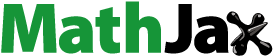
ABSTRACT
Development of fabrication technologies for three-dimensional structuring and integration of nanomaterials in devices is important for a broad range of applications, including next-generation high energy density batteries, super(de)wetting and biomedical coatings, and miniaturized biomedical diagnostics. Amongst various nanofabrication approaches, the flame synthesis route accounts for some of the first man-made nanomaterials and industrial production of various nanoparticle commodities such as carbon black, fumed silica, and pigmentary titania. In the past two decades, flexibility in nanomaterials and facile fabrication of nanostructured films by aerosol self-assembly has motivated the exploration of this technology for device applications. In this review, we present a perspective of recent progress in flame-assisted nanofabrication and its application to emerging technologies. The fundamentals of flame synthesis will be briefly reviewed to evaluate trends in flame reactor designs and directions for improvements. A selection of exemplary flame-made nanostructures will be presented across the major categories of catalysis, energy conversion devices, membranes and sensors, highlighting weakness and strengths of this synthesis route. We will conclude with an outlook towards possible implementation of flame-assisted self-assembly as a scalable tool for nanofabrication in emerging devices and a critical assessment of the persisting challenges for its broader industrial uptake.
Graphical Abstract
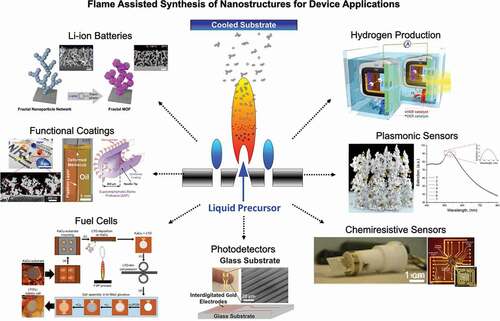
1. Introduction
Recent progress in nanomanufacturing is leading the transformation of our information, defence, energy, and healthcare technologies, with nanomaterials having become critical building blocks for the next generation of microprocessors, optoelectronic devices, solar cells, high-capacity batteries, and biomedical sensors. In the past decades, silicon-based manufacturing has been dominant, ranging from the intricate design of CPUs with a size of a few cm2 to the scale of km-wide photovoltaic solar farms. However, the feasibility of integrating various types of nanomaterials, and in particular catalysts, in devices is significantly less advanced, resulting in slow progress of critically needed technologies such as electrolysers for hydrogen production, point-of-care, and portable biomedical sensors, and high energy density storage batteries. This is particularly challenging as, in addition to the vast range of potentially useful material compositions, it is often necessary to reproducibly fabricate non-planar mesoporous architectures with a hierarchy of functionality across multiple length scales. The three-dimensional self-assembly of porous textures of nanomaterials by the deposition of nanoparticle-loaded aerosols is increasingly considered a powerful approach for the fabrication and integration of non-silicon-based nanomaterials in devices.
Amongst emerging fabrication technologies for the generation of nanoparticle aerosols, the flame synthesis route provides some distinct features including a large range of feasible material compositions, one-step synthesis process, high scalability, and production rate [Citation1]. Flame synthesis has a long implementation history accounting for both the first man-made nanomaterials with the reported production of nanopigments in sooting flames already in 3rd century BC and the first large-scale production of nanoparticle commodities, including carbon black, fumed silica, pigmentary titania, and P25 catalysts [Citation2]. While original application of flame synthesis has been almost entirely dedicated to the production of nanoparticle powders [Citation2], the direct integration of flame-made materials in devices has rapidly advanced in the last decades [Citation3], demonstrating potential for numerous applications [Citation4], including fuel cells [Citation5], chemical [Citation6,Citation7] and light-sensing [Citation8], (photo)electrocatalysis [Citation9], biomedical [Citation10] and super-hydrophilic/hydrophobic coatings [Citation11,Citation12].
First attempts to use flames for the fabrication of nanotextured layers have been largely related to the evolution of thermal spray reactors [Citation13], with application mostly in the deposition of biocompatible and decorative coatings. Latter progress in flame synthesis reactor design partially converged to a majority of systems using liquid precursors as a convenient and flexible feedstock for the desired nanomaterials [Citation1]. A major distinction between liquid-fed flame synthesis reactors can be made as a function of whether the reaction enthalpy is mainly provided via the precursor solution, often referred to as flame spray pyrolysis (FSP), or by a supporting flame, often cited as liquid-fed spray flame synthesis [Citation1]. Recently major research efforts have been dedicated to understand the nucleation and growth process of various materials within their flight in the flame [Citation14] and ensuing hot aerosols as well as the formation of porous layers by the self-assembly of the nanoparticle aerosols on target substrates [Citation3,Citation15,Citation16]. The establishment of commercial flame reactor designs has also significantly progressed, resulting in reproducible low-cost fabrication tools broadly available in various research centres, and increasingly more in industrial manufacturing centres.
In this review article, we will attempt to provide a broad and in-depth introduction to the flame-assisted synthesis of nanomaterials for integration in devices. We start by summarizing the key fundamental mechanisms controlling the nucleation of nanoparticle aerosols in liquid-fed flames and the self-assembly of three-dimensionally nanostructured films by deposition of these flame-made aerosols. We will review recent liquid-fed flame reactor designs highlighting fundamental components and their evolution. A selection of applications of flame-assisted synthesis for the integration of nanomaterials in devices will be presented across the following categories, namely catalysis, energy conversion devices, membranes, coatings, and sensors. The articles in this review were chosen based on specific keywords such as ‘flame synthesis, aerosol self-assembly, nanoparticle networks, three-dimensional structuring, device integration, catalysis, fuel cell, batteries, hydro-phobic/phillic, coatings, membranes, chemiresistive, plasmonic, photodetector, and sensors’. The list was then further refined by selecting the top 10 cited manuscripts to show diverse aerosol nanoparticles and mixed-matrix systems synthesized using FSP for a plethora of device applications. Lastly, we will conclude with an outlook to the future potential development of flame-assisted synthesis as a scalable tool for the industrial fabrication of three-dimensional structured nanomaterials in devices, highlighting the strength and shortcomings of current flame synthesis technologies.
2. Fundamentals of flame synthesis
2.1. Nanoparticle aerosol generation
summarizes a simplified mechanism of nanoparticle and aerosol formation via liquid-fed flame synthesis. A liquid precursor solution is usually atomized into fine droplets with average diameter ranging from few to hundreds of micrometres. These droplets can thereafter either undergo full evaporation/pyrolysis before nucleation of the target materials (vapour-to-cluster route) or be only partially evaporated (droplet-to-cluster route). In the former vapour-to-cluster route a saturated or super-saturated vapour of the target materials is formed. Insufficient vapour saturation does not allow nucleation of the cluster in the gas-phase, and nanomaterials may heterogeneously nucleate from a substrate into a variety of morphologies including nanowires. Such nanowires have been reported to be utilized for their controlled wetting properties. For instance, Tricoli et al. demonstrated the synthesis of anti-fogging coatings composed of mixed SiO2-TiO2 nanowire films using direct flame aerosol deposition on glass substrates [Citation11,Citation17]. The process of vapour-to-cluster shares some similarity to chemical vapour deposition (CVD) from metal organic (MO) precursors [Citation18]. In contrast, if sufficient super-saturation is achieved during evaporation of the atomized precursor solution, clusters nucleate homogeneously in the gas phase and growth by Brownian coagulation and condensation, resulting in the formation of a nanoparticle aerosol. The latter aerosols feature different levels of particle agglomeration as a function of the extent of coalescence induced by the particle concentration, residence time, and temperature profile of the aerosol. Aerosols consisting of fractal-like agglomerates comprised of primary nanoparticles are often obtained at common liquid-fed spray flame operation conditions.
Figure 1. Simplified mechanism of nanoparticle aerosol formation via flame synthesis. Under the vapour-to-cluster route, the atomized liquid precursor undergoes full evaporation and nucleation to form nanoparticle aerosols/nanowires. Under droplet-to-cluster route, the atomized liquid precursor undergoes partial evaporation leading to incomplete nucleation resulting in the formation of large or hollow particle aerosols
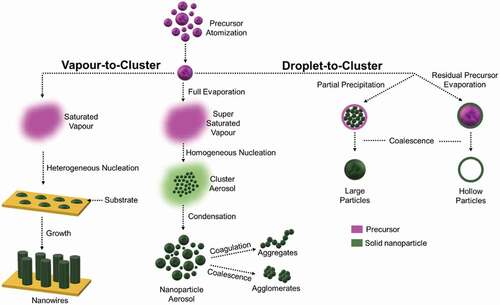
Figure 2. Different types of burners (a, b) and atomizer (c) employed in flame spray setups. (a) Flame Spray Pyrolysis. (b) Hydrogen-Oxygen Flame. (c) Ultrasonic atomizer
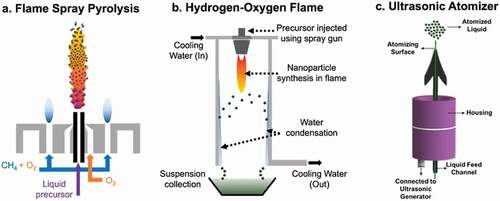
Figure 3. Effect of deposition temperature and regime on the film morphology obtainable by self-assembly of aerosol nanoparticles. High sintering rate leads to simultaneous deposition and coalescence of aerosol particles resulting in a wide range of porous (~12%–80%) nanostructures. Low sintering rate of self-assembly can proceed either through Brownian diffusion, randomly oriented ballistic deposition, or ballistic motion resulting in films with ~98% to ~86% average porosity
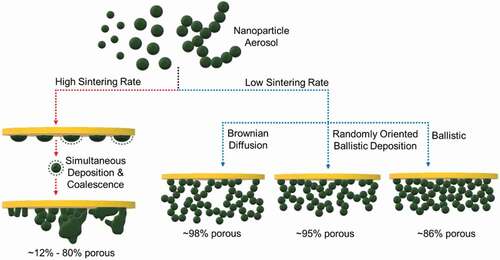
Figure 4. SEM image of (a) TiO2 films deposited at 4.1 cm resulting in granular morphology. (b) TiO2 films deposited at 1.7 cm resulting in columnar morphology
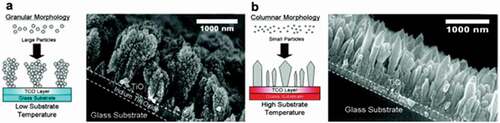
Figure 5. (a) Limiting porosities as a number of primary particles in an agglomerate. (b) Limiting porosities as a function of fractal dimension (Df). (c) Representive slice packing density profiles in the ballistic diffusion regime. (d) Structures of aerosol deposited formed by filtration of nanoparticles (50 nm) for Pe = 0.01–10. (e) Solid volume fraction profile for Pe = 10 and Rc = 1, 2, 4 μm. (f) Top-view SEM of SnO2 nanoparticle aerosol film deposited using FSP with 98% porosity. Reprinted with permission [Citation3,Citation15,Citation16,Citation55]
![Figure 5. (a) Limiting porosities as a number of primary particles in an agglomerate. (b) Limiting porosities as a function of fractal dimension (Df). (c) Representive slice packing density profiles in the ballistic diffusion regime. (d) Structures of aerosol deposited formed by filtration of nanoparticles (50 nm) for Pe = 0.01–10. (e) Solid volume fraction profile for Pe = 10 and Rc = 1, 2, 4 μm. (f) Top-view SEM of SnO2 nanoparticle aerosol film deposited using FSP with 98% porosity. Reprinted with permission [Citation3,Citation15,Citation16,Citation55]](/cms/asset/c34050e1-9466-4900-9cd9-a1f96e252d36/tapx_a_1997153_f0005_oc.jpg)
Figure 6. (a) Simplified schematic illustration of the photo-catalysis mechanism. (b) H2 production from methanol reformation using different TiO2 samples. (c) Oxygen evolution of aqueous modified BiVO4 under visible light irradiation (λ = 420 nm). (d) Yield of CO produced after CO2 reduction using ZnO/CeO2 nanocomposites (e) Yield of CH4 produced after CO2 reduction using ZnO/CeO2 nanocomposites. (f) Absorbance spectra of methyl Orange with La2Ti2O7 nanoparticles treated at 1000°C/3 h/air after UV irradiation. Reprinted with permission [Citation93–96]
![Figure 6. (a) Simplified schematic illustration of the photo-catalysis mechanism. (b) H2 production from methanol reformation using different TiO2 samples. (c) Oxygen evolution of aqueous modified BiVO4 under visible light irradiation (λ = 420 nm). (d) Yield of CO produced after CO2 reduction using ZnO/CeO2 nanocomposites (e) Yield of CH4 produced after CO2 reduction using ZnO/CeO2 nanocomposites. (f) Absorbance spectra of methyl Orange with La2Ti2O7 nanoparticles treated at 1000°C/3 h/air after UV irradiation. Reprinted with permission [Citation93–96]](/cms/asset/5273702a-89e6-41b3-af89-f183110d9a87/tapx_a_1997153_f0006_oc.jpg)
Figure 7. (a) Schematic representation of H2 production using (photo)electrochemical catalytic mechanism. (b) Dependence of photocurrent-time plots for BiVO4 and BiVO4-rGO electrodes (Inset: photocurrent transient response curve at a constant potential). (c) Photocurrent density of CuO/ITO electrodes at different sintering temperatures. (d) Cyclic voltammogram of 1st, 300th, 600th, and 1000th cycles for 5 s deposited Co3O4 nano-islands. (e) Long-term electro-catalytic testing at a constant current density of 10 mA/cm2 for the 5 s deposited Co3O4 nano-islands. (f) Cross-sectional SEM micrograph depicting flame made BiVO4 photoanodes at different heights above burner: 15 cm, 10 cm, and 6 cm showcasing different porosities. Reprinted with permission [Citation9,Citation60,Citation98,Citation100]
![Figure 7. (a) Schematic representation of H2 production using (photo)electrochemical catalytic mechanism. (b) Dependence of photocurrent-time plots for BiVO4 and BiVO4-rGO electrodes (Inset: photocurrent transient response curve at a constant potential). (c) Photocurrent density of CuO/ITO electrodes at different sintering temperatures. (d) Cyclic voltammogram of 1st, 300th, 600th, and 1000th cycles for 5 s deposited Co3O4 nano-islands. (e) Long-term electro-catalytic testing at a constant current density of 10 mA/cm2 for the 5 s deposited Co3O4 nano-islands. (f) Cross-sectional SEM micrograph depicting flame made BiVO4 photoanodes at different heights above burner: 15 cm, 10 cm, and 6 cm showcasing different porosities. Reprinted with permission [Citation9,Citation60,Citation98,Citation100]](/cms/asset/9df52a3b-9508-4203-b0e3-28dd174ffe82/tapx_a_1997153_f0007_oc.jpg)
