett. 1993;18(23):2032.
- Marc MW, Keith AN. Generation of high-fidelity programmable ultrafast optical waveforms. Opt Lett. 1995;20(9):1047.
- Hillegas CW, Tull JX, Goswami D, et al. Femtosecond laser pulse shaping by use of microsecond radio-frequency pulses. Opt Lett. 1994;19(10):737.
- Ren Y-X, Lu R-D, Gong L. Tailoring light with a digital micromirror device. Ann. Phys.-Berlin. 2015;527(7–8):447.
- Daniel JK, Rick T. Characterization of arbitrary femtosecond pulses using frequency-resolved optical gating. IEEE J. Quantum Elect. 1993;29(2):571.
- Iaconis C, Walmsley IA. Spectral phase interferometry for direct electric-field reconstruction of ultrashort optical pulses. Opt Lett. 1998;23(10):792.
- Lozovoy VV, Pastirk I, Dantus M. Multiphoton intrapulse interference IV Ultrashort laser pulse spectral phase characterization and compensation. Opt Lett. 2004;29:775.
- Pastirk I, Resan B, Fry A, et al. No loss spectral phase correction and arbitrary phase shaping of regeneratively amplified femtosecond pulses using MIIPS. Opt Express. 2006;14(20):9537.
- Fetterman MR, Goswami D, Keusters D, et al. Ultrafast pulse shaping: amplification and characterization. Opt Express. 1998;3(10):366.
- Roth M, Mehendale M, Bartelt A, et al. Acousto-optical shaping of ultraviolet femtosecond pulses. Appl. Phys. B-Lasers O. 2005;80(4–5):441.
- Parker DSN, Nunn ADG, Minns RS, et al. Frequency doubling and Fourier domain shaping the output of a femtosecond optical parametric amplifier: easy access to tuneable femtosecond pulse shapes in the deep ultraviolet. Appl. Phys. B-Lasers O. 2009;94(2):181.
- Nuernberger P, Selle R, Langhojer F, et al. Polarization-shaped femtosecond laser pulses in the ultraviolet. Journal of Optics a-Pure and Applied Optics. 2009;11(8):085202.
- Hertz E, Billard F, Karras G, et al. Shaping of ultraviolet femtosecond laser pulses by Fourier domain harmonic generation. Opt Express. 24(24):27702.
- Shimizu S, Nabekawa Y, Obara M, et al. Spectral phase transfer for indirect phase control of sub-20-fs deep UV pulses. Opt Express. 2005;13(17):6345.
- Lazzarino LL, Kazemi MM, Haunhorst C, et al. Shaping femtosecond laser pulses at short wavelength with grazing-incidence optics. Opt Express. 2019;27:13479.
- Krogen P, Suchowski H, Liang HK, et al. Generation and multi-octave shaping of mid-infrared intense single-cycle pulses. Nat Photonics. 2017;11(4):222.
- Maksimenka R, Nuernberger P, Lee KF, et al. Direct mid-infrared femtosecond pulse shaping with a calomel acousto-optic programmable dispersive filter. Opt Lett. 2010;35(21):3565.
- Shim SH, Strasfeld DB, Fulmer EC, et al. Femtosecond pulse shaping directly in the mid-IR using acousto-optic modulation. Opt Lett. 2006;31(6):838.
- Ma J, Li Q, Zhou W, et al. Progress of Terahertz Pulse Shaping Techniques. Laser Optoelectron Prog. 2012;49:090004.
- Hoffmann MC, Grguras I, Behrens C, et al. Femtosecond profiling of shaped x-ray pulses. New J Phys. 2018;20(3):033008.
- Misawa K. Applications of polarization-shaped femtosecond laser pulses. Adv. Phys.-X. 2016;1:544.
- Kakehata M, Ueda R, Takada H, et al. Combination of high-intensity femtosecond laser pulses for generation of time-dependent polarization pulses and ionization of atomic gas. Appl. Phys. B-Lasers O. 2000;70:s207.
- Villeneuve DM, Aseyev SA, Dietrich P, et al. Forced Molecular Rotation in an Optical Centrifuge. Phys Rev Lett. 2000;85:542.
- Kitzler M, Lezius M. Spatial Control of Recollision Wave Packets with Attosecond Precision. Phys Rev Lett. 2005;95(25):253001.
- Shafir D, Mairesse Y, Villeneuve DM, et al. Atomic wavefunctions probed through strong-field light–matter interaction. Nat Phys. 2009;5:412.
- Brixner T, Gerber G. Femtosecond polarization pulse shaping. Opt Lett. 2001;26(8):557.
- Brixner T, Krampert G, Niklaus P, et al. Generation and characterization of polarization-shaped femtosecond laser pulses. Appl. Phys. B-Lasers O. 2002;74:s133.
- Plewicki M, Weise F, Weber SM, et al. Phase, amplitude, and polarization shaping with a pulse shaper in a Mach-Zehnder interferometer. Appl. Optics. 2006;45(32):8354.
- Plewicki M, Weber SM, Weise F, et al. Independent control over the amplitude, phase, and polarization of femtosecond pulses. Appl. Phys. B-Lasers O. 2007;86:259.
- Weise F, Lindinger A. Full parametric pulse shaping in phase, amplitude, and polarization using an effective four-array modulator. Appl. Phys. B-Lasers O. 2010;101(1–2):79.