Figure 8. (a) Operational illustration of a thermochemical catalytic reactor. (b) H2 and CO production rates during 100 cycles of CO2 splitting using 3% Ce-Mn3O4. (c) H2 and CO yield produced using 3% Ce-Mn3O4. (d) Methanol production for different materials. (e) CO oxidation of different Hopcalite nanoparticles synthesized from nitrates through inverse micro-emulsions. (f) H2/CO ratio produced using 10 wt.% Ni supported Si/CeZr synthesized using D-FSP. Reprinted with permission [Citation103–106]
![Figure 8. (a) Operational illustration of a thermochemical catalytic reactor. (b) H2 and CO production rates during 100 cycles of CO2 splitting using 3% Ce-Mn3O4. (c) H2 and CO yield produced using 3% Ce-Mn3O4. (d) Methanol production for different materials. (e) CO oxidation of different Hopcalite nanoparticles synthesized from nitrates through inverse micro-emulsions. (f) H2/CO ratio produced using 10 wt.% Ni supported Si/CeZr synthesized using D-FSP. Reprinted with permission [Citation103–106]](/cms/asset/c84899be-9d08-4aa0-9741-e1454ffa98bf/tapx_a_1997153_f0008_oc.jpg)
Figure 9. (a) Simplified schematic representation of a fuel cell. (b) SEM micrograph representing a cross-sectional view of anode-electrolyte and cathode-electrolyte interface. (c) Arrhenius plots of ionic conductivity of Ce1-xGdxO2-x/2. (d) TEM micrograph of flame-made Ce1-xGdxO2-x/2. (e) Methanol oxidation and (f) CO stripping reactions by FSP synthesized carbon supported Pt-Ru catalyst compared to commercial E-TEK catalyst. Reprinted with permission [Citation5,Citation109,Citation110]
![Figure 9. (a) Simplified schematic representation of a fuel cell. (b) SEM micrograph representing a cross-sectional view of anode-electrolyte and cathode-electrolyte interface. (c) Arrhenius plots of ionic conductivity of Ce1-xGdxO2-x/2. (d) TEM micrograph of flame-made Ce1-xGdxO2-x/2. (e) Methanol oxidation and (f) CO stripping reactions by FSP synthesized carbon supported Pt-Ru catalyst compared to commercial E-TEK catalyst. Reprinted with permission [Citation5,Citation109,Citation110]](/cms/asset/355000be-a072-4a9d-9f1c-cb12ab7689c1/tapx_a_1997153_f0009_oc.jpg)
Figure 10. (a) Schematic mechanism of charge/discharge in a Li-ion battery. (b) Stepwise fabrication of LTO electrode and battery cell. (c) Charge/discharge curves of LTO batteries. (d) Charge/Discharge curves NCM111. (e) Schematic representation of peeling off strategy facilitated by self-supported ZIF-8 membrane for Li-S battery. (f) Electrochemical Impedance Spectrum depicting ionic conductivity and blocking effect. Reprinted with permission [Citation111–113]
![Figure 10. (a) Schematic mechanism of charge/discharge in a Li-ion battery. (b) Stepwise fabrication of LTO electrode and battery cell. (c) Charge/discharge curves of LTO batteries. (d) Charge/Discharge curves NCM111. (e) Schematic representation of peeling off strategy facilitated by self-supported ZIF-8 membrane for Li-S battery. (f) Electrochemical Impedance Spectrum depicting ionic conductivity and blocking effect. Reprinted with permission [Citation111–113]](/cms/asset/6b39ec48-c2b1-42db-b2aa-9ef47d4ec0fb/tapx_a_1997153_f0010_oc.jpg)
Figure 11. (a) Representation of a liquid drop on a (super)hydrophilic surface. (b) Flame deposited SiO2 nanofiber film. (c) Antifogging property demonstrated by flame-made pure SiO2, TiO2, and mixed silica-titania nanoparticle layers. (d) Anti-fogging property of SiO2 nanoparticles studied at different time intervals. (e) SEM micrograph of FSP deposited SiO2 coating on PC board. (f) Water contact angle measurement for different samples under dark and solar radiations. Reprinted with permission [Citation11,Citation121–123]
![Figure 11. (a) Representation of a liquid drop on a (super)hydrophilic surface. (b) Flame deposited SiO2 nanofiber film. (c) Antifogging property demonstrated by flame-made pure SiO2, TiO2, and mixed silica-titania nanoparticle layers. (d) Anti-fogging property of SiO2 nanoparticles studied at different time intervals. (e) SEM micrograph of FSP deposited SiO2 coating on PC board. (f) Water contact angle measurement for different samples under dark and solar radiations. Reprinted with permission [Citation11,Citation121–123]](/cms/asset/8841b4ec-96c2-48be-b591-2c181bb8da57/tapx_a_1997153_f0011_oc.jpg)
Figure 12. (a) Schematic representation of a liquid drop on a (super)hydrophobic surface. (b) Contact water angle was demonstrated by 2 μl water (left) and 5 μl hexadecane drop (right) on superamphiphobic substrate. (c) RMS roughness test as a function of number of revolutions after Taber wheel test on flame made TiO2 and SiO2 surfaces. (d) SEM micrograph of TiO2 nanoparticles deposited using FSP demonstrates water contact angle of 163°. (e) Schematic illustration of growth mechanism of omnidirectional transparent super-oleophobic nanostructure. (f) Optical image representing water, n-tetradecane, sunflower oil, and ethylene glycol on super-oleophobic surface. (g) Detailed schematic representing synthesis of SAP (h): SAP (left) and bare needle (right) dipped in tetradecane oil displaying contamination-free property. Reprinted with permission [Citation12,Citation125–128]
![Figure 12. (a) Schematic representation of a liquid drop on a (super)hydrophobic surface. (b) Contact water angle was demonstrated by 2 μl water (left) and 5 μl hexadecane drop (right) on superamphiphobic substrate. (c) RMS roughness test as a function of number of revolutions after Taber wheel test on flame made TiO2 and SiO2 surfaces. (d) SEM micrograph of TiO2 nanoparticles deposited using FSP demonstrates water contact angle of 163°. (e) Schematic illustration of growth mechanism of omnidirectional transparent super-oleophobic nanostructure. (f) Optical image representing water, n-tetradecane, sunflower oil, and ethylene glycol on super-oleophobic surface. (g) Detailed schematic representing synthesis of SAP (h): SAP (left) and bare needle (right) dipped in tetradecane oil displaying contamination-free property. Reprinted with permission [Citation12,Citation125–128]](/cms/asset/8b55bc35-46d3-46fa-b2f2-355e014cf75e/tapx_a_1997153_f0012_oc.jpg)
Figure 13. (a) Schematic description of introduction of FSP synthesized nanomaterials into the cell for TERM applications. (b) Real-time Reverse Transcription Polymerase Chain Reaction (RT-PCR) analysis of osteocalcin indicating up-regulation in cell culture in the presence of Sr-doped glass nanoparticles. (c) SEM micrograph depicting interaction between cell and coating after 14 days on the ultraporous neural network and spin-coated surface. (d) Immunostaining analysis of F-Actin and Osteocalcin. Reprinted with permission [Citation10,Citation132]
![Figure 13. (a) Schematic description of introduction of FSP synthesized nanomaterials into the cell for TERM applications. (b) Real-time Reverse Transcription Polymerase Chain Reaction (RT-PCR) analysis of osteocalcin indicating up-regulation in cell culture in the presence of Sr-doped glass nanoparticles. (c) SEM micrograph depicting interaction between cell and coating after 14 days on the ultraporous neural network and spin-coated surface. (d) Immunostaining analysis of F-Actin and Osteocalcin. Reprinted with permission [Citation10,Citation132]](/cms/asset/e869078f-9e14-4d69-a662-fbe7b2664b7b/tapx_a_1997153_f0013_oc.jpg)
Figure 14. (a) Schematic summary of different antimicrobial mechanisms facilitated by FSP synthesized nanomaterials. (b) Scanning Transmission Electron Microscopy (STEM) micrograph of Ag/SiO2 nanoparticles synthesized using silver acetate/HDMSO precursor solution. (c) Scanning Transmission Electron Microscopy (STEM) micrograph of Ag/SiO2 nanoparticles synthesized using silver nitrate/HDMSO precursor solution. (d) SEM micrograph of PET paper sample before (inset) and after coating of Ag NPs. (e) Study of antimicrobial property of Ag NPs coated on PET paper against E. coli. (f) Digital image of crystal violet stained uncoated and TiO2 and P25 coated aluminium substrates with and without UV irradiation. (f) Spectrophotometric study (at 595 nm) of biofilm mass quantification using crystal violet staining. Reprinted with permission [Citation133–135]
![Figure 14. (a) Schematic summary of different antimicrobial mechanisms facilitated by FSP synthesized nanomaterials. (b) Scanning Transmission Electron Microscopy (STEM) micrograph of Ag/SiO2 nanoparticles synthesized using silver acetate/HDMSO precursor solution. (c) Scanning Transmission Electron Microscopy (STEM) micrograph of Ag/SiO2 nanoparticles synthesized using silver nitrate/HDMSO precursor solution. (d) SEM micrograph of PET paper sample before (inset) and after coating of Ag NPs. (e) Study of antimicrobial property of Ag NPs coated on PET paper against E. coli. (f) Digital image of crystal violet stained uncoated and TiO2 and P25 coated aluminium substrates with and without UV irradiation. (f) Spectrophotometric study (at 595 nm) of biofilm mass quantification using crystal violet staining. Reprinted with permission [Citation133–135]](/cms/asset/281f48f6-a74b-498f-a7cb-49580f117320/tapx_a_1997153_f0014_oc.jpg)
Figure 15. (a) Sensing mechanism of a chemiresistive gas sensor. (b) Sensor signal of different Pt doped SnO2 sensor towards different CO concentrations at 400°C. (c) Sensor response of 10 mol% Si-doped WO3 towards acetone (600 ppb) at different relative humidity at 400°C. (d) Dynamic changes in resistance under exposure of H2 at 150°C. (e) Selectivity plot of the developed Pt doped WO3 sensor towards interfering analytes. (f) Selectivity plots of 0.5 wt.% CuO doped SnO2 respectively. Reprinted with permission [Citation6,Citation141–143]
![Figure 15. (a) Sensing mechanism of a chemiresistive gas sensor. (b) Sensor signal of different Pt doped SnO2 sensor towards different CO concentrations at 400°C. (c) Sensor response of 10 mol% Si-doped WO3 towards acetone (600 ppb) at different relative humidity at 400°C. (d) Dynamic changes in resistance under exposure of H2 at 150°C. (e) Selectivity plot of the developed Pt doped WO3 sensor towards interfering analytes. (f) Selectivity plots of 0.5 wt.% CuO doped SnO2 respectively. Reprinted with permission [Citation6,Citation141–143]](/cms/asset/c8b4dea9-388b-4a99-97c1-dd2d88c4614c/tapx_a_1997153_f0015_oc.jpg)
Figure 16. (a) Plasmonic sensing mechanism based on the detection of transmitted light. (b) Schematic illustration of enclosed flame reactor for synthesis of SiO2-Ag NP. (c) Plasmonic biosensor adsorption kinetics of partially coated (squared, triangles) and fully coated (circles, diamonds) SiO2-Ag NP. (d) Schematic rendering of resonant Au-TiO2 fractal metamaterial. (e) Dynamic LSPR response of the resonant fractal metamaterial towards various concentrations of toluene. (f) Bar plot representing response of Au-Bi2O3 towards different VOCs exposed at different concentrations. Reprinted with permission [Citation144,Citation150,Citation152]
![Figure 16. (a) Plasmonic sensing mechanism based on the detection of transmitted light. (b) Schematic illustration of enclosed flame reactor for synthesis of SiO2-Ag NP. (c) Plasmonic biosensor adsorption kinetics of partially coated (squared, triangles) and fully coated (circles, diamonds) SiO2-Ag NP. (d) Schematic rendering of resonant Au-TiO2 fractal metamaterial. (e) Dynamic LSPR response of the resonant fractal metamaterial towards various concentrations of toluene. (f) Bar plot representing response of Au-Bi2O3 towards different VOCs exposed at different concentrations. Reprinted with permission [Citation144,Citation150,Citation152]](/cms/asset/929daa05-2e30-4aa2-b069-843b01290887/tapx_a_1997153_f0016_oc.jpg)
Figure 17. (a) Simplified schematic of the working principle of a photoresistor detector based on a porous network of semiconductor nanoparticles. (b) I–V characteristic (c) responsivity graphs of ZnO ultra porous photodetectors as a function of light and bias. (d) Schematic architectural representation of the photodetector device was composed of a photoresponsive nanoparticle layer (ZnO), a dielectric spacer layer (SiO2), and a highly transparent tunable filter layer (TiO2). (e) I–V characteristics of the device as a function of the TiO2 film thickness (illumination = 370 nm; light density = 86 μW /cm2 (f) I–V characteristics of 40 s sputtered NiO/ZnO under dark and UV lights condition at a bias between 0 and 2 mV. Reprinted with permission [Citation8,Citation154,Citation155]
![Figure 17. (a) Simplified schematic of the working principle of a photoresistor detector based on a porous network of semiconductor nanoparticles. (b) I–V characteristic (c) responsivity graphs of ZnO ultra porous photodetectors as a function of light and bias. (d) Schematic architectural representation of the photodetector device was composed of a photoresponsive nanoparticle layer (ZnO), a dielectric spacer layer (SiO2), and a highly transparent tunable filter layer (TiO2). (e) I–V characteristics of the device as a function of the TiO2 film thickness (illumination = 370 nm; light density = 86 μW /cm2 (f) I–V characteristics of 40 s sputtered NiO/ZnO under dark and UV lights condition at a bias between 0 and 2 mV. Reprinted with permission [Citation8,Citation154,Citation155]](/cms/asset/60444bb0-5b31-48bd-9ebe-8f8ec048bd5b/tapx_a_1997153_f0017_oc.jpg)
A very distinct morphology of nanomaterials is obtained if the cluster starts nucleating in droplets before the droplet evaporation (droplet-to-cluster route). Nanocrystals may nucleate on the surface or bulk of the droplets with similar mechanisms to those for the sol-gel synthesis route. The subsequent sintering of these nanocrystals in the high-temperature flame and aerosol leads to the formation of core-shell microparticles with various levels of fragmentation or too dense sub-microparticles in case of nucleation on the droplet surface and bulk, respectively. Overall, the droplet-to-cluster route provides less reproducible and controllable materials than the vapour-to-cluster route, making the latter the preferred candidate for the nanofabrication and integration in devices. Strobel and Pratsinis demonstrated the synthesis of a variety of metal-oxides using FSP via the droplet-to-cluster route [Citation19]. In their attempt to study the effect of solvent composition on particle formation during FSP, they observed that the growth mechanism followed by large particles of Al2O3, Fe2O3, and CoxOy was the droplet-to-cluster route, while small nanoparticles of the same oxides were formed via complete evaporation of the precursor (i.e. via vapour-to-cluster route). They also noticed that for the flame synthesis of ZnO and MgO the dominant growth mechanism was the vapour-to-cluster route.
Engineering of the precursor atomization process and solution composition is therefore essential to control the nanomaterial synthesis mechanism. Jossen et al. proposed the first framework for the design of the precursor solution with the critical factors being the boiling point (Tbp) of the solvent, decomposition or melting point (Td/mp) of metal precursor, and the combustion enthalpy of the solution [Citation20]. It was suggested that a high Tbp/Td/mp ratio (> 1.05) and combustion enthalpy (> 4.7 kJ/ggas) would lead to particle formation via the vapour-to-cluster route. While low Tbp/Td/mp along with low combustion enthalpy (<4.7 kJ/ggas) would lead to incomplete precursor droplet evaporation and nucleation in the liquid phase via the droplet-to-cluster route. However, it was later observed that multi-component systems could be developed depending on the material properties, kinetics, and thermodynamics of different particle configurations [Citation4].
2.2. Liquid-fed flame synthesis reactors
2.2.1. Burners
Various types of burners have been employed in laboratories and industries for the production of aerosol nanoparticles using liquid-fed flames. In a typical FSP burner (), a soluble precursor is dissolved in a flammable liquid solvent and dispersed through a two-fluid atomizer with oxygen or air used as atomization/dispersion gas. The resulting precursor droplets are ignited by a pilot flame, usually a premixed oxygen-methane flame that also helps stabilize the combustion of the liquid precursor solution. The burner can be enclosed in a cylindrical chamber with a supply of additional air to ensure that sufficient oxygen is provided for the combustion and to cool the aerosol prior to collection in filters. The advantages of FSP burners include scalability, synthesis of various types of nanomaterials, including pure metals, oxides, and easy functionalization of the aerosol downstream of the burner.
Usage of hydrogen fuel () for the synthesis of aerosol nanoparticles has also been widely studied [Citation21,Citation22] due to its advantage of the production of non-toxic by-products and low enthalpy requirements (as the main source of energy is provided by H2 flame). A cold walled chamber around the flame helps in the collection of both the synthesized aerosols and water vapour in a suspension that are produced via thermophoresis and condensation, respectively.
The counter-flow flame has a quasi-one-dimensional geometry used to synthesize single oxide (SiO2/Al2O3) and multi-component oxide (TiO2/SiO2, SiO2/GeO2) nanoparticles, with reduced complexity. The burner is composed of two vertically opposed nozzle cross-sections separated at a certain distance. The fuel (H2/CH4+ inert gas) flows from the lower tube upwards, while the oxidizer (O2+ inert gas) flows from the upper tube downwards, resulting in the formation of a thin flame sheet that can be controlled by regulating the flow rate of dilution gas [Citation23,Citation24].
2.2.2. Atomizers
A key step to achieve nanoparticle aerosol formation by vapour-to-particle route is the effective atomization of the precursor solution into sufficiently small droplets that evaporate before nucleation of the precursor in the liquid phase. For effective atomization of the liquid precursor, the inertial force applied on the precursor to aid atomization must be larger than its surface tension. Driving forces employed to assist atomization include ultrasonic, electrostatic, centrifugal, and pressure [Citation25]. The most common approach is the use of a two-fluid atomizer. A two-fluid atomizer operates by applying a shear force to the liquid, causing the expansion of pressurized gas fed through a small gap [Citation26]. Another common approach is the ultrasonic atomizers () operate under sheer force via ultrasonic vibrations that are generated with a piezoelectric disc, resulting in droplet size ranging between 1 and 10 μm which can be varied by controlling the frequency of vibration [Citation27].
2.2.3. Reactors
The reactor setup is the operating unit that provides the high thermal energy required for the evaporation of the precursor and facilitates the chemical conversation for the synthesis of nanoparticles. Over the years, different reactors have been utilized for aerosol phases synthesis of nanomaterials for different device applications.
A hot wall reactor utilizes either fuel combustion or electricity to help heat the precursor, which is subsequently flown through a metal/ceramic tube to evaporate solids to typically synthesize non-oxide nanoparticles [Citation28,Citation29]. The advantage of such a type of reactor is its well-defined temperature and residence time distributions that have helped in the oxidation of SiCl4 for the development of light guide preform and decomposition of SiH4 for silicon synthesis [Citation28]. Additionally, hot-wall reactors have also been reported to be used for decomposition of Ni(CO)4 for Ni synthesis [Citation30] and synthesis of various carbides, borides, and other nanomaterials [Citation31].
Plasma reactors produce gas containing charged nanoparticles (plasma), ions, or electrons with the help of different electrode configurations. Plasma can also be generated with the help of needles or flat electrodes placed around a plume capable of generating electric field or via spark aerosol generators [Citation32]. The advantage of plasma reactors is that by tuning their temperature, either coatings of sensitive material (at low temperature/with non-equilibrium plasma) or evaporation or decomposition of solid precursors (at high temperatures/with thermal plasma) can be achieved and also they produce narrow distribution of particles as Coulomb forces are more prevalent than Brownian coagulation [Citation2]. Plasma reactors have been known to synthesize luminescent Si nanoparticles [Citation33], super-hard carbide films [Citation34], and various heterogeneous catalysts [Citation35].
Microwave and laser reactors are other commonly used reactor setups. Microwave reactors utilize a microwave cavity [Citation36], while a laser reactor operates with the help of a laser beam [Citation37] to generate a high-energy environment to produce plasma for the synthesis of nanoparticles. Microwave reactors have been reported for the synthesis of size-controlled Si nanoparticles in bulk [Citation38], while laser reactors have been used for the synthesis of non-oxide nanoparticles and Si for solar panels [Citation32].
Inert gas condensation has revolutionized the synthesis of nanostructured ceramics and metals with the evaporation of metal precursors at low pressure (under inert helium/argon conditions), which are subsequently cooled naturally or via forced convection and finally collected on cold surfaces [Citation39,Citation40]. In such a reactor, the particles travel from a supersaturated region in the crucible to a cold rotating substrate via a convective flow within the reactors. Once the particle sticks to the cold substrate, the growth of the nanostructure is terminated and is scraped off the substrate for collection. Inert gas condensation reactors have been used to synthesize cerium oxide powders under helium environment [Citation41] along with silver-copper nanoparticles [Citation42].
Among the various aforementioned reactor setups, flame aerosol reactors have been considered as the most energetically favourable owing to the direct usage of energy and fuel from the precursors for the synthesis of films and powders for device applications [Citation43]. Flame reactors utilize high temperatures (~500–1700°C) to produce high particle concentration (>107 cm−3) [Citation44]. However, the nanoparticle produced is greatly affected by the burner type, precursor/oxygen rates, turbulent flow conditions, and relative average velocities within the flame. Flame reactors have been widely used for the synthesis of various catalysts [Citation45], magnetic nanocomposites [Citation46], nutritional materials [Citation47], and bio-materials [Citation48].
In a typical FSP reactor, the apparatus is divided into three sub-components: the atomization device, burners and flame configuration, and particle collection/deposition system. While the latter two have been previously discussed, it is essential to note the importance of nanoparticle collection system/insitu assembly of nanoparticles in an FSP reactor setup for device applications. The nanomaterials generated using FSP can either be collected in the form of powders or directly deposited onto substrates depending on different applications. Flame-made powders can be collected using several different types of systems such as baghouse filters [Citation49], parallel plate electrostatic precipitators [Citation13,Citation50], and glass fiber filters using vacuum pump [Citation51]. The advantage of FSP setups is the flexibility offered to alter the system according to specific applications. In recent years, direct deposition of flame-made materials has been prioritized to eliminate further processing steps for fabrication of devices, therefore the powder collection systems have been replaced with suitable substrates. Some of the other advantages of direct deposition of flame-made nanomaterials onto substrates include high characteristic growth rate, uniformity, and polycrystalline quality of the deposited material. Various characteristics of the substrate such as roughness, thickness, and position from the burner, must be considered for optimized device fabrication. The substrates are generally placed downstream of the flame nozzle with an orthogonal impact angle using a temperature-controlled holder.
Depending on the temperature and different types of substrates, particle morphology and microstructure of the coating can be varied and optimized for specific device applications. Chew et al. studied the effect of substrate on different morphological synthesis of LiMn2O4 films deposited via FSP and in situ annealing [Citation52]. Lithium acetylacetonate and manganese acetylacetonate in organic solutions as precursor solutions were ignited and stabilized in a premixed CH4/O2 flame through a nozzle and the resultant nanoparticles were collected on two different substrates, stainless steel (SS) and aluminium coated carbon-based primer (ACP). From morphological SEM analysis they observed that prior to in situ annealing, the films on both SS and ACP substrates had a homogeneous, crack-free film (thickness ~7 µm, 98% porosity) with a lace-like structure of uniformly sized nanograins. After in situ annealing, it was noticed that the lace-like nanograins on SS substrates transform into cauliflower-like structures, however, there was no change observed on ACP substrates. It was also observed that the SS substrates showed better adhesion towards the deposited film than ACP substrates.
2.3. Nanomaterial integration and device fabrication
The flame-assisted nucleation of nanoparticles in the gas phase provides a flexible tool for the fabrication of porous nanostructured films via the spontaneous self-assembly of aerosols on a substrate (). Building on the original framework introduced by Péclet, Rodriguez-Perez et al. proposed scaling laws to describe the morphology of aerosol self-assembled nanoparticle layers as a function of the mechanism of particle arrivals [Citation53,Citation54]. Overall, stochastically reproducible morphologies have been observed and correlated to the scaled ratio between the advective velocity and diffusivity, namely the Péclet number (Pe). The average porosity of such films has been observed to follow an S-shaped curved varying from ca 86% in the ballistic regime (Pe ≫ 1) to ca 98% in the diffusion regime (Pe ≪ 1). Between these regimes a transition regime is found, where both ballistic and diffusion-driven mechanisms provide comparable contributions to the resulting nanoparticle film morphology and an average film porosity between 86% and 98% is observed.
Recent studies have broadened these original findings, considering the impact of deposition of nanoparticle agglomerates [Citation55] instead of single particles and the effect induced by the particle size [Citation16]. In aerosols, agglomerates are formed either by the collision of spheroidal primary particles or among smaller agglomerates at temperatures sufficiently low to avoid complete coalescence [Citation56]. These agglomerates can either be bonded via weak van der Waals forces or stronger covalent bonds if sufficient high sintering temperature and time are experienced in the aerosol. The formation of agglomerates is initiated when the time required for coalescence (τs) exceeds the time for particle collision (τc). An increased rate (dτs/dt ≫ dτc/dt after τc = τs) facilitates the formation of soft spherical agglomerates whereas a slightly increased rate (dτs/dt > dτc/dt) forms hard oblong agglomerates [Citation57]. The advantages of direct deposition of agglomerates over spherical particles include the ease of fabrication and increased porosity.
Mädler et al. studied the dependence of average film porosity, aerosol deposition time and agglomerates vs single-particle deposition as a function of the film thickness [Citation55]. From their theoretical analysis, they concluded that for agglomerate deposition, the film porosity is dependent on the number (Np) and size of primary particles along with the fractal dimension (Df) of the agglomerate. They observed that with increasing agglomerate size, both diffusion and ballistic regimes would lead to similar film porosities, mostly controlled by the porosity of the depositing agglomerates. Nasiri et al. studied the effect of decreasing particle size, observing that in the diffusion regime particles smaller the mean free path of the aerosol would lead to a denser morphology than that expected for the diffusion regime. This densification of the nanoparticle layers is attributed to the discrete motion of the particles leading to a quasi-ballistic deposition, labelled there as randomly oriented ballistic deposition, a fourth self-assembly regime of nanoparticles [Citation16].
While high porosity may be advantageous for some applications such as gas sensing [Citation6,Citation58], it does limit the use of flame-made materials to interact in gaseous environments as capillary forces in liquids result in the rapid disintegration of these nanoparticle networks. Tricoli et al. demonstrated that for application as an electrode in dye-sensitized solar cell (DSSC), ZnO nanoparticle films with ~98% porosity had a too fragile morphology that disintegrated when immersed in liquid solutions [Citation59]. Their study revealed that in situ sintering of the nanoparticles during aerosol deposition would decrease the film porosity and result in a more robust morphology. A maximum threshold porosity of 89% porosity would lead to sufficiently robust films with up 200 μm thickness for use in DSSC. Tran-Phu et al. studied the effect of temperature on film morphology and its performance as photo-anodes for water oxidation [Citation60]. They observed that under high temperature (thermophoretic deposition of BiVO4, the particles undergo in situ sintering and the resultant film is less porous and more robust compared to the 98% porous films obtained at low-temperature depositions. They attributed the corresponding morphology to the increase in substrate surface temperature from 600 to 800°C obtained by varying the height above the burner and identified an optimal film porosity of 46% for use as robust photo-anodes for water oxidation.
The morphology of the resultant films is also dependent on the sintering behaviour of the aerosol particles, as demonstrated by Thimsen et al. [Citation61]. They fabricated nanostructured TiO2 films on ITO substrates using a single-step flame aerosol synthesis technique for dye-sensitized solar cell and photo water splitting application. The SEM micrographs showed that two different morphologies could be obtained by varying the distance between the substrate and burner. The first, granular morphology () was obtained when TiO2 nanoparticles were deposited directly from the aerosol-phase (substrate was at 4.1 cm from the flame) to form a fractal-like network on the substrate. As these aerosol particles underwent little or almost no restructuring after deposition, they formed granular like structures. The second columnar () morphology was observed when the nanoparticles were deposited out of the flame (substrate was at 1.7 cm from the flame) directly onto the ITO substrates. Such a columnar morphology was obtained due to the sintering of flame-made particles. Controlling the sintering behaviour of the aerosol particles on the substrate can result in varied morphologies. If the sintering dynamics is slow, granular like films are formed, however, if the rate of sintering is rapid, columnar morphology can be observed.
2.4. Post-processing techniques of aerosol nanomaterials
To further enhance the properties or preserve specific characteristics of FSP produced nanomaterials for specific device applications, additional in situ or ex situ techniques are employed. Some of the in situ techniques would include mixing, sintering, surface functionalization, while some of the ex situ techniques include annealing, calcination, spin coating, and so many more. Some of these post-processing techniques are briefly discussed in the following sections.
2.4.1. In situ techniques
In situ mixing of flame-made lithium titanate (Li4Ti5O12) and carbon black, as demonstrated by Gockeln et al. has shown to enhance the electrical conductivity of Li-ion battery systems [Citation62]. With xylene as the source of carbon black, N2 as the dispersion gas (instead of O2) and with no additional polymer blinders, use of the D-FSP for the introduction of carbon black into the system has proven to be a cost-effective Li-ion battery electrode manufacturing technique. Similarly, Blattmann and Pratsinis also synthesized Fe2O3, SiO2 or TiO2 – poly(vinyl alcohol) (PVA) or poly(3,4- ethylenedioxythiophene):poly(styrenesulfonate) (PEDOT:PSS) polymer nanocomposite films using a single-step FSP and in situ mixing combination [Citation63]. The advantage of the developed fabrication technique includes requirement of no further post-fabrication treatment or vacuum conditions. Additionally, the technique develops films with no agglomerates and voids with film thickness ranging from tens of nanometers to multiple micrometers, which can be extensively used for various industrial applications such as micro-electronics, optics, and biomedical.