- Esumi Y, Kabir MD, Kannari F. Spatiotemporal vector pulse shaping of femtosecond laser pulses with a multi-pass two-dimensional spatial light modulator. Opt Express. 2009;17:19153.
- Zhao Z, Wang J, Li S, et al. Metamaterials-based broadband generation of orbital angular momentum carrying vector beams. Opt Lett. 2013;38(6):932.
- Harris H, Hill CA, Tapster PR. Tapster, and Vaughan, Physical review. A, Atomic, molecular, and optical physics. 1994;49(4):3119.
- Strain MJ, Cai X, Wang J, et al. Fast electrical switching of orbital angular momentum modes using ultra-compact integrated vortex emitters. Nat Commun. 2014;5:4856.
- Cai X, Wang J, Strain MJ, et al. Integrated Compact Optical Vortex Beam Emitters. Science. 2012;338(6105):363.
- Du LP, Man ZS, Zhang YQ, et al. Manipulating orbital angular momentum of light with tailored in-plane polarization states. Sci Rep. 2017;7:41001.
- Chen SQ, Liu WW, Li ZC, et al. Metasurface-Empowered Optical Multiplexing and Multifunction. Adv Mater. 2020;32(3):1805912.
- Li ZC, Liu WW, Cheng H, et al. Spin-Selective Full-Dimensional Manipulation of Optical Waves with Chiral Mirror. Adv Mater. 2020;32(26):1907983.
- Liu WW, Ma DN, Li ZC, et al. Aberration-corrected three-dimensional positioning with a single-shot metalens array. Optica. 2020;7(12):1706.
- Bruhl E, Buckup T, Motzkus M. Minimization of 1/f^n phase noise in liquid crystal masks for reliable femtosecond pulse shaping. Opt Express. 2017;25(19):23376.
- Dinda S, Bandyopadhyay SN, Goswami D. Rapid programmable pulse shaping of femtosecond pulses at the MHz repetition rate. OSA Contin. 2019;2(4):1386.
- Yushkov KB, Molchanov VY. Randomly Spaced Phase-Only Transmission Combs for Femtosecond Pulse Shaping. IEEE J. Sel. Top. Quant. 2020;26(5):8700108.
- Kohler J, Wollenhaupt M, Bayer T, et al. Zeptosecond precision pulse shaping. Opt Express. 2011;19(12):11638.
- Yakovlev VV, Bardeen CJ, Che J, et al. Chirped pulse enhancement of multiphoton absorption in molecular iodine. J Chem Phys. 1998;108(6):2309.
- Song Y-D, Chen Z, Yang X, et al. Control of the photoionization/photodissociation processes of cyclopentanone with trains of femtosecond laser pulses. Chinese Phys B. 2013;22:103301.
- Mildner J, Sarpe C, Gotte N, et al. Emission signal enhancement of laser ablation of metals (aluminum and titanium) by time delayed femtosecond double pulses from femtoseconds to nanoseconds. Appl Surf Sci. 2014;302:291.
- Hu Z, Singha S, Liu Y, et al. Mechanism for the ablation of Si⟨111⟩ with pairs of ultrashort laser pulses. Appl Phys Lett. 2007;90(13):131910.
- Hu Z, Singha S, Zhao YB, et al. Coherent Control of the Photoionization of Pyrazine. J Phys Chem Lett. 2012;3:2744.
- Gordon RJ, Hu Z, Seideman T, et al. Coherent phase control of internal conversion in pyrazine. J Chem Phys. 2015;142(14):144311.
- Avalos MN, Babiano R, Cintas P, et al. Absolute Asymmetric Synthesis under Physical Fields: facts and Fictions. Chem Rev. 1998;98:2391.
- Yachmenev A, Yurchenko SN. Detecting Chirality in Molecules by Linearly Polarized Laser Fields. Phys Rev Lett. 2016;117(3):033001.
- Gershnabel E, Averbukh IS. Orienting Asymmetric Molecules by Laser Fields with Twisted Polarization. Phys Rev Lett. 2018;120(8):083204.
- Tutunnikov I, Gershnabel E, Gold S, et al. Selective Orientation of Chiral Molecules by Laser Fields with Twisted Polarization. J Phys Chem Lett. 2018;9(5):1105.
- Milner AA, Fordyce JAM, MacPhail-Bartley I, et al. Controlled Enantioselective Orientation of Chiral Molecules with an Optical Centrifuge. Phys Rev Lett. 2019;122(22):223201.
- Ohmura H, Ito F, Tachiya M. Phase-sensitive molecular ionization induced by a phase-controlled two-color laser field in methyl halides. Phys Rev A. 2006;74(4):043410.
- Hoki K, Kröner D, Manz J. Selective preparation of enantiomers from a racemate by laser pulses: model simulation for oriented atropisomers with coupled rotations and torsions. Chem Phys. 2001;267(1–3):59.
- Li Y, Bruder C. Dynamic method to distinguish between left- and right-handed chiral molecules. Phys Rev A. 2008;77(1):015403.
- Cardoza D, Baertschy M, Weinacht T. Understanding learning control of molecular fragmentation. Chem Phys Lett. 2005;411(4–6):311.
- Cruz JMD, Lozovoy VV, Dantus M. Quantitative Mass Spectrometric Identification of Isomers Applying Coherent Laser Control. J Phys Chem A. 2005;109(38):8447.