In situ annealing has been developed by Tricoli et al. for thermally stabilizing low-temperature-deposited nanoparticles [Citation7,Citation11,Citation58,Citation64]. Upon short exposure of aerosol-free xylene flame to the thermophoretically deposited nanoparticles, they were able to transform the porous fractal network to a cauliflower-like structure. Due to the formation of sintered necks, in situ annealing facilitated a reduction in specific surface area and porosity and improved the adhesion and mechanical stability of the percolating particles, subsequently enhancing the gas sensing performance.
2.4.2. Ex situ techniques
In addition to in situ technique, ex situ annealing in furnaces/electric ovens have also been employed for specific applications. For instance, in gas sensing applications, sensor films are ex situ annealed (~300–500°C) to further improve the crystallinity and thermally stabilize the sensors [Citation65,Citation66]. For battery applications as well, researchers have shown that ex situ annealing electrodes transform the nanomaterial from amorphous to crystalline structures. Krumeich et al. reported that after ex situ annealing of FSP synthesized carbon-coated LiFePO4 nanoparticles at elevated temperature (~800°C) it leads to the crystallization of the material and remarkably improves the electrochemical performance [Citation67]. Some other reports have also shown improved properties after ex situ annealing [Citation68–70].
While most of the reactor setups still produce aerosol powders, transfer of as collected nanoparticles under slightly reduced FSP conditions to suitable substrates is extremely crucial. Different ex situ transfer techniques such as roll-to-roll lamination and spin-coating have been explored for the same. Schopf et al. demonstrated the use of two roll laminators composed of chrome plated steel pressure roll on the top and a drive roll covered with 80-durometer-silicone rubber at the bottom [Citation71]. They used this system to transfer FSP made TiO2 nanoparticles onto polypropylene foil (100 µm thickness) or aluminium foil (30 µm thickness). With defined roll speed (~7.5 cm/s) and low pressure (~2.5 MPa) they observed that the agglomerate particles form new contacts within the particles of the porous material and also between the particles and the substrate. Such a technique can be further explored for super-hydrophilic coatings or dye-sensitized solar cell applications where the nanoparticle layer must endure a liquid environment or needs to be resistant to abrasion. Liewhiran et al. explored spin-coating technique to transfer flame made SnO2 nanoparticles for gas sensing application [Citation72]. They prepared a homogeneous paste of ethyl cellulose, terpineol (temporary binder), and SnO2 nanoparticles. The paste was then spin-coated at 700 RPM for 10 s and at 3000 RPM for 30 s on Al2O3 substrates with interdigitated Au electrodes. To remove the binder, the sensor films were annealed at 150°C for 1 h. The drawbacks associated with such ex situ techniques for transfer of flame made nanoparticles include requirement of additional fabrication steps, bulky infrastructure, and toxic chemicals. To curb these disadvantages, one-step direct deposition of flame-made particles have greatly been researched.
2.5. Aerosol film characterization
Characterization of gas-phased synthesized nanoparticles is essential to understand their properties for various applications. Some of the techniques utilized for aerosol films are summarized in .
Table 1. Techniques employed for characterization of flame-based aerosol films
Nanoparticles synthesized using FSP can be characterized using a variety of ex situ powder structures, physical and chemical characterization techniques such as Powdered X-Ray Diffraction (XRD), Brunauer-Emmett-Teller (BET), Transmission Electron Microscopy (TEM), and so many more [Citation73].
3. Theoretical modelling and experimental validation
FSP offers both single-component and multi-component synthesis of metal oxide nanomaterials. Numerous physio-chemical processes (such as diffusion, condensation, nucleation, and aerosol coagulation) are involved in the synthesis of nanoparticles in the turbulent flame, making numerical simulations for the improvement of such systems challenging.
The population balance model (PMB) studies the effect of size distribution during nanoparticles generation, utilizing different synthesis parameters such as fluid velocity, temperature, and species information with reaction kinetics. While molecular dynamics (MD) simulations aid in understanding the role of further physical details such as collision and coalescence. Additionally, reactive MD simulations help understanding synthesis mechanism involved in heterogeneous reactions [Citation74]. All these theoretical models can significantly help improve the overall FSP procedure and optimize FSP reactors.
3.1. Time-scale analysis
Initial time-scale analysis of gas-phase synthesized nanoparticles aid in the determination of important physical factors (calculation of characteristic reaction times) involved in estimating the final size and morphology. Such simulations can further assist decoupling various multi-scale processes such as Brownian coagulation and sintering [Citation75,Citation76]. Time-scale analysis reveals that when the Brownian coagulation time is larger than the sintering time, primary particles have sufficient time to merge into new particles before the collision. However, if sintering time is larger than coagulation time, the formation of aggregates and agglomerates will be dominated [Citation75].
Early numerical studies of SiO2-TiO2 aerosol systems by Zachariah and Dimitriou showed that with characteristic time-scale analysis formation of agglomerates can be avoided through controlled nucleation rates [Citation77]. Experimental studies conducted by Wang et al. on the synthesis of TiO2 anatase phase described the effect of substrate-to-burner distance, precursor loading rate, and sintering and growth mechanism on the substrate. Their theoretical studies strongly supported experimental results that sintering and growth of TiO2 nanoparticles (4–5 nm) are not supported on cold (~104°C) substrates [Citation78].
3.2. Population balance model (PBM)
In a typical flame synthesis of nanomaterials, the PBM studies define the time derivative of particle concentration, taking into consideration nucleation, coagulation, and surface growth. All these parameters are governed by the aerosol general dynamic equation:
where ‘k’ is the number of atoms/molecules in the particle system.
As FSP for device application is generally a one-step direct synthesis technique, the growth mechanism of nanomaterials in such systems is primarily determined by the local fluid field along with residence time of particles and temperature profile and thus is influenced by both physical and chemical processes involved in the synthesis. In laminar flames, the combination of PBM and fluid dynamics can be utilized for particle generation. However, in turbulent flow, computational fluid dynamics (CFD) with additional assumptions and approximations might be required, as demonstrated by Johannessen et al. [Citation79] and Mühlenweg et al [Citation80].
Chemical reactions of the precursors also play a vital role in particle growth during FSP. At high temperatures, the breakdown of the volatile precursor molecules initiates vapour formation, followed by nucleation and finally the growth of nanoparticles. A variety of kinetic reactions such as pyrolysis, hydrolysis, and surface reactions contribute to the synthesis of final metal oxide particles. Computational modelling has been employed to understand such principal reactions. For instance, Shmakov et al. studied both experimentally and computationally the combustion of titanium tetra-isopropoxide (TTIP) precursor for TiO2 nanoparticle synthesis via FSP [Citation81]. Their study concluded that pyrolysis and hydrolysis of TTIP with high and low activation energy, respectively, are the two predominant reactions in OH-rich flames. Some of the other showing similar studies have been well reported [Citation82–84].
3.3. Molecular dynamic (MD) simulations
MD describes the Newtonian dynamic equations to understand the motion of atoms based on their interatomic potentials. These have been utilized to quantitatively understand collision-coalescence reactions, heterogeneous catalytic reactions, and various other physical and chemical processes involved in aerosol nanoparticle generation for device applications.
Understanding the effect of interacting and intrinsic forces between nanoparticles is important in fabricating structures for specific applications to enhance nanoparticle property and overcome the drawbacks of agglomeration. Initial work by Yan et al. was an attempt to understand the contribution of dispersive van der Waals (vdW) and Coulombic dipolar forces between pairs of co-oriented and counter-oriented charge neutral TiO2 anatase nanoparticles in vacuum for different particle sizes and initial particle temperatures [Citation85]. With the help of MD simulations, Matsui-Akaogi interatomic potential and Hamaker approximations their study concluded that attractive Coulombic dipolar forces have greater influence than vdW forces. Their study also showed that at high temperatures, thermal fluctuations due to Ti and O surface ions could lead to increased fluctuations in the angular directions of nanoparticle dipole vector, subsequently decreasing the magnitude of the time-averaged dipole, causing weakened Coulombic dipolar interactions between two TiO2 nanoparticles.
Determining the porosity and thickness of the nanoparticle deposited using one-step FSP is also an important parameter for fabricating suitable devices for different applications. Theoretical studies carried out by Mädler et al. showed that morphological characteristics of nanoparticle agglomerates, such as fractal dimensions (Df) along with a number (Np) and size (dp) of the primary particles can greatly affect the film porosity [Citation55]. Their studies showed that the direct dependence of Np and indirect dependence of Df on film porosity deposited (). They also reported that the porosity and thickness of the deposited fractal film can be controlled independently by varying the deposition time and temperature difference between the flame and depositing surface (i.e. controlling the height between the flame nozzle and substrate holder). Studies by Nasiri et al. have shown ultra-fine nanoparticle films deposited in the ballistic regime follow a parallel agglomerate growth in the vertical direction thereby resulting in 300% denser morphologies [Citation16]. Their study also showed that in the diffusion regime, the resulting films had denser morphologies near the surface and decrease the particle size from 100 to 1 nm .
3.4. Langevin dynamics (LD) simulations
LD has been used to simulate trajectories of aerosols to understand particle transport in internal and external flows. Elmøe et al. studied the effect on three-dimensional morphology and time-evolution during nanoparticle filtration through capillaries using LD simulations as a function of Pe number (0.011–10) [Citation15]. Their theoretical studies identified three distinctive regimes: capillary deposition, clogging, and cake growth. As the Pe number is increased and capillary clogging time is decreased, the formation of a void cone-like structure is observed (inside the capillary) where the solid volume increases upon increasing the distance from the capillary inlet (). Additionally, the solid volume fraction profile () showed that a constant solid volume fraction is observed at high Pe number (Pe = 10) and small capillary radii (Rc = 1 μm), however, at larger Rc, the cake has not developed completely. Therefore their studies concluded that at low Pe number, the structures obtained are of high porosity (98%) () [Citation3], and at low Pe number, the porosity is reduced, leading to the formation of a void cone upstream.
4. Integration of flame-made nanoparticles in devices
4.1. Catalysis
In addition to their use as pigments for the paint industry and filler to reinforce rubber, flame-made materials have found one of their first use in catalysis, accounting for the production of one of the most widely used commercial photo-catalysts, Degussa (Evonik) P25 [Citation2]. Conventionally catalysts are produced using sol-gel, hydrothermal, co-precipitation, or various other wet-phase techniques, which require multiple post-processing steps such as calcination, drying, and filtration [Citation86]. Flame synthesis has the advantage to produce nanocatalysts and films in one step. Furthermore, the steep temperature gradient in the flame enables the production of metastable material compositions with structural properties that are not possible through other steady-state approaches [Citation87,Citation88]. The scalability, single step, and relatively low cost of the flame synthesis route in combination with the feasibility of producing a large range of single, binary, and ternary metal oxide nanoparticles are particularly suited to the engineering of the activity and selectivity of catalysts and their industrial production. Despite this advantage, the direct integration of flame-made catalysts in devices by aerosol deposition has often encountered issues related to the insufficient mechanical stability and limited electrical and thermal conductivity of the self-assembled layers [Citation7]. In the next subsections, we will report some recent achievements in the application of flame synthesis to the engineering of catalysts for application in photo-catalysis, (photo)electrochemistry, and thermochemistry.
4.1.1. Photo-catalysis
The direct use of solar energy to drive chemical reactions via photo-catalysis provides ample application potential ranging from water purification to the production of important chemical commodities and renewable energy carriers such as H2. summarizes the representative use of photo-catalysis for water purification [Citation89]. The mechanism can be summarized into four steps: (1) absorption of photons having energy equal to or greater than the material’s band gap to generate electron-hole pairs; (2) separation of the charges; (3) migration of electrons and holes to the surface with production of active radicals; and (4) degradation of the pollutants in more benign products by active radicals [Citation90]. A similar mechanism can be utilized to drive various processes such as the oxygen evolution reaction (OER) and hydrogen evolution reaction (HER), mimicking the natural photosynthesis process for water splitting. While most use of flame-made materials for photo-catalysis has been demonstrated with powder liquid suspensions, the application of photo-catalysts on substrates has been investigated as a mean to impart specific functionality such as self-cleaning [Citation91] to various surfaces including the external walls of buildings and windows, and to produce integrated devices such as for water purification [Citation92] and water splitting [Citation61].
Chiarello et al. reported the use of TiO2-based powder samples (), synthesized by FSP, for hydrogen production in a methanol reformer [Citation93]. Methanol was used as an efficient hole scavenger to reduce the recombination rate of electrons and holes, and thus increasing the conduction band electrons available for HER. Kho et al. demonstrated the use of BiVO4 thin films for photo-catalytic oxygen evolution [Citation94]. (). Their photo-catalytic thin films were prepared by doctor blading FSP synthesized BiVO4 and acetylacetone paste onto ITOs. They observed that aqueous modification of FSP-made BiVO4 using nitric acid solution improved the photo-catalytic activity by 20% due to the crystallization-reconstruction of the BiVO4 unit cell. Xiong et al. prepared ZnO/CeO2 nanocomposite powders using FSP for CO2 reduction to CO and CH4 gases [Citation95] (, e). They attributed the high photo-catalytic performance to the formation of a ZnO/CeO2 heterojunction structure and co-existence of Ce3+/Ce4+ ions that promoted the separation of photogenerated electron-hole pairs under UV-Visible light illumination. Abe et al. evaluated the performance of ferroelectric La2Ti2O7 perovskite-slab type photo-catalyst powders, made by FSP, for photo-degradation of methyl orange under UV irradiation [Citation96] (). They credited the increased photo-catalytic activity to the improved crystallinity and reduced defect density.
It should be noted that while most of these studies demonstrated a good photo-catalytic performance using nanoparticle suspensions, their application as immobilized photo-catalysts once integrated into a device requires further engineering of the meso- and macro-scale layer morphology and porosity to overcome possible bottlenecks related to the diffusion of reactants and products within the layer. Immobilization of flame-made ZnO nanoparticles for use in dye-sensitized solar cells [Citation59] has shown a strong dependency of the cell efficiency from the ZnO layer morphology, indicating that higher porosity and a distribution of meso- and micro-scale pores can significantly increase the electrolyte mass transfer and cell efficiency. However, increasing the ZnO layer porosity above a maximal threshold of ca 89% resulted in the disintegration of the layer due to capillary forces. As such, engineering the morphology and porosity of flame-made layers to achieve an optimal compromise between mass transfer rates and mechanical stability remains an important research topic for the future design of nanostructured layers for applications in liquids.