- Chen Z, Tong Q-N, Zhang -C-C, et al. Identification of isomers and control of ionization and dissociation processes using dual-mass-spectrometer scheme and genetic algorithm optimization. Chinese Phys B. 2015;24(4):043303.
- Kotur M, Weinacht T, Pearson BJ, et al. Closed-loop learning control of isomerization using shaped ultrafast laser pulses in the deep ultraviolet. J Chem Phys. 2009;130:134311.
- Stapelfeldt H, Seideman T. Colloquium: aligning molecules with strong laser pulse. Rev Mod Phys. 2003;75(2):543.
- Seideman T, Hamilton E. Advances in Atomic. Molecular and Optical Physics. 2006;52:289.
- Leibscher M, Averbukh IS, Rabitz H. Molecular Alignment by Trains of Short Laser Pulses. Phys Rev Lett. 2003;90(21):213001.
- Ortigoso J. Conservation of Molecular Alignment for Cyclic Rotational Wave Packets in Periodic Pulse Trains. Phys Rev Lett. 2004;93(7):073001.
- Leibscher M, Averbukh IS, Rabitz H. Enhanced molecular alignment by short laser pulses. Phys Rev A. 2004;69(1):013402.
- Zeng GP, Zhong FJ, Wu CY, et al. Field-free molecular alignment and its application. Laser Phys. 2009;19(8):1691.
- Zhang S, Lu C, Jia T, et al. Manipulation of molecular rotational dynamics with multiple laser pulses. Phys Chem Chem Phys. 2012;14(34):11994.
- Rouzee A, Hertz E, Lavorel B, et al. Towards the adaptive optimization of field-free molecular alignment. J. Phys. B-At. Mol. Opt. 2008;41(7):074002.
- Hertz E, Rouzee A, Guerin S, et al. Optimization of field-free molecular alignment by phase-shaped laser pulses. Phys Rev A. 2007;75(3):031403.
- Bisgaard CZ, Poulsen MD, Peronne E, et al. Observation of Enhanced Field-Free Molecular Alignment by Two Laser Pulses. Phys Rev Lett. 2004;92(17):173004.
- Lee KF, Litvinyuk IV, Dooley PW, et al. Two-pulse alignment of molecules. J. Phys. B-At. Mol. Opt. 2004;37(3):l43.
- Artamonov M, Seideman T. Optimal-control approach to field-free three-dimensional alignment of polyatomic molecules. Phys Rev A. 2010;82(2):023413.
- Viftrup SS, Kumarappan V, Trippel S, et al. Holding and Spinning Molecules in Space. Phys Rev Lett. 2007;99(14):143602.
- Lee KF, Villeneuve DM, Corkum PB, et al. Field-Free Three-Dimensional Alignment of Polyatomic Molecules. Phys Rev Lett. 2006;97:173001.
- Zhdanovich S, Milner AA, Bloomquist C, et al. Control of Molecular Rotation with a Chiral Train of Ultrashort Pulses. Phys Rev Lett. 2011;107(24):243004.
- Bloomquist C, Zhdanovich S, Milner AA, et al. Directional spinning of molecules with sequences of femtosecond pulses. Phys Rev A. 2012;86(6):063413.
- Lapert M, Hertz E, Guerin S, et al. Field-free permanent molecular planar alignment. Phys Rev A. 2009;80(5):051403.
- Abe H, Ohtsuki Y. Development of nonresonant optimal control simulation to include polarization effects of laser pulses. Chem Phys. 2012;400:13.
- Wells E, Rallis CE, Zohrabi M, et al. Adaptive strong-field control of chemical dynamics guided by three-dimensional momentum imaging. Nat Commun. 2013;4(1):2895.
- Alnaser AS, Kubel M, Siemering R, et al. Subfemtosecond steering of hydrocarbon deprotonation through superposition of vibrational modes. Nat Commun. 2014;5:3800.
- Blaga CI, Catoire F, Colosimo P, et al. Strong-field photoionization revisited. Nat Phys. 2009;5(5):335.
- Quan W, Lin Z, Wu M, et al. Classical Aspects in Above-Threshold Ionization with a Midinfrared Strong Laser Field. Phys Rev Lett. 2009;103:093001.
- Mancuso CA, Dorney KM, Hickstein DD, et al. Controlling Nonsequential Double Ionization in Two-Color Circularly Polarized Femtosecond Laser Fields. Phys Rev Lett. 2016;117(13):133201.
- Eckart S, Richter M, Kunitski M, et al. Nonsequential Double Ionization by Counterrotating Circularly Polarized Two-Color Laser Fields. Phys Rev Lett. 2016;117:133202.
- He LX, Lan PF, Le AT, et al. Real-Time Observation of Molecular Spinning with Angular High-Harmonic Spectroscopy. Phys Rev Lett. 2018;121:163201.
- Pinkham D, Jones RR. Intense laser ionization of transiently aligned CO. Phys Rev A. 2005;72:023418.
- Son S-K, Chu S-I. Theoretical study of orientation-dependent multiphoton ionization of polyatomic molecules in intense ultrashort laser fields: a new time-dependent Voronoi-cell finite difference method. Chem Phys. 2009;366:91.
- Larsen JJ, Wendt-Larsen I, Stapelfeldt H. Controlling the Branching Ratio of Photodissociation Using Aligned Molecules. Phys Rev Lett. 1999;83(6):1123.
- Weise F, Weber SM, Plewicki M, et al. Application of phase, amplitude, and polarization shaped pulses for optimal control on molecules. Chem Phys. 2007;332(2–3):313.
- Brixner T, Krampert G, Pfeifer T, et al. Quantum Control by Ultrafast Polarization Shaping. Phys Rev Lett. 2004;92(20):208301.
- Suzuki T, Minemoto S, Kanai T, et al. Optimal control of multiphoton ionization processes in aligned I2 Molecules with time-dependent polarization pulses. Phys Rev Lett. 2004;92(13):133005.
- Malik DA, Kimel AV, Kirilyuk A, et al. Coherent Control of Angular Momentum Transfer in Resonant Two-Photon Light-Matter Interaction. Phys Rev Lett. 2010;104:133001.
- Dudovich N, Oron D, Silberberg Y. Quantum Control of the Angular Momentum Distribution in Multiphoton Absorption Processes. Phys Rev Lett. 2004;92:103003.
- Wollenhaupt M, Krug M, Kohler J, et al. Photoelectron angular distributions from strong-field coherent electronic excitation. Appl. Phys . B-Lasers O. 2009;95(2):245.
- Pengel D, Kerbstadt S, Johannmeyer D, et al. Electron Vortices in Femtosecond Multiphoton Ionization. Phys Rev Lett. 2017;118:053003.
- Ramakrishna S, Seideman T. Torsional Control by Intense Pulses. Phys Rev Lett. 2007;99(10):103001.
- Madsen CB, Madsen LB, Viftrup SS, et al. Manipulating the Torsion of Molecules by Strong Laser Pulses. Phys Rev Lett. 2009;102(7):073007.
- Floss J, Grohmann T, Leibscher M, et al. Nuclear spin selective laser control of rotational and torsional dynamics. J Chem Phys. 2012;136(8):084309.
- Hansen JL, Nielsen JH, Madsen CB, et al. Control and femtosecond time-resolved imaging of torsion in a chiral molecule. J Chem Phys. 2012;136:204310.
- Strasfeld DB, Middleton CT, Zanni MT. Mode selectivity with polarization shaping in the mid-IR. New J Phys. 2009;11(10):105046.
- Sundstrom V. Femtobiology. Annu Rev Phys Chem. 2008;59:53.
- Hildner R, Brinks D, van Hulst NF. Femtosecond coherence and quantum control of single molecules at room temperature. Nat Phys. 2011;7(2):172.
- Bardeen CJ, Yakovlev VV, Squier JA, et al. Quantum Control of Population Transfer in Green Fluorescent Protein by Using Chirped Femtosecond Pulses. J Am Chem Soc. 1998;120(50):13023.
- Wohlleben W, Buckup T, Herek JL, et al. Coherent Control for Spectroscopy and Manipulation of Biological Dynamics. Chemphyschem. 2005;6(5):850.
- Lambert N, Chen YN, Cheng YC, et al. Quantum biology. Nat Phys. 2013;9(1):10.
- Shipp DW, Sinjab F, Notingher I. Raman spectroscopy: techniques and applications in the life sciences. Adv Opt Photonics. 2017;9:315.
- Krafft C, Schie IW, Meyer T, et al. Developments in spontaneous and coherent Raman scattering microscopic imaging for biomedical applications. Chem Soc Rev. 2016;45(7):1819.
- Freudiger CW, Min W, Saar BG, et al. Label-Free Biomedical Imaging with High Sensitivity by Stimulated Raman Scattering Microscopy. Science. 2008;322(5909):1857.
- Daum R, Brauchle EM, Berrio DAC, et al. Non-invasive detection of DNA methylation states in carcinoma and pluripotent stem cells using Raman microspectroscopy and imaging. Sci Rep. 2019;9(1):7014.
- Zhang X, Roeffaers MBJ, Basu S, et al. Label-Free Live-Cell Imaging of Nucleic Acids Using Stimulated Raman Scattering Microscopy. Chemphyschem. 2012;13:1054.
- Ji MB, Orringer DA, Freudiger CW, et al. Rapid, Label-Free Detection of Brain Tumors with Stimulated Raman Scattering Microscopy. Sci Transl Med. 2013;5:201ra119.
- Hoffman DP, Valley D, Ellis SR, et al. Optimally shaped narrowband picosecond pulses for femtosecond stimulated Raman spectroscopy. Opt Express. 2013;21:21685.
- Dudovich N, Oron D, Silberberg Y. Single-pulse coherently controlled nonlinear Raman spectroscopy and microscopy. Nature. 2002;418:512.
- Scully MO, Kattawar GW, Lucht RP, et al. FAST CARS: engineering a laser spectroscopic technique for rapid identification of bacterial spores. P. Natl Acad Sci Usa. 2002;99(17):10994.
- Freudiger CW, Min W, Holtom GR, et al. Highly specific label-free molecular imaging with spectrally tailored excitation-stimulated Raman scattering (STE-SRS) microscopy. Nat Photonics. 2011;5:103.
- Ogilvie JP, Debarre D, Solinas X, et al. Use of coherent control for selective two-photon fluorescence microscopy in live organisms. Opt Express. 2006;14(2):759.
- Tu HH, Liu Y, Turchinovich D, et al. Stain-free histopathology by programmable supercontinuum pulses. Nat Photonics. 2016;10:534.