4.1.2. (Photo)Electrochemical catalysis
In addition to their initial use as photo-catalysts, the application of flame synthesis for the one-step fabrication of (photo)electrochemical devices has been increasingly investigated in the past few years, demonstrating the rapid and facile preparation of a variety of photo-anodes [Citation97] and -cathodes [Citation98]. In particular, the electrochemical production of H2 has attracted great research and industrial interest as a carbon emission-free route for chemical storage and off-the-grid use of renewable energy. Among various approaches, electrochemical (EC) and photo-electrochemical (PEC) H2 production are highly promising [Citation99].
shows a simplified schematic description of water splitting mechanisms employed in EC and PEC systems. The EC setup uses an external power source, such as renewably produced electricity in photovoltaic and wind turbine plants, to drive the reduction-oxidation reactions between the electrodes. In contrast, PEC-based technologies rely on the direct use of sunlight to provide the energy required for water oxidation and water reduction reactions [Citation99]. Early studies by Ng et al. showed the comparison of properties of flame-made pristine BiVO4 and BiVO4-rGO composite () [Citation100], for PEC water splitting. From the transient photocurrent responses of both the materials, they observed a slow recombination process in BiVO4-rGO nanocomposite owing to the improved electron transport. The presence of numerous grain boundaries and rapid electron transfer between BiVO4 and rGO (due to its flat band potential) led to an enhancement in PEC activity.
Chiang et al. investigated FSP synthesized CuO nanoparticles (FSP-CuO) spin-coated onto ITO substrates for PEC H2 production () [Citation98]. They compared the performance of the FSP-CuO with commercially purchased CuO nanoparticles and concluded that the photocurrent generated by FSP-CuO was 4.2–7.8 times higher due to the uniform morphology of the synthesized particles.
Liu et al. studied the EC activity of Co3O4 nano-islands made by direct FSP synthesis and deposition as OER catalysts for H2 production on FTO glass substrates [Citation9]. Different deposition times were analysed, and it was concluded that increasing the deposition time initially improved the performance (3–15 s) and thereafter did not provide further enhancements (15 s to 4 min) due to the poor electron conductivity and inaccessibility of catalytic sites within the thick Co3O4 layers (thickness greater than 30 ± 6 nm). They also noted redox events before the onset of water-splitting reactions, which were attributed to the oxidation of the Co3O4 surface to CoOOH (). An excellent long-term term electrochemical stability was reported with no obvious deterioration in the performance over 12 h of operation at constant current (). The direct aerosol deposition route enabled facile deposition of these flame-made Co3O4 co-catalysts on GaN nanowire photo-anodes for PEC OER.
Tran-Phu et al. investigated the photooxidation activity of direct thermophoretically deposited and in situ sintered flame-made BiVO4 photoanodes on FTO-coated glass substrates with various porosities ranging between 12% and 18% (Figure 56) [Citation60]. They observed that 46% porosity provided the best photocurrent density for sulphite and water oxidation due to enhanced electrochemically active surface area and optimal charge transport and surface reactions compared to 12% and 80% porous films.
4.1.3. Thermochemical catalysis
The use of flame-made nanomaterials as catalysts in thermochemical reactions has been explored as one of their first application due to stability of metal oxides, synthesized in flames in harsh chemical and temperature environments. In particular, the use of flame-made thermocatalysts for CO2 reduction and water splitting has been the focus of various recent studies. Increased anthropogenic activities, such as the burning of fossil fuel and emission of greenhouse gases have increased the amount of atmospheric CO2 and tropospheric O3 emission resulting in significant environmental impact. In order to curb CO2 emissions, three main approaches are considered: (1) shifting energy carrier from fossil fuel to greener sources such as hydrogen; (2) CO2 capture, and (3) CO2 utilization [Citation101]. Amongst the three strategies, CO2 utilization in catalytic conversions is considered as a rapidly implementable solution with 20–40 times more efficiency for CO2 reduction than CO2 sequestration [Citation102].
Thermochemical catalytic conversion of CO2 to various value-added products such as carbon monoxide (CO), methanol (CH3OH), dimethyl ether (DME), and methane (CH4) have been widely explored. represents the general working principle of a thermochemical catalytic reactor for CO2 reduction. Gao et al. explored the use of FSP-made manganese (Mn) powders for thermochemical synthesis of fuels (H2 and CO) from CO2 [Citation103]. They observed that the incorporation of cerium (Ce) ions into the Mn crystal lattice significantly increased the oxygen exchange capacity and resulting mass specific H2 and CO yield (). To assess the thermochemical stability of the synthesized material, they performed 100 continuous redox cycles and observed that after some initial variations the step-yields stabilized after 50 cycles, which was credited to the decrease in surface area of nanoparticles during sintering ().
Tada et al. studied the conversion of CO2 to CH3OH using D-FSP synthesized CuO-ZrO2 powdered nanocatalysts [Citation104]. They observed that these catalysts had higher catalytic activity and normalized by mass of surface, exposed copper compared to commercial catalyst owing to the incorporation of ZrO2 into the crystal lattice of CuO (). Wegner et al. synthesized powdered Hopcalite catalysts using FSP and compared its performance with commercial Hopcalite towards CO conversion under dry and humid conditions [Citation105]. They observed that the catalytic activity is dependent on the surface area and carbon contents among the different samples (). Lovell et al. studied the performance of D-FSP synthesized Si/CeZr as supports for Nickel (Ni) for dry catalytic reforming of CO2 to CH4 [Citation106]. They observed that the enhanced oxygen-storage capacity provided by D-FSP Si/CeZr was due to the increased CO2 conversion and surface O* (indicates the oxygen species adsorbed on the active sites of the D-FSP synthesized catalyst) that ultimately promoted reverse water gas shift ().
Catalysts often need to possess a high surface area with crystalline porous structural morphology for enhanced catalytic performance. As FSP is generally performed at high temperatures, it results in the production of catalysts that have high thermal stability and prevents extensive sintering, which thereby facilitates in enhanced catalytic reactions. Additionally, FSP can be utilized to produce catalysts based on composite metal oxide, supported noble metals, and porous membrane layers, with increased scalability, which have greatly attracted the production of flame-based catalysts for industrial applications.
4.2. Energy conversion devices
The use of flame-made materials in energy conversion devices has been mostly focused on their integration as active materials in electrodes of fuel cells, batteries, supercapacitors, and dye-sensitized solar cells. The high purity and crystallinity of metal oxide semiconductors obtained through the flame route result in good chemical stability and electronic properties that have shown some benefits for integration in various energy conversion devices. In the next sections, we will present a succinct outlook of some successful flame-made material compositions and device architecture.
4.2.1. Fuel cells
The fuel cell is a key technology to enable direct combustion-less production of electrical energy from hydrogen. Fuel cells feature several advantages for the use of hydrogen as clean energy carrier including the environmental friendliness of the final products being heat and water, a high energy conversion efficiency, and a compact and simple design [Citation107]. A fuel cell is composed of two electrodes (cathode and anode) on either side of an electrolyte layer (). Oxygen from air is continuously fed to the cathode, while hydrogen is fed to the anode, where it is oxidized into ions. The electrolyte layer acts as an insulator only allowing the H+ positive ions to pass from the anode to the cathode. This results in electrical potential between the anode and cathode that can be directly utilized through an external circuit. The overall reactions at the cathode and anode are:
Among the various types of fuel cells, solid-state fuel cells have been widely studied due to their enhanced efficiency. However, a major drawback is their high operational temperature [Citation5]. The development of solid-state electrolytes with higher low-temperature ion conductivity and better design of the electrodes can help lower the operating temperature of solid-state fuel cells. Early studies by Choy et al. demonstrate the performance of directly deposited lanthanum strontium manganese oxide (LSM) as a cathode for fuel cells using a combination of spray pyrolysis and flame synthesis [Citation108]. They studied the interfacial electrical resistance at different temperatures and observed that their cathode material required a relatively low activation energy of 96.29 kJ/mol. They attributed this behaviour to the porous morphology of the structure, which helps in enhancing the oxygen reduction reactions, thereby lowering the interfacial resistance loss compared to electrodes produced by other techniques such as slurry paint.
Liu et al. fabricated fuel cell electrodes composed of 70 wt.% Sm0.5Sr0.5CoO3-δ and 30 wt.% Sm0.1Ce0.9O3-δ as cathode and 70% wt.% Ni and 30 wt.% Sm0.1Ce0.9O3-δ as an anode, both prepared on Ni-Ce0.9Gd0.1O1.95 electrolyte pellet via combustion chemical vapour deposition () [Citation5]. They observed that their fuel cell electrodes performed better with lower polarization resistance compared to electrodes prepared using other techniques such as slurry painting, screen printing, and spin-coating owing to the highly porous nanostructured electrodes with high surface area.
Seo et al. synthesized Ce1-xGdxO2-x/2 nanoparticles () using FSP, which were subsequently pelletized and sintered for 3 h at 1400°C for a solid electrolyte for fuel cells [Citation109]. A linear relation between temperature and ionic conductivity of the electrolyte was observed, confirming that at a lower temperature the formation of defect associations decreases the ionic conductivity; while at a higher temperature, increased oxide ion mobility increases the conductivity (). While studying the dependence of the concentration of Gd content and ionic conductivity prepared by different techniques, they observed that FSP produced nanoparticles had the highest conductivity due to larger lattice constants.
In addition to lowering the operating temperature, the incorporation of catalyst into the electrodes also helps in preventing CO poisoning of the fuel cell. Lee et al. compared the performance of flame synthesized carbon-supported Pt-Ru catalyst with commercial E-TEK catalyst as a cathode for the fuel cell to overcome CO poisoning [Citation110]. They observed that flame-made materials had enhanced methanol oxidation reaction and CO stripping performance and competitive electrochemical activity, thereby enhancing the performance over that of commercial catalysts having the same composition (, f).
4.2.2. Li-ion batteries
Electrical energy storage in batteries has become increasingly important due to the need to power an increasing number of portable electrical devices and the demand for electrically powered vehicles and storage of renewably generated electricity. Amongst various technologies, rechargeable Li-ion batteries have gained prevalence due to their comparably high energy density and life cycle. A typical Li-ion battery is composed of an anode, cathode, separator soaked in an electrolyte (). Li-ions shuttle via the electrolyte from the anode to the cathode during their charging and discharging cycles. Conventionally, Li-ion batteries have been prepared using solid-state processing techniques or sol-gel routes. The latter approaches have some drawbacks associated with long processing time, a large number of steps, and the presence of impurities. As the performance of Li-ion batteries is strongly dependent on composition, crystallinity, and specific surface area of the electrode, various studies have focused on improving material fabrication and electrode assembly.
Gockeln et al. fabricated Li4Ti4O12 (LTO) battery using FSP via a direct deposition technique on flexible polyimide foil, as illustrated in [Citation111]. At higher current densities, they observed incremental deviation of the potential plateau along with a reduction in the plateau length. Both the phenomena were attributed to the typical kinetic effect caused by polarization of the cell at high current densities (). Gockeln et al. also studied the performance of D-FSP synthesized in situ coated nano Li4Ti4O12 (LTO/C) batteries [Citation62]. They utilized the combination of D-FSP and pressure-based lamination technique for the fabrication of LTO/C batteries and found that the potential had a characteristic flat voltage plateau up to a discharge rate of 1°C, indicating that the synthesized microstructure allows ease of charge/discharge reaction kinetics due to increased electronic conductivity.
Abram et al. compared the synthesis parameters and performance of LiNi0.8Co0.1Mn0.1O2 (NCM811) and LiNi0.33Co0.33Mn0.33O2 (NCM111) batteries [Citation112]. They prepared their cathodes by blending the FSP synthesized powders (90%) with conductive carbon (3%), PVDF binder (7%), and N-methyl-2-pyrrolidone solvent onto aluminium foil. They concluded that the particle morphology is strongly dependent on solution composition, precipitation, and oxidation of the precursor along with the flame temperature. NCM811 resulted in irregular shapes with broad size distribution due to higher Ni content in the precursor solution, which lowers the solubility of Ni nitrate and causes rapid precipitation and collapsing of the shell-like structure during aerosol synthesis; while NCM111 results in spherical particles from single droplets as the lower concentration of Ni in the precursor does not limit oxidation of precursor solution during synthesis. Their charge/discharge curves of both the materials presented a characteristic voltage plateau with reasonable first-cycle efficiency and initial discharge capacity. They attributed the better performing cycling behaviour of NCM811 () to lower synthesis (1350 K) and higher preheating (450 K) temperatures which help in improving the uniformity in the size distribution of primary particles and structural stability.
Bo et al. studied the use of Zeolitic Imidazolate Framework-8 (ZIF-8) self-supporting membranes () prepared through vapour-phase conversion of flame-made ZnO fractal networks for Li-S batteries [Citation113]. They observed that their tunable and hierarchical ZIF-8 membrane facilitated the use as battery separators and acted as efficient barrier for selective Li+ transport (). They attributed such improved battery cyclability to the selective diffusion of Li+ (0.076 nm) through the pores of ZIF-8 (0.34 nm) compared to long-chain Li-polysulfides (>0.68 nm).
Fuel cells and Li-ion batteries are traditionally fabricated using solvo-/hydrothermal, sol-gel, solution-combustion, or solid-state techniques [Citation114,Citation115]. The drawback associated with these techniques includes multiple fabrication steps, expensive infrastructure, and extensive post-treatment processes. With recent advancements in flame-made materials for energy storage devices, nanoparticles with higher specific surface area, shorted diffusion path for Li-ion, high charge/discharge rates [Citation116] can be easily synthesized, therefore proving as a suitable alternative to conventional techniques.
4.3. Membranes and coatings
The inherent mesoscale porosity of aerosol self-assembled layers made by deposition of nanoparticle aerosols has led to the exploration of their application as super-hydrophilic/hydrophobic layers [Citation117,Citation118] biomedical coatings and membranes [Citation119]. While preparing dense membranes with aerosol-deposited nanoparticles has shown limited success due to the high sintering temperature of various oxides and the very low initial density of these layers, recent conversion of such nanoparticle networks in metal-organic frameworks has revealed significant potential [Citation113].
4.3.1. Super-hydrophilic coatings
Super-hydrophilic coatings have been investigated in light of their wide application potential including lenses, windshields, mirrors, heat exchangers and electrolysers [Citation120]. The tendency of a substrate to repel or attract water is reflected in its water droplet contact angle (CA). Historically, if the water droplet CA is less than 90°, the water has been considered to show an affinity towards spreading over a surface, and such a phenomenon has been referred to as hydrophilicity. In case that the water CA on a hydrophilic surface is less than 10° in ca 0.5–1 s, the surface has been considered as super-hydrophilic (). Such coatings have been explored to impart anti-fogging functionality, increased heat exchange rate and increased liquid-solid interaction on a variety of materials including glasses [Citation120].