- Wei L, Min W. Pump-probe optical microscopy for imaging nonfluorescent chromophores. Anal Bioanal Chem. 2012;403(8):2197.
- Flynn DC, Bhagwat AR, Ogilvie JP. Chemical-contrast imaging with pulse-shaping based pump-probe spectroscopy. Multiphoton Microscopy in the Biomedical Sciences Xiii. 2013;8588:85881z.
- Li B, Turinici G, Ramakrishna V, et al. Optimal dynamic discrimination of similar molecules through quantum learning control†. J Phys Chem B. 2002;106(33):8125.
- Roth M, Guyon L, Roslund J, et al. Quantum Control of Tightly Competitive Product Channels. Phys Rev Lett. 2009;102(25):253001.
- Rondi A, Kiselev D, Machado S, et al. Discriminating Biomolecules with Coherent Control Strategies. Chimia (Aarau). 2011;65(5):346.
- Petersen J, Mitric R, Bonacic-Koutecky V, et al. How Shaped Light Discriminates Nearly Identical Biochromophores. Phys Rev Lett. 2010;105(7):073003.
- Courvoisier F, Boutou V, Guyon L, et al. Discriminating bacteria from other atmospheric particles using femtosecond molecular dynamics. Photobio. A. 2006;180(3):300.
- Tseng C-H, Weinacht TC, Rhoades AE, et al. Using shaped ultrafast laser pulses to detect enzyme binding. Opt Express. 2011;19(24):24638.
- Rondi A, Bonacina L, Trisorio A, et al. Coherent manipulation of free amino acids fluorescence. Phys Chem Chem Phys. 2012;14(26):9317.
- Nie B, Saytashev I, Chong AD, et al. Biomed. Biomedical Optics Express. 2012;3:1750.
- Balu M, Saytashev I, Hou J, et al. Sub-40 fs, 1060-nm Yb-fiber laser enhances penetration depth in nonlinear optical microscopy of human skin. J Biomed Opt. 2015;20(12):120501.
- Xi P, Andegeko Y, Weisel LR, et al. Greater signal, increased depth, and less photobleaching in two-photon microscopy with 10fs pulses. Opt Commun. 2008;281:1841.
- Xi P, Andegeko Y, Pestov D, et al. Two-photon imaging using adaptive phase compensated ultrashort laser pulses. J Biomed Opt. 2009;14(1):014002.
- Pestov D, Andegeko Y, Lozovoy VV, et al. Pulse shaping for reducing photodamage in multiphoton microscopy. Multiphoton Microscopy in the Biomedical Sciences X. 2010;7569:756926.
- Walowicz KA, Pastirk I, Lozovoy VV, et al. Multiphoton Intrapulse Interference. 1. Control of Multiphoton Processes in Condensed Phases. J Phys Chem A. 2002;106(41):9369.
- Cruz JD, Lozovoy VV, Dantus M. Coherent control improves biomedical imaging with ultrashort shaped pulses. J. Photoch. Photobio. A. 2006;180:307.
- Cruz JD, Pastirk I, Comstock M, et al. Use of coherent control methods through scattering biological tissue to achieve functional imaging. P. Natl Acad Sci Usa. 2004;101(49):16996.
- Konar A, Lozovoy VV, Dantus M. Stimulated Emission Enhancement Using Shaped Pulses. J Phys Chem A. 2016;120:2002.
- Kawano H, Nabekawa Y, Suda A, et al. Attenuation of photobleaching in two-photon excitation fluorescence from green fluorescent protein with shaped excitation pulses. Biochem Biophys Res Commun. 2003;311(3):592.
- Midorikawa K, Chen JF, Kawano H, et al. Coherent control of multiphoton excitation process for biological fluorescence imaging. Commercial and Biomedical Applications of Ultrafast Lasers V. 2005;5714:99.
- Tkaczyk ER, Mauring K, Tkaczyk AH, et al. Control of the blue fluorescent protein with advanced evolutionary pulse shaping. Biochem Biophys Res Commun. 2008;376(4):733.
- Tkaczyk ER, Mignot A, Ye JY, et al. Increasing two-photon fluorescence signals by coherent control. Multiphoton Microscopy in the Biomedical Sciences VI. 2006;6089:608910.
- Tkaczyk ER, Tkaczyk AH, Mauring K, et al. Control of Two-photon Fluorescence of Common Dyes and Conjugated Dyes. J. Fluoresc. 2009;19(3):517.
- Savolainen J, Fanciulli R, Dijkhuizen N, et al. Controlling the efficiency of an artificial light-harvesting complex. Proc Natl Acad Sci U S A. 2008;105(22):7641.
- Yang D, Savolainen J, Jafarpour A, et al., 12th World Congress of the International Photodynamic Association: photodynamic Therapy: back to the Future 7380 (2009) p.73806h.
- Rhijn ACWV, Jafarpour A, Jurna M, et al. Coherent control of vibrational transitions: discriminating molecules in mixtures. Faraday Discuss. 2011;153:227.
- Edwards JR, Ruparel H, Ju JY. Mass-spectrometry DNA sequencing. Mutat. Res.-Fund. Mol. M. 2005;573(1–2):3.
- Tost J, Gut IG. DNA analysis by mass spectrometry—past, present and future. J Mass Spectrom. 2006;41(8):981.