In 2009, Tricoli et al. reported the fabrication of super-hydrophobic coatings by direct deposition of FSP-made pure SiO2, TiO2 and mixed silica-titania nanoparticle layers onto glass substrates [Citation11]. They observed that a small thickness (< 100 nm) of these nanoparticle layers would result in excellent wettability and transparency, also imparting an antifogging functionality to glass slides (, c). While the TiO2 super-hydrophilicity would eventually vanish upon long-time storage in dark conditions, the SiO2 and SiO2-TiO2 nanocomposites provided durable super-hydrophilicity, emphasizing the role played by layer morphology and material surface chemistry. In 2010, Tricoli et al. extended these results demonstrating the spontaneous growth of SiO2 nanowires films by FSP, which also provided tunable super-hydrophilic-hydrophobic properties [Citation17].
In 2013, Jia et al. reported the fabrication of a directly deposited multifunctional silica nanofilm on plain glass using seedless FSP technique for anti-fogging applications [Citation121]. From their time-resolved contact angle measurements, they observed that due to the presence of hydroxyl groups in high concentrations on the surface of SiO2 along with their porous morphology, their film exhibited super-hydrophilicity (). They also tested the samples for anti-fogging applications and noticed that the property remains essentially constant for all samples.
In 2014, Tuominen et al. demonstrated the creation of super-hydrophilic surfaces of paper and board using SiO2 nanoparticles via different techniques such as FSP, flame treatment, atmospheric plasma and corona [Citation122]. They observed that super-hydrophilic surfaces prepared by FSP were stable and maintained its super-hydrophilicity () for at least 6 months while the other techniques were stable only for one day. They attributed the enhanced stability and super-hydrophilic behaviour of FSP prepared nanoparticles to the alteration of surface chemistry by SiO2 on the paper and board surfaces.
In 2018, De Falco et al. demonstrated the hydrophilic properties using TiO2 coatings that were prepared using FSP and thermophoretic deposition [Citation123]. They observed that by reducing the particle size of the nanoparticles, increased the exposed surface to volume ratio and surface-active sites. This results in an increase of hydroxylated groups on the surface, thereby increasing the hydrophilicity of the prepared coatings ().
4.3.2. Super-hydrophobic coatings
Hydrophobic coatings are usually considered as those displaying a water droplet CA above 90°, indicating low affinity toward interaction with water. In the case that a water droplet CA is > 150°, the water droplet preserves its integrity levitating over the solid surface, and the surface is termed super-hydrophobic () [Citation124]. Such surfaces have found application in chemical shielding, stain-proof coatings, membrane technologies and antifouling [Citation120].
In 2012, Deng et al. demonstrated the facile fabrication of a super-amphiphobic oil- and water-repellent coating composed of soot enclosed in SiO2 shell using flame and chemical vapour deposition (CVD) [Citation125]. They collected the soot particles by holding the substrate to be coated over a paraffin candle. To further improve the super-amphiphobicity, soot was coated with semi-fluorinated silane using CVD. They observed that the coated SiO2/soot particles exhibited enhanced heat resistance and had sufficient oil-repellent properties ().
In 2013, Stepien et al. fabricated a wear-resistant nanoparticle coating on paperboard using flame-made TiO2 and SiO2 hydrophobic coatings [Citation126]. They observed only small changes in the wettability of hydrophilic SiO2 and super-hydrophobic TiO2. They also observed that under severe abrasion tests, TiO2 maintained its hydrophobic property, but great amounts were removed. In comparison to TiO2, SiO2 coatings were more resistant to abrasion due to stronger inter-particle and particle-to-surface adhesion ().
In 2015, Mäkelä et al. studied the super-hydrophobicity of directly deposited nanostructured TiO2 and SiO2 using Liquid Flame Spray (LFS) for controlling the wettability of flexible and moving paper and paperboard materials () [Citation127]. They observed that TiO2 coated materials showed a switching property in their hydrophilicity/hydrophobicity. They attributed this behaviour to the photo-catalytic property of TiO2. Upon artificial daylight and heat treatment in the oven, the material changed from super-hydrophobic to super-hydrophilic. The switching behaviour was mainly due to the removal and regeneration of hydroxyl groups and organic contaminants on the surface of TiO2 coating.
In 2017, Wong et al. demonstrated in addition to water-repelling it was possible to fabricate super-oleophobic coatings that repel lower surface tension liquids by direct FSP deposition of SiO2 layers and their post functionalization by atmospheric pressure chemical vapour deposition () [Citation12]. presents an optical image of their super-oleophobic textures on clear glass demonstrating the beading of n-tetradecane, ethylene glycol, sunflower oil, and water with a characteristic > 150° CA. They also studied the wetting dynamics of different oil droplets and observed that the surface textures obtained by aerosol self-assembly provided multiple re-entrancy resulting in excellent rebounding dynamics and oil repulsion.
A key advantage of aerosol self-assembly is that it enables omnidirectional coating of non-flat substrates. Wong et al. demonstrated the fabrication of super-amphiphobic bionic proboscis (SAP) using flame-made SiO2 nanoparticles () [Citation128]. They observed that the needles were able to generate and release ultra-small nanoliter droplets of liquids due to the nanotexturing of the inner and outer surfaces of the commercial needle. They also observed that their SAPs demonstrated contamination-free handling of oil and water in both air and liquid environments.
4.3.3. Tissue engineering
With the increasing number of implants and wearable biomedical technologies and the limited donor availability for tissue and organ transplantation, nanostructured membranes and coatings are finding increasing applications in the field of tissue engineering and regenerative medicine (TERM) [Citation129]. As shown in , flame synthesized nanostructures are being investigate for TERM as a tool for enhancing intracellular delivery, cell differentiation [Citation130] and scaffold properties and also to enable real-time monitoring of cellular events [Citation131].
In 2013, Strobel et al. investigated the in-vitro influence of bioactive powdered glass nanoparticles with and without strontium (Sr) doping on the growth of human bone marrow stromal cells (hBMSCs) [Citation132]. Their studies were conducted on osteogenic differentiation of hBMSCs as represented in , it can be observed that the expression of osteocalcin and collagen type 1 was significantly enhanced for samples containing Sr doping.
In 2016, Nasiri et al. directly deposited an ultraporous biomimetic network of highly concentrated aerosol hydroxyapatite (HAp) using FSP on Titanium alloy disks (thickness = 1 mm, diameter = 15 mm) and studied its ability towards cellular adhesion and infiltration for improving osseointegration on metallic implants [Citation10]. The SEM micrographs shown in reveal that the osteoblast has firmly attached to the ultra porous coating and responded to its morphology by forming nanoscale filopodia. It can also be observed that the ultra porous neural network has higher osteoconductivity as compared to the plasma spray technique and can promote better osseointegration. The immunostaining response of the osteoblasts to the ultra porous network shows a positive response towards two prime cytoskeletal markers: F-Actin and Osteocalcin (). This suggests that ultra porous HAp networks obtained by aerosol deposition of flame-made nanoparticles may induce higher osteoconductivity than denser plasma spray coatings resulting in long-term better osseo-integration.
4.3.4. Antimicrobial
Nanostructures have also been widely used for antimicrobial, anti-inflammatory, and antiangiogenic properties. summarizes the main antimicrobial mechanisms of nanomaterials according to three steps: (1) formation of reactive oxygen species (ROS), (2) interaction with the cell membrane, and (3) release of ions into the cell.
In 2011, Sotiriou et al. investigated the use of flame-made Ag nanoparticles (Ag NPs) immobilized onto SiO2 for its antibacterial activity against Escherichia coli (E. coli) [Citation133]. They observed that Ag NPs synthesized using either combination of silver nitrate and HMDSO (bimodal size distribution) or silver acetate and HMDSO precursor (unimodal size distribution) showed similar antibacterial activity (, c). They concluded that smaller Ag NPs show stronger antibacterial activity due to more active sites and higher surface area and the bimodal size distribution does not really contribute to its antibacterial activity.
In 2017, Brobbey et al. studied the combinational use of LFS and roll-to-roll technology for direct deposition of Ag NPs on various paper substrates to examine its antibacterial property against E. coli [Citation134]. SEM analysis () shows the self-assembly and accumulation of silver nanoparticles onto PET paper substrates. It was observed that silver-coated paper with three flame sweeps could be used to decrease the growth of E. coli (). Their study showed that the combination of LFS and roll-to-roll technique could help in mass production of Ag NP coating/filters on various substrates without the need for additional chemical processing, to be further utilized for its antibacterial property in packaging or textile industries. Falco et al. studied the antimicrobial activity of flame synthesized TiO2 coatings against Candida Albicans, Aspergillus niger, Staphylococcus aureus, and Streptococcus mutans [Citation135]. They observed that the presence of higher amounts of anatase phase and larger exposed surface area of FSP-prepared TiO2 nanoparticles enhanced the antimicrobial activity over that of commercial P25 TiO2 catalysts ().
Membranes and coatings for device application have been previously fabricated using different techniques such as photolithography, hydrothermal synthesis, anodization, spin-coating CVD, and electro-deposition.
4.4. Chemical sensors and opto-electronic devices
A variety of techniques such as sol-gel, hydrothermal, sputtering, chemical vapour deposition, and so many more have been previously utilized for the fabrication of chemical sensors and different opto-electronic devices. Even though these techniques offer several advantages, these generally require different processes for the synthesis of nanomaterials and their integration into devices. However, the flame-based technique provides a unique advantage of rapid one-step fabrication, eliminating the need for additional processing steps with improved sensing responses, cost-effectiveness, and scalability. The high surface area of flame-made nanomaterials and films make them a potential candidate for application in chemical and biosensing as well as in optoelectronic applications, where the tunability of the interface charge-transfer and light absorption provide additional optimization potential. The porous nature of self-assembled aerosol films provides benefit for increasing the interaction between an analyte and the nanoparticle layers.
4.4.1. Chemiresistive
Chemiresistive gas sensors rely on the change of conductance of a nanostructured film due to the interaction of its surface with a target gas molecule () [Citation136]. With respect to other gas sensor technologies, chemiresistive sensors offer the advantages of a significantly smaller footprint and ease of miniaturization down to chip-sized devices, high sensitivity, and ease of use. Major challenges are the achievement of sufficient selectivity that hinders their broader application in complex gas mixtures. However, engineering the surface catalytic activity of metal oxide nanoparticles has enabled the successful development of sufficiently selective gas sensors for environmental [Citation137] and healthcare [Citation138,Citation139] applications.
Mädler et al. studied the chemiresistive gas sensing property of flame synthesized Pt/SnO2 nanoparticles for low concentration detection of CO [Citation140]. They fabricated their sensors via drop-coating of the FSP synthesized nanoparticles on alumina substrates with inter-digitated Pt electrodes on the front side and heater on the backside. They observed that 0.2 wt.% Pt doping resulted in the highest sensor signal along with shorter response time and large sensitivity. They attributed this behaviour to the low-temperature catalytic behaviour of Pt and dissociative adsorption of oxygen followed by oxygen reverse spill-over from the SnO2 onto Pt sites in isolated Pt-clusters ().
Righettoni et al. developed a highly selective and sensitive flame-made acetone (breath biomarker for diabetes) sensor composed of Si-doped WO3 [Citation141]. They fabricated their sensors via direct deposition of the FSP synthesized nanoparticles on alumina substrates with inter-digitated Au electrodes. They observed that 10 mmol% of Si doping within WO3 lattice, improved the selectivity towards acetone compared to other VOCs. They attributed this high selectivity towards the acentric structure of ε-WO3 along with strong interactions between the surface dipole of ε-WO3 and acetone molecules (). They also observed that under 90% relative humidity (similar to real breath conditions), the developed sensor showed pronounced response towards acetone.
Samerjai et al. compared the performance of WO3 and Pt-loaded WO3 towards selective sensing of H2 for environmental monitoring [Citation142]. Their sensing films were prepared by mixing the FSP synthesized nanoparticles along with ethyl cellulose and terpineol, which were subsequently spin-coated on alumina substrates with inter-digitated Au electrodes. illustrates the dynamic sensor response of different doping levels of Pt towards H2 indicating a typical n-type behaviour when pulsed with different concentrations of H2. They also observed that small amounts of Pt loading into the crystal lattice of WO3 could improve H2 sensing with rapid response and recovery at low operating temperatures (150°C) due low density of the adsorbed oxygen species along with the spill-over effect. They also evaluated the response towards other interfering volatile organic compounds (VOCs) and concluded that the developed sensor was highly selective towards H2 ().
Chen et al. reported a highly selective and stable H2S sensor using CuO-doped SnO2 sensing films fabricated using FSP [Citation143]. They prepared their sensors by uniformly coating a homogeneous mixture of FSP synthesized CuO-SnO2 nanoparticles and terpineol over alumina substrates with inter-digitated Pt electrodes on the front and heater at the back. Their fabricated sensor showed high selectivity towards H2S in the presence of other interfering VOCs (). The higher selective sensor response was attributed to the induction of Cu into the composite of CuO-SnO2 lattice.
Recently, the formation of nanoscale heterojunctions between a flame-made porous n-type semiconductor layer and a p-type semiconductor, sequentially deposited by sputtering, has shown the potential to enhance the room-temperature performance of chemiresistive devices. In 2018, Chen et al. demonstrated the use of ultra porous NiO-ZnO nanoheterojunction deposited on glass substrates with inter-digitated Pt electrodes, synthesized using a combination of FSP and magnetron sputtering for room temperature VOC sensing [Citation144]. Their nanoheterojunction sensor showed a ~ 4–5 times enhanced selective sensing towards ethanol with a limit of detection of 10 ppb compared to pure ZnO films at room temperature. They also observed that the sensitivity of the sensor was further enhanced (~11 times) with a limit of detection down to 2 ppb under an operation of 150°C with light illumination. They attributed the selective and sensitive sensing behaviour to the formation of p-n junction nanoheterojunctions and the ultra porous morphology. The p-n junction facilitated the separation of photogenerated electron-hole pair while the ultra porous morphology assisted rapid diffusion of VOCs.
4.4.2. Plasmonic
Utilization of the localized surface plasmon resonance (LSPR) of metallic nanostructures for chemical and bio-sensing has been widely studied for various applications including detection of biomolecules, VOCs, and environmental monitoring. LSPR relies on the collective resonant oscillations of conductive band electrons induced by the oscillating electric field of light [Citation145]. When a light wave propagates through the metallic nanostructures, surface plasmons that are in contact with the nanostructure are excited. As the resonance frequency of the plasmonic resonance also depends form the refractive index of the surrounding medium, this approach can be utilized for sensing changes in the chemical composition of the local environment surrounding the plasmonic nanomaterials () [Citation146].
Flame synthesis has been utilized for the rapid fabrication of two- and three-dimensional metasurfaces of applications in biomolecule and gas sensing. In 2010, Sotiriou et al. studied the toxicity effect of dry-coated SiO2 silver nanoparticles (SiO2-Ag NP) for plasmonic bio-detection of model protein: Bovine Serum Albumin (BSA) [Citation147]. They fabricated their biosensors using a one-step aerosol method followed by swirl injection of silica precursor vapour for the synthesis of SiO2-Ag NP, which were deposited on poly(L-lysine) coated glass substrates (). The resultant protocol developed hermetically coated Ag NP without any loss of plasmonic performance, thereby allowing the extended use of such nanoparticles for intracellular bio-applications such as targeted drug delivery and cancer cell therapy. From the adsorption kinetics, it is observed that compared to the fully coated devices, partially coated biosensors have low performance due to the reduced effective surface area as a result of flocculation or agglomeration ().