- Calderaro A, Arcangeletti MC, Rodighiero I, et al. Identification of different respiratory viruses, after a cell culture step, by matrix assisted laser desorption/ionization time of flight mass spectrometry (MALDI-TOF MS). Sci Rep. 2016;6(1):36082.
- Kurata-Nishimura M, Ando Y, Kobayashi T, et al. Sequencing of Isotope-Labeled Small RNA Using Femtosecond Laser Ablation Time-of-Flight Mass Spectrometry. Appl. Phys. Expres. 2010;3:047002.
- Rethfeld B, Sokolowski-Tinten K, Linde DVD, et al. Timescales in the response of materials to femtosecond laser excitation. APPLIED PHYSICS A-MATERIALS SCIENCE & PROCESSING. 2004;79:767.
- Koji S. Progress in ultrafast laser processing and future prospects. Nanophotonics-Berlin. 2017;6(2):393.
- Spyridaki M, Koudoumas E, Tzanetakis P, et al. Temporal pulse manipulation and ion generation in ultrafast laser ablation of silicon. Appl Phys Lett. 2003;83(7):1474.
- Dachraoui H, Husinsky W. Thresholds of Plasma Formation in Silicon Identified by Optimizing the Ablation Laser Pulse Form. Phys Rev Lett. 2006;97:107601.
- Choi TY, Hwang DJ, Grigoropoulos CP. Femtosecond laser induced ablation of crystalline silicon upon double beam irradiation. Appl Surf Sci. 2002;197–198:720.
- Ihtesham HC, Xianfan X, Weiner AM. Ultrafast double-pulse ablation of fused silica. Appl Phys Lett. 2005;86(15):151110.
- Preuss S, Spath M, Zhang Y, et al. Time resolved dynamics of subpicosecond laser ablation. Appl Phys Lett. 1993;62:3049.
- Deng YP, Xie XH, Xiong H, et al. Optical breakdown for silica and silicon with double femtosecond laser pulses. Opt Express. 2005;13(8):3096.
- Han Z, Zhou C, Dai E, et al. Ultrafast double pulses ablation of Cr film on glass. Opt Commun. 2008;281(18):4723.
- Babushok VI, Jr FCD, Gottfried JL, et al. Double pulse laser ablation and plasma: laser induced breakdown spectroscopy signal enhancement. Spectrochimica Acta Part B. 2006;61(9):999.
- Semerok A, Dutouquet C. Ultrashort double pulse laser ablation of metals. Thin Solid Films. 2004;453–454:501.
- Zhang Z, Rompay PAV, Pronkoa PP. Ion characteristics of laser-produced plasma using a pair of collinear femtosecond laser pulses. Appl Phys Lett. 2003;83(3):431.
- Singha S, Hu Z, Gordon RJ. Ablation and plasma emission produced by dual femtosecond laser pulses. J Appl Phys. 2008;104(11):113520.
- Hommes V, Miclea M, Hergenröder R. Silicon surface morphology study after exposure to tailored femtosecond pulses. Appl Surf Sci. 2006;252(20):7449.
- Klini A, Loukakos PA, Gray D, et al. Laser Induced Forward Transfer of metals by temporally shaped femtosecond laser pulses. Opt Express. 2008;16(15):11300.
- Noel S, Hermann J. Reducing nanoparticles in metal ablation plumes produced by two delayed short laser pulses. Appl Phys Lett. 2009;94:053120.
- Noel S, Axente E, Hermann J. Investigation of plumes produced by material ablation with two time-delayed femtosecond laser pulses. Appl Surf Sci. 2009;255(24):9738.
- Wang QX, Luo SZ, Chen Z, et al. Drilling of aluminum and copper films with femtosecond double-pulse laser. Opt Laser Technol. 2016;80:116.
- Stoian R, Boyle M, Thoss A, et al. Laser ablation of dielectrics with temporally shaped femtosecond pulses. Applied Physics Letter. 2002;80(3):353.
- Stoian R, Boyle M, Thoss A, et al. Dynamic temporal pulse shaping in advanced ultrafast laser material processing. APPLIED PHYSICS A-MATERIALS SCIENCE & PROCESSING. 2003;77:265.
- Burakov IM, Bulgakova NM, Stoian R, et al. Theoretical investigations of material modification using temporally shaped femtosecond laser pulses. Appl Phys A-Mater. 2005;81:1639.
- Stoian R, Mermillod-Blondin A, Bulgakova NM, et al. Optimization of ultrafast laser generated low-energy ion beams from silicon targets. Appl Phys Lett. 2005;87(12):124105.
- Hergenroder R, Miclea M, Hommes V. Controlling semiconductor nanoparticle size distributions with tailored ultrashort pulses. Nanotechnology. 2006;17(16):4065.
- Colombier JP, Combis P, Rosenfeld A, et al. Optimized energy coupling at ultrafast laser-irradiated metal surfaces by tailoring intensity envelopes: consequences for material removal from Al samples. Phys Rev B. 2006;74(22):224106.
- Gunaratne T, Kangas M, Singh S, et al. Influence of bandwidth and phase shaping on laser induced breakdown spectroscopy with ultrashort laser pulses. Chem Phys Lett. 2006;423:197.
- Hu Z, Singha S, Gordon RJ. Controlling the photoluminescence of gallium arsenide with trains of ultrashort laser pulses. Phys Rev B. 2010;82(11):115204.
- Singha S, Hu Z, Gordon RJ. Closed Loop Coherent Control of Electronic Transitions in Gallium Arsenide. J Phys Chem A. 2011;115(23):6093.
- Garrelie F, Bourquard F, Loir AS, et al. [INVITED] Control of femtosecond pulsed laser ablation and deposition by temporal pulse shaping. Opt Laser Technol. 2016;78:42.
- Jiang L, Tsai HL. Repeatable nanostructures in dielectrics by femtosecond laser pulse trains. Appl Phys Lett. 2005;87(15):151104.
- Jiang L, Tsai H-L. Energy Transport and Nanostructuring of Dielectrics by Femtosecond Laser Pulse Trains. J. Heat Trans.-T. Asme. 2006;128:926.
- Jiang L, Tsai H-L. Modeling of ultrashort laser pulse-train processing of metal thin films. Int. J. Heat Mass Tran. 2007;50(17–18):3461.
- Englert L, Rethfeld B, Haag L, et al. Control of ionization processes in high band gap materials via tailored femtosecond pulses. Opt Express. 2007;15(26):17855.
- Englert L, Wollenhaupt M, Haag L, et al. Material processing of dielectrics with temporally asymmetric shaped femtosecond laser pulses on the nanometer scale. Appl. Phys. A-Mater. 2008;92(4):749.
- Uchugonova A, Zhang HJ, Lemke C, et al. Nanosurgery with near-infrared 12-femtosecond and picosecond laser pulses. Multiphoton Microscopy in the Biomedical Sciences Xi. 2011;7903:79031n.
- Vorobyev AY, Guo CL. Direct femtosecond laser surface nano/microstructuring and its applications. Laser Photonics Rev. 2013;7:385.
- Her T-H, Finlay RJ, Wu C, et al. Microstructuring of silicon with femtosecond laser pulses. Appl Phys Lett. 1998;73(12):1673.
- Castleman AW, Bowen KH. Clusters: structure, Energetics, and Dynamics of Intermediate States of Matter. J Phys Chem. 1996;100:12911.
- Tsuji T, Watanabe N, Tsuji M. Laser induced morphology change of silver colloids: formation of nano-size wires. Appl Surf Sci. 2003;211(1–4):189.
- Nichols WT, Sasaki T, Koshizaki N. Laser ablation of a platinum target in water. II. Ablation rate and nanoparticle size distributions. J Appl Phys. 2006;100:114912.
- Lee J, Lee M. Laser-Induced Conversion of Au Powders to Highly Stable Nanoparticles with a Narrow Size Distribution. J Phys ChemC. 2016;120(24):13256.
- Suzuki K, Tanaka N, Ando A, et al. Size-selected copper oxide nanoparticles synthesized by laser ablation. J Nanopart Res. 2012;14:863.
- Vuckovic S, Svanqvist M, Popok VN. Laser ablation source for formation and deposition of size-selected metal clusters. Rev Sci Instrum. 2008;79:073303.
- Tsakiris N, Anoop KK, Ausanio G, et al. Ultrashort laser ablation of bulk copper targets: dynamics and size distribution of the generated nanoparticles. J Appl Phys. 2014;115(24):243301.
- Kobayashi T, Kato T, Matsuo Y, et al. Wavelength-dependent fragmentation and clustering observed after femtosecond laser ablation of solid C60. J Chem Phys. 2007;127(11):111101.
- Doppner T, Fennel T, Diederich T, et al. Controlling the Coulomb Explosion of Silver Clusters by Femtosecond Dual-Pulse Laser Excitation. Phys Rev Lett. 2005;94(1):013401.
- Martchenko T, Siedschlag C, Zamith S, et al. Optimal control of femtosecond laser–cluster interactions. Phys Rev A. 2005;72(5):053202.
- Link S, Burda C, Nikoobakht B, et al. Laser-Induced Shape Changes of Colloidal Gold Nanorods Using Femtosecond and Nanosecond Laser Pulses. J Phys Chem B. 2000;104:6152.
- Guillermin M, Liebig C, Garrelie F, et al. Adaptive control of femtosecond laser ablation plasma emission. Appl Surf Sci. 2009;255:5163.
- Bulgakov AV, Ozerov I, Marine W. Silicon clusters produced by femtosecond laser ablation: non-thermal emission and gas-phase condensation. Appl. Phys. A-Mater. 2004;79(4–6):1591.
- Siders CW, Cavalleri A, Sokolowski-Tinten K, et al. Detection of Nonthermal Melting by Ultrafast X-ray Diffraction. Science. 1999;286:1340.
- Sokolowski-Tinten K, Solis J, Bialkowski J, et al. Dynamics of Ultrafast Phase Changes in Amorphous GeSb Films. Phys Rev Lett. 1998;81:3679.
- Lindenberg AM, Larsson J, Sokolowski-Tinten K, et al. Atomic-Scale Visualization of Inertial Dynamics. Science. 2005;308(5720):392.
- Sokolowski-Tinten K, Blome C, Dietrich C, et al. Femtosecond X-Ray Measurement of Ultrafast Melting and Large Acoustic Transients. Phys Rev Lett. 2001;87(22):225701.
- Katz O, Natan A, Silberberg Y, et al. Standoff detection of trace amounts of solids by nonlinear Raman spectroscopy using shaped femtosecond pulses. Appl Phys Lett. 2008;92(17):171116.