In 2019, Fusco et al. reported a one-step rapid high-temperature synthesis of Au metasurfaces using FSP on quartz substrate [Citation148]. They demonstrated the use of highly faceted monocrystalline Au nano-islands with tunable diameter, surface density, and spacing for LSPR-based gas sensing at room temperature. To further enhance the sensitivity of the optical gas sensor, they nanotextured the plasmonic metasurfaces with highly porous TiO2 fractal layers (~98% porosity) and observed that the integration of TiO2 enhanced the optical response to ethanol gas molecules by 400%. They attributed the enhanced sensing performance to the well-defined plasmonic-dielectric interface. Fusco et al. further explored the use of flame-made Au nano-islands for high-performing surface-enhanced Raman scattering (SERS) substrates [Citation149]. They observed that the enhanced SERS response was due to the coupling between laser radiation and plasmonic wavelength and the controlled faceting degree of the closely packed Au nano-islands.
In 2020, Fusco et al. reported for the first time the fabrication of a three-dimensional plasmonic media consisting of Au nanoparticles stochastically distributed in a fractal TiO2 matrix via the self-assembly of two flame-made aerosols [Citation150]. They further demonstrated the use of this plasmonic fractal metamaterial as an enhanced platform for optical detection of VOCs at room temperature. depicts the resonant Au-TiO2 photonic fractal metamaterial that was utilized for the detection of toluene, ethanol, and acetone. shows the dynamic response of the LSPR centroid of the material when exposed to different concentrations of toluene. The dynamic response shows the rapid response and recovery of the developed plasmonic sensor at room temperature. They attributed their enhanced sensing performance towards the ultra porous network with an increased surface area of the semiconducting matrix that facilitates gas penetration and adsorption throughout the fractal metamaterial. Tran-Phu et al. fabricated a plasmonic sensor based on three-dimensional Au-Bi2O3 for optical sensing of acetone [Citation151]. As represented in , the developed sensor showed high selectivity towards acetone. The strong response was attributed to the possible differences in binding energies and higher affinity towards acetone in comparison to other gases.
4.4.3. Photodetectors
Photodetectors are optoelectronic devices capable of detecting light and transduce it in an electrical or optical signal. Amongst electrical photodetectors, there are two main mechanisms: (i) photodiodes relies on the photogeneration of a voltage across a junction, often constituted by an n- and p-type semiconductors; (ii) photoresistors measure the change of resistance induced by light on a light-sensitive material, usually a semiconductor.
Flame synthesized photodetectors mostly rely on the photoresistor mechanism that is highly reliant on the film morphology and nanoparticle size () [Citation8]. High film porosity helps the penetration of oxygen into the lowest layers of the film ensuring that the entire film participates in the sensing reactions and avoids the formation of non-electron depleted or light-insensitive domains that could alter the electrical conductivity and photo-to dark-current ratio. In 2015, Nasiri et al. demonstrated a CMOS-compatible ultra porous ZnO network-based portable visible-blind UV photodetector [Citation8] made by directly deposited self-assembly of flame-made nanoparticle aerosols on inter-digitated Pt electrodes with Pt resistance temperature detector (RTD) on the front side and Pt heater at the back. and c shows the characteristic I–V response and responsivity of the thickest ZnO film (deposition time = 100 s) operated under different UV-A irradiations, respectively. They observed that all the samples had low dark current attributed to the ultraporous ZnO morphology and the electron-depleted composition of the network. From the responsivity spectra it was observed that by decreasing the wavelength, there was an increase in responsivity by a factor of 7, which can be credited to the wide bandgap of ZnO (3.37 eV) confirming that their ultra porous structure were visible-blind photodetectors. It was later reported that optimization of the density of grain boundaries and Debye length to ZnO particle size ratio of these ultra porous nanoparticle networks is key to achieve excellent UV photodetection performance [Citation153].
In 2016, Nasiri et al. showed a tunable bandwidth photodetector fabricating multiple integrated metal-oxide nanoparticle networks using FSP [Citation154]. The architecture of the device was composed of a directly deposited photoresponsive nanoparticle layer (ZnO), a dielectric spacer layer (SiO2) and a highly transparent tunable filter layer (TiO2) on glass substrates with inter-digitated Pt electrodes (). A major advantage of their device was the efficient blocking against UVB radiation and significant penetration of UVA light that did not affect the dark-current ratio (). They also observed that by varying the thickness of the filter layer facilitated in tuning the bandwidth of the device thereby allowing rapid fabrication of band-selective UVA photodetectors.
In 2017, a fast responsive UV-based photodetector composed of three-dimensional nano-heterojunction networks using NiO-ZnO p-n junction was also developed by Nasiri et al. [Citation155]. The photodetector was fabricated by direct deposition of ZnO aerosols using FSP on glass substrates with inter-digitated Pt electrodes followed by NiO deposition using a commercial magnetron sputtering system. Along with the rapid response, they also demonstrated that their photodetector could be operated under low power consumption, as illustrated in . They observed that under the operation of less than 2 mV, the device was able to detect UV densities below 80 µW /cm2 with large photo-to-dark current ratio, thereby enabling the use of such device as wearable UV-photodetectors.
5. Conclusion and future prospects
In the past two decades, the use of flame-assisted synthesis for the fabrication of three-dimensional nanostructures as functional components in devices has been explored for a variety of applications including catalysis, energy conversion, surface wetting, and sensors. A major strength of the flame fabrication route is the broad range of liquid precursors that can be accessed, making it a flexible tool for the exploration of innovative material compositions. Furthermore, the reproducible self-assembly of porous films comprising of three-dimensional nanoparticle network morphologies by spontaneous deposition of flame-made aerosols provides a powerful and flexible approach for the integration of non-silicon-based nanocomponents in devices.
We have reviewed the design of current liquid-fed flame synthesis reactors noting the critical need to ensure the evaporation/pyrolysis of the precursor prior to its nucleation in the liquid phase to achieve nanoparticle formation by the preferred vapour-to-cluster route. Engineering of an effective precursor atomization and composition is essential to this aim. The development of flame reactors has seen a convergence of design towards the use of two-fluid atomizers. Ultrasonic atomizers may provide an effective alternative by reducing the gas volume requirements. Simultaneous aerosol deposition and sintering have been demonstrated as a path to increase the mechanical stability of aerosol self-assembled films achieving control of the porosity from around 98%, expected for non-sintered layers, to 12% from highly sintered materials, such as BiVO4 utilized for photoelectrochemical water oxidation.
Major benefits of the flame-made route for fabrication and integration of nanomaterial in devices across various applications are the accessible surface area, the reproducible and scalable multi-scale porosity, and the incorporation of multi-components, such as plasmonic and semiconductor nanoparticles, with controllable stochastic distribution. These features have been used for the fabrication of highly active catalysts for photo-, electrochemical, and thermochemical applications. Similarly, flame-made films of the metal oxide semiconductor and noble metal nanoparticles have demonstrated excellent performance for chemiresistive gas sensing, where they provide both the catalytic active sites for detection of gas molecules and the transduction mechanism by modulation of the surface band bending. The mesoscale porosity of flame-made layers has shown potential to provide a re-entrant structure for super(de)wetting coatings and local topological clues for the promotion of cell growth on biomedical implants. Recent applications have also been reported for light detection and Li-S batteries, where the conversion of porous metal-oxide nanoparticle films in metal-organic frameworks provided an opportunity to fabricate thick and dense membranes.
Despite this broad range of benefit and potential, the use of flame-assisted synthesis for the integration of nanocomponents in device still face some challenges. Amongst those, the large amount of nanomaterial produced in the aerosol phase, the need to manage large gas volumes, and high temperature makes the integration of flame reactors in clean rooms and standard nanofabrication facilities difficult. The intrinsic very high porosity of aerosol self-assembled nanoparticle films at low temperature makes them fragile and prevents the use of such films in a liquid environment. Sufficient in situ sintering of the layers during deposition is not always possible for materials having low sintering rates and for substrates not able to withstand sufficiently high temperatures. Innovations are required in the design of flame-assisted synthesis to overcome these challenges and establish it as a standard fabrication route for the integration of nanomaterials in a variety of devices and applications.
Acknowledgments
This research was funded by and has been delivered in partnership with Our Health in Our Hands (OHIOH), a strategic initiative of the Australian National University, which aims to transform healthcare by developing new personalised health technologies and solutions in collaboration with patients, clinicians, and health care providers. A.T. gratefully acknowledges financial support from the North Atlantic Treaty Organization Science for Peace and Security Programme project AMOXES (#G5634) and ARENA (#AS008). A.T. also acknowledges the support of an Australian Research Council Future Fellowship (FT200100939) and DP190101864.
Disclosure statement
No potential conflict of interest was reported by the author(s).
Additional information
Funding
References
- Strobel R, Pratsinis SE. Flame aerosol synthesis of smart nanostructured materials. J Mater Chem. 2007;17:4743–50.
- Pratsinis SE. Flame aerosol synthesis of ceramic powders. Prog Energy Combust Sci. 1998;24:197–219.
- Tricoli A, Elmøe TD. Flame spray pyrolysis synthesis and aerosol deposition of nanoparticle films. AIChE J. 2012;58:3578–3588.
- Teoh WY, Amal R, Mädler L. Flame spray pyrolysis: an enabling technology for nanoparticles design and fabrication. Nanoscale. 2010;2:1324–1347.
- Liu Y, Zha S, Liu M. Novel nanostructured electrodes for solid oxide fuel cells fabricated by combustion chemical vapor deposition (CVD). Adv Mater. 2004;16:256–260.
- Mädler L, Roessler A, Pratsinis SE, et al. Direct formation of highly porous gas-sensing films by in situ thermophoretic deposition of flame-made Pt/SnO2 nanoparticles. Sens Actuators B Chem. 2006;114:283–295.
- Tricoli A, Graf M, Mayer F, et al. Micropatterning layers by flame aerosol deposition‐annealing. Adv Mater. 2008;20:3005–3010.
- Nasiri N, Bo R, Wang F, et al. Ultraporous electron‐depleted ZnO nanoparticle networks for highly sensitive portable visible‐blind UV photodetectors. Adv Mater. 2015;27:4336–4343.
- Liu G, Karuturi SK, Simonov AN, et al. Robust sub‐monolayers of Co3O4 nano‐islands: a Highly transparent morphology for efficient water oxidation catalysis. Adv Energy Mater. 2016;6:1600697.
- Nasiri N, Ceramidas A, Mukherjee S, et al. Ultra-porous nanoparticle networks: a biomimetic coating morphology for enhanced cellular response and infiltration. Sci Rep. 2016;6:1–11.
- Tricoli A, Righettoni M, Pratsinis SE. Anti-fogging nanofibrous SiO2 and nanostructured SiO2− TiO2 films made by rapid flame deposition and in situ annealing. Langmuir. 2009;25:12578–12584.
- Wong WS, Liu G, Nasiri N, et al. Omnidirectional self-assembly of transparent superoleophobic nanotextures. Acs Nano. 2017;11:587–596.
- Karthikeyan J, Berndt C, Tikkanen J, et al. Preparation of nanophase materials by thermal spray processing of liquid precursors. Nanostruct Mater. 1997;9:137–140.
- Mueller R, Mädler L, Pratsinis SE. Nanoparticle synthesis at high production rates by flame spray pyrolysis. Chem Eng Sci. 2003;58:1969–1976.
- Elmøe TD, Tricoli A, Grunwaldt J-D, et al. Filtration of nanoparticles: evolution of cake structure and pressure-drop. J Aerosol Sci. 2009;40:965–981.
- Nasiri N, Elmøe TD, Liu Y, et al. Self-assembly dynamics and accumulation mechanisms of ultra-fine nanoparticles. Nanoscale. 2015;7:9859–9867.
- Tricoli A, Righettoni M, Krumeich F, et al. Scalable flame synthesis of SiO2 nanowires: dynamics of growth. Nanotechnology. 2010;21:465604.
- Williams JO. Metal organic chemical vapor deposition (MOCVD) perspectives and prospects. Angew Chem Int Ed Engl. 1989;28:1110–1120.
- Strobel R, Pratsinis SE. Effect of solvent composition on oxide morphology during flame spray pyrolysis of metal nitrates. Phys Chem Chem Phys. 2011;13:9246–9252.
- Jossen R, Pratsinis SE, Stark WJ, et al. Criteria for flame‐spray synthesis of hollow, shell‐like, or inhomogeneous oxides. J Am Ceram Soc. 2005;88:1388–1393.
- Mäkelä J, Keskinen H, Forsblom T, et al. Generation of metal and metal oxide nanoparticles by liquid flame spray process. J Mater Sci. 2004;39:2783–2788.
- Mäkelä JM, Haapanen J, Harra J, et al. Liquid flame spray—a hydrogen-oxygen flame based method for nanoparticle synthesis and functional nanocoatings. KONA Powder Part J. 2017;34:141–154.
- Chung S-L, Katz JL. The counterflow diffusion flame burner: a new tool for the study of the nucleation of refractory compounds. Combust Flame. 1985;61:271–284.
- Xing Y, Koylu UO, Rosner DE. In situ light-scattering measurements of morphologically evolving flame-synthesized oxide nanoaggregates. Appl Opt. 1999;38:2686–2697.
- Masters, K.Spray Drying Handbook. Spray Drying Handbook, fourth edition; Halstead Press: New York, 1985.
- Chang H, Park J-H, Jang HD. Flame synthesis of silica nanoparticles by adopting two-fluid nozzle spray. Colloids Surf A Physicochem Eng Asp. 2008;313:140–144.
- Jung DS, Hong SK, Ju SH, et al. (CeTb) MgAl11O19 phosphor particles prepared by spray pyrolysis from spray solution containing citric acid and ethylene glycol. Jpn J Appl Phys. 2005;44:4975.
- Alam M, Flagan R. Controlled nucleation aerosol reactors: production of bulk silicon. Aerosol Sc Technol. 1986;5:237–248.
- Wiggers H, Starke R, Roth P. Silicon particle formation by pyrolysis of silane in a hot wall gasphase reactor. Chem Eng Technol. 2001;24:261–264.
- Bain Wasmund E, Saberi S, Coley KS. Modeling of an aerosol reactor for optimizing product properties. AIChE J. 2007;53:1429–1440.
- Weimer AW, Roach RP, Haney CN, et al. Rapid carbothermal reduction of boron oxide in a graphite transport reactor. AIChE J. 1991;37:759–768.
- Buesser B, Pratsinis SE. Design of nanomaterial synthesis by aerosol processes. Annu Rev Chem Biomol Eng. 2012;3:103–127.
- Mangolini L, Thimsen E, Kortshagen U. High-yield plasma synthesis of luminescent silicon nanocrystals. Nano Lett. 2005;5:655–659.
- Liao F, Girshick S, Mook W, et al. Superhard nanocrystalline silicon carbide films. Appl Phys Lett. 2005;86:171913.
- Phillips J, Luhrs CC, Richard M. Engineering particles using the aerosol-through-plasma method. IEEE Trans Plasma Sci. 2009;37:726–739.
- Vollath D, Szabo D. Synthesis of nanocrystalline MoS2 and WS2 in a microwave plasma. Mater Lett. 1998;35:236–244.
- van Erven J, Munao D, Fu Z, et al. The improvement and upscaling of a laser chemical vapor pyrolysis reactor. KONA Powder Part J. 2009;27:157–173.
- Gupta A, Swihart MT, Wiggers H. Luminescent colloidal dispersion of silicon quantum dots from microwave plasma synthesis: exploring the photoluminescence behavior across the visible spectrum. Adv Funct Mater. 2009;19:696–703.
- Gleiter H. Nanocrystalline materials. Adv Struct Funct Mater. 1991;33:1–37.
- Granqvist C, Buhrman R. Ultrafine metal particles. J Appl Phys. 1976;47:2200–2219.
- Guillou N, Nistor L, Fuess H, et al. Microstructural studies of nanocrystalline CeO2 produced by gas condensation. Nanostruct Mater. 1997;8:545–557.
- Ceylan A, Jastrzembski K, Shah SI. Enhanced solubility Ag-Cu nanoparticles and their thermal transport properties. Metall Mater Trans A. 2006;37:2033–2038.
- Osterwalder N, Capello C, Hungerbühler K, et al. Energy consumption during nanoparticle production: how economic is dry synthesis? J Nanopart Res. 2006;8:1–9.
- Meierhofer F, Fritsching U. Synthesis of metal oxide nanoparticles in flame sprays: review on process technology, modeling, and diagnostics. Energy Fuels. 2021;35:5495–5537.
- Schimmoeller B, Pratsinis SE, Baiker A. Flame aerosol synthesis of metal oxide catalysts with unprecedented structural and catalytic properties. ChemCatChem. 2011;3:1234–1256.
- Wiggers H. Novel material properties based on flame-synthesized nanomaterials. KONA Powder Part J. 2009;27:186–194.
- Zimmermann MB, Hilty FM. Nanocompounds of iron and zinc: their potential in nutrition. Nanoscale. 2011;3:2390–2398.
- Athanassiou EK, Grass RN, Stark WJ. Chemical aerosol engineering as a novel tool for material science: from oxides to salt and metal nanoparticles. Aerosol Sc Technol. 2010;44:161–172.
- Mueller R, Kammler HK, Pratsinis SE, et al. Non-agglomerated dry silica nanoparticles. Powder Technol. 2004;140:40–48.
- Karthikeyan J, Berndt C, Tikkanen J, et al. Nanomaterial powders and deposits prepared by flame spray processing of liquid precursors. Nanostruct Mater. 1997;8:61–74.
- Mekasuwandumrong O, Phothakwanpracha S, Jongsomjit B, et al. Influence of flame conditions on the dispersion of Pd on the flame spray-derived Pd/TiO2 nanoparticles. Powder Technol. 2011;210:328–331.
- Chew S, Patey TJ, Waser O, et al. Thin nanostructured LiMn2O4 films by flame spray deposition and in situ annealing method. J Power Sources. 2009;189:449–453.
- Rodriguez-Perez D, Castillo JL, Antoranz JC. Density scaling laws for the structure of granular deposits. Phys Rev E. 2007;76:011407.
- Rodríguez-Pérez D, Castillo JL, Antoranz JC. Relationship between particle deposit characteristics and the mechanism of particle arrival. Phys Rev E. 2005;72:021403.
- Mädler L, Lall AA, Friedlander SK. One-step aerosol synthesis of nanoparticle agglomerate films: simulation of film porosity and thickness. Nanotechnology. 2006;17:4783.
- Bandyopadhyaya R, Lall AA, Friedlander SK. Aerosol dynamics and the synthesis of fine solid particles. Powder Technol. 2004;139:193–199.
- Windeler RS, Lehtinen KE, Friedlander SK. Production of nanometer-sized metal oxide particles by gas phase reaction in a free jet. II: particle size and neck formation—comparison with theory. Aerosol Sc Technol. 1997;27:191–205.
- Tricoli A, Pratsinis SE. Dispersed nanoelectrode devices. Nat Nanotechnol. 2010;5:54–60.
- Tricoli A, Nasiri N, Chen H, et al. Ultra-rapid synthesis of highly porous and robust hierarchical ZnO films for dye sensitized solar cells. Solar Energy. 2016;136:553–559.
- Tran‐Phu T, Chen H, Bo R, et al. High‐temperature one‐step synthesis of efficient nanostructured bismuth vanadate photoanodes for water oxidation. Energy Technol. 2019;7:1801052.
- Thimsen E, Rastgar N, Biswas P. Nanostructured TiO2 films with controlled morphology synthesized in a single step process: performance of dye-sensitized solar cells and photo water splitting. J Phys Chem C. 2008;112:4134–4140.
- Gockeln M, Pokhrel S, Meierhofer F, et al. Fabrication and performance of Li4Ti5O12/C Li-ion battery electrodes using combined double flame spray pyrolysis and pressure-based lamination technique. J Power Sources. 2018;374:97–106.
- Blattmann CO, Pratsinis SE. Single-step fabrication of polymer nanocomposite films. Materials. 2018;11:1177.
- Tricoli A, Graf M, Pratsinis SE. Optimal doping for enhanced SnO2 sensitivity and thermal stability. Adv Funct Mater. 2008;18:1969–1976.
- Sahm T, Mädler L, Gurlo A, et al. Flame spray synthesis of tin dioxide nanoparticles for gas sensing. Sens Actuators B Chem. 2004;98:148–153.
- Abegg S, Klein Cerrejon D, Güntner AT, et al. Thickness optimization of highly porous flame-aerosol deposited WO3 films for NO2 sensing at PPB. Nanomaterials. 2020;10:1170.
- Krumeich F, Waser O, Pratsinis SE. Thermal annealing dynamics of carbon-coated LiFePO4 nanoparticles studied by in-situ analysis. J Solid State Chem. 2016;242:96–102.
- Waser O, Büchel R, Hintennach A, et al. Continuous flame aerosol synthesis of carbon-coated nano-LiFePO4 for Li-ion batteries. J Aerosol Sci. 2011;42:657–667.
- Hamid N, Wennig S, Hardt S, et al. High-capacity cathodes for lithium-ion batteries from nanostructured LiFePO4 synthesized by highly-flexible and scalable flame spray pyrolysis. J Power Sources. 2012;216:76–83.
- Hamid N, Wennig S, Heinzel A, et al. Influence of carbon content, particle size, and partial manganese substitution on the electrochemical performance of LiFe x Mn 1-x PO 4/carbon composites. Ionics. 2015;21:1857–1866.
- Schopf SO, Salameh S, Mädler L. Transfer of highly porous nanoparticle layers to various substrates through mechanical compression. Nanoscale. 2013;5:3764–3772.
- Liewhiran C, Tamaekong N, Wisitsoraat A, et al. Highly selective environmental sensors based on flame-spray-made SnO2 nanoparticles. Sens Actuators B Chem. 2012;163:51–60.
- Kammler HK, Mädler L. Characterization of fine dry powders. In: B Lee, S Komarneni, eds. Chemical Processing of Ceramics. 2nd ed.Boca Raton: CRC Press; 2005: 233- 265.
- Li S, Ren Y, Biswas P, et al. Flame aerosol synthesis of nanostructured materials and functional devices: processing, modeling, and diagnostics. Prog Energy Combust Sci. 2016;55:1–59.
- Zong Y, Li S, Niu F, et al. Direct synthesis of supported palladium catalysts for methane combustion by stagnation swirl flame. Proc Combust Inst. 2015;35:2249–2257.
- Zhang Y, Shuiqing L, Deng S, et al. Direct synthesis of nanostructured TiO2 films with controlled morphologies by stagnation swirl flames. J Aerosol Sci. 2012;44:71–82.
- Zachariah MR, Dimitriou P. Controlled nucleation in aerosol reactors for suppression of agglomerate formation: a numerical study. Aerosol Sc Technol. 1990;13:413–425.
- Wang J, Li S, Yan W, et al. Synthesis of TiO2 nanoparticles by premixed stagnation swirl flames. Proc Combust Inst. 2011;33:1925–1932.
- Johannessen T, Pratsinis SE, Livbjerg H. Computational fluid-particle dynamics for the flame synthesis of alumina particles. Chem Eng Sci. 2000;55:177–191.
- Mühlenweg H, Gutsch A, Schild A, et al. Process simulation of gas-to-particle-synthesis via population balances: investigation of three models. Chem Eng Sci. 2002;57:2305–2322.
- Shmakov A, Korobeinichev O, Knyazkov D, et al. Combustion chemistry of Ti (OC3H7) 4 in premixed flat burner-stabilized H2/O2/Ar flame at 1 atm. Proc Combust Inst. 2013;34:1143–1149.
- Tsantilis S, Kammler H, Pratsinis S. Population balance modeling of flame synthesis of titania nanoparticles. Chem Eng Sci. 2002;57:2139–2156.
- Tsantilis S, Pratsinis SE. Narrowing the size distribution of aerosol-made titania by surface growth and coagulation. J Aerosol Sci. 2004;35:405–420.
- Manuputty MY, Akroyd J, Mosbach S, et al. Modelling TiO2 formation in a stagnation flame using method of moments with interpolative closure. Combust Flame. 2017;178:135–147.
- Yan W, Li S, Zhang Y, et al. Effects of dipole moment and temperature on the interaction dynamics of titania nanoparticles during agglomeration. J Phys Chem C. 2010;114:10755–10760.
- Schwarz JA, Contescu C, Contescu A. Methods for preparation of catalytic materials. Chem Rev. 1995;95:477–510.
- Johannessen T, Jensen JR, Mosleh M, et al. Flame synthesis of nanoparticles: applications in catalysis and product/process engineering. Chem Eng Res Des. 2004;82:1444–1452.
- Stark WJ, Pratsinis SE, Baiker A. Heterogeneous catalysis by flame-made nanoparticles. CHIMIA Int J Chem. 2002;56:485–489.
- Belver C, Bedia J, Gómez-Avilés A, et al. Semiconductor photocatalysis for water purification. Nanoscale Mater Water Purif. 2019;581–651.
- Wang Y, Wang Q, Zhan X, et al. Visible light driven type II heterostructures and their enhanced photocatalysis properties: a review. Nanoscale. 2013;5:8326–8339.
- Murugan K, Subasri R, Rao T, et al. Synthesis, characterization and demonstration of self-cleaning TiO2 coatings on glass and glazed ceramic tiles. Prog Org Coat. 2013;76:1756–1760.
- Kim SC, Park Y-K, Nah JW. Property of a highly active bimetallic catalyst based on a supported manganese oxide for the complete oxidation of toluene. Powder Technol. 2014;266:292–298.
- Chiarello GL, Selli E, Forni L. Photocatalytic hydrogen production over flame spray pyrolysis-synthesised TiO2 and Au/TiO2. Appl Catal B Environ. 2008;84:332–339.
- Kho YK, Teoh WY, Iwase A, et al. Flame preparation of visible-light-responsive BiVO4 oxygen evolution photocatalysts with subsequent activation via aqueous route. ACS Appl Mater Interfaces. 2011;3:1997–2004.
- Xiong Z, Lei Z, Xu Z, et al. Flame spray pyrolysis synthesized ZnO/CeO2 nanocomposites for enhanced CO2 photocatalytic reduction under UV–Vis light irradiation. J CO2 Util. 2017;18:53–61.
- Abe Y, Laine RM. Photocatalytic plate‐like La2Ti2O7 nanoparticles synthesized via liquid‐feed flame spray pyrolysis (LF‐FSP) of metallo‐organic precursors. J Am Ceram Soc. 2020;103:4832–4839.
- Chen H, Bo R, Tran‐Phu T, et al. One‐Step Rapid and Scalable Flame Synthesis of Efficient WO3 Photoanodes for Water Splitting. ChemPlusChem. 2018;83:569–576.
- Chiang C-Y, Aroh K, Franson N, et al. Copper oxide nanoparticle made by flame spray pyrolysis for photoelectrochemical water splitting–Part II. Photoelectrochemical study. Int J Hydrogen Energy. 2011;36:15519–15526.
- Chen H, Mulmudi HK, Tricoli A. Flame spray pyrolysis for the one-step fabrication of transition metal oxide films: recent progress in electrochemical and photoelectrochemical water splitting. Chin Chem Lett. 2020;31:601–604.
- Ng YH, Iwase A, Kudo A, et al. Reducing graphene oxide on a visible-light BiVO4 photocatalyst for an enhanced photoelectrochemical water splitting. J Phys Chem Lett. 2010;1:2607–2612.
- Saeidi S, Amin NAS, Rahimpour MR. Hydrogenation of CO2 to value-added products—A review and potential future developments. J CO2 Util. 2014;5:66–81.
- Porosoff MD, Yan B, Chen JG. Catalytic reduction of CO 2 by H 2 for synthesis of CO, methanol and hydrocarbons: challenges and opportunities. Energy Environ Sci. 2016;9:62–73.
- Gao X, Liu G, Zhu Y, et al. Earth-abundant transition metal oxides with extraordinary reversible oxygen exchange capacity for efficient thermochemical synthesis of solar fuels. Nano Energy. 2018;50:347–358.
- Tada S, Larmier K, Büchel R, et al. Methanol synthesis via CO 2 hydrogenation over CuO–ZrO 2 prepared by two-nozzle flame spray pyrolysis. Catal Sci Technol. 2018;8:2056–2060.
- Wegner K, Medicus M, Schade E, et al. Tailoring catalytic properties of copper manganese oxide nanoparticles (Hopcalites‐2G) via flame spray pyrolysis. ChemCatChem. 2018;10:3914–3922.
- Lovell EC, Großman H, Horlyck J, et al. Asymmetrical double flame spray pyrolysis-designed SiO2/Ce0. 7Zr0. 3O2 for the dry reforming of methane. ACS Appl Mater Interfaces. 2019;11:25766–25777.
- Kirubakaran A, Jain S, Nema R. A review on fuel cell technologies and power electronic interface. Renew Sust Energ Rev. 2009;13:2430–2440.
- Choy K, Charojrochkul S, Steele B. Fabrication of cathode for solid oxide fuel cells using flame assisted vapour deposition technique. Solid State Ion. 1997;96:49–54.
- Seo DJ, Ryu KO, Park SB, et al. Synthesis and properties of Ce1− xGdxO2− x/2 solid solution prepared by flame spray pyrolysis. Mater Res