- Rasskazov G, Ryabtsev A, Dantus M. Eye-safe near-infrared trace explosives detection and imaging. Opt Express. 2017;25:5832.
- Glenn R, Dantus M. Single Broadband Phase-Shaped Pulse Stimulated Raman Spectroscopy for Standoff Trace Explosive Detection. J Phys Chem Lett. 2016;7:117.
- Bremer MT, Dantus M. Standoff explosives trace detection and imaging by selective stimulated Raman scattering. Appl Phys Lett. 2013;103:061119.
- Braun A, Korn G, Liu X, et al. Self-channeling of high-peak-power femtosecond laser pulses in air. Opt Lett. 1995;20(1):73.
- Kasparian J, Rodriguez M, Mejean G, et al. White-Light Filaments for Atmospheric Analysis. Science. 2003;301(5629):61.
- Mejean G, Kasparian J, Yu J, et al. Remote detection and identification of biological aerosols using a femtosecond terawatt lidar system. Appl. Phys. B-Lasers O. 2004;78(5):535.
- Brixner T, Damrauer NH, Niklaus P, et al. Photoselective adaptive femtosecond quantum control in the liquid phase. Nature. 2001;414(6859):57.
- Calegari F, Vozzi C, Gasilov S, et al. Rotational Raman Effects in the Wake of Optical Filamentation. Phys Rev Lett. 2008;100:123006.
- Cai H, Wu J, Peng Y, et al. Comparison study of supercontinuum generation by molecular alignment of N2 and O2. Opt Express. 2009;17:5822.
- Cai H, Wu J, Li H, et al. Elongation of femtosecond filament by molecular alignment in air. Opt Express. 2009;17(23):21060.
- Chen AM, Li SY, Qi HX, et al. Elongation of plasma channel generated by temporally shaped femtosecond laser pulse. Opt Commun. 2017;93:144.
- Varma S, Chen YH, Milchberg HM. Trapping and Destruction of Long-Range High-Intensity Optical Filaments by Molecular Quantum Wakes in Air. Phys Rev Lett. 2008;101:205001.
- Wu J, Cai H, Lu PF, et al. Intense ultrafast light kick by rotational Raman wake in atmosphere. Appl Phys Lett. 2009;95(22):221502.
- Joshi D, Kumar D, Maini AK, et al. Detection of biological warfare agents using ultra violet-laser induced fluorescence LIDAR. Spectrochim Acta A. 2013;112:446.
- Kaushal H, Kaddoum G. Applications of Lasers for Tactical Military Operations. IEEE Access. 2017;5:20736.
- Valerie CC. The Challenges Facing Submarine Optical Communications. Opt Photonics News. 2014;25:28.
- Smitha P, Singh I, Najim M, et al. Development of thin broad band radar absorbing materials using nanostructured spinel ferrites. Sci.-Mater. El. 2016;27–7731.
- Shen ZZ, Chen JH, Li B, et al. Recent progress in SiC nanowires as electromagnetic microwaves absorbing materials. J Alloy Compd. 2020;815:152388.
- Hernandez-Rueda J, Gotte N, Siegel J, et al. Nanofabrication of Tailored Surface Structures in Dielectrics Using Temporally Shaped Femtosecond-Laser Pulses. ACS Appl Mater Inter. 2015;7(12):6613–6619.
- Fraggelakis F, Stratakis E, Loukakos PA. Control of periodic surface structures on silicon by combined temporal and polarization shaping of femtosecond laser pulses. Appl Surf Sci. 2018;444:154–160.
- Deng JN, Qi HX, Zhao L, et al. Control of ablation morphology on Cu film with tailored femtosecond pulse trains. Appl. Phys. A-Mater. 2020;126(6):425. DOI: https://doi.org/10.1007/s00339-020-03589-0.
- Qi Y, Qi HX, Wang QX, et al. The influence of double pulse delay and ambient pressure on femtosecond laser ablation of silicon. Opt Laser Technol. 2015;66:68.
- Iam Choon K. Nonlinear Organic Liquid-Cored Fiber Array for All-Optical Switching and Sensor Protection Against Short-Pulsed Lasers. IEEE J. Sel. Top. Quant. 2008;14:946.
- Qi Y, Qi HX, Chen AM, et al. Improvement of aluminum drilling efficiency and precision by shaped femtosecond laser. Appl Surf Sci. 2014;317:252.
- Wang C, Fomovsky M, Miao GX, et al. Femtosecond laser crosslinking of the cornea for non-invasive vision correction. Nat Photonics. 2018;12(7):416.
- Soong HK, Malta JB. Femtosecond Lasers in Ophthalmology. Am J Ophthalmol. 2009;147(2):189.
- Mourou G, Brocklesby B, Tajima T, et al. The future is fibre accelerators. Nat Photonics. 2013;7:258.
- Breitkopf S, Eidam T, Klenke A, et al. Stack and dump: peak-power scaling by coherent pulse addition in passive cavities. Eur. Phys. J.-Spec. Top. 2015;224(13):2573.
- Wille H, Rodriguez M, Kasparian J, et al. Teramobile: a mobile femtosecond-terawatt laser and detection system. Eur. Phys. J.-Appl. Phys. 2002;20(3):183.
- Dicaire I, Jukna V, Praz C, et al. Spaceborne laser filamentation for atmospheric remote sensing. Laser Photonics Rev. 2016;10(3):481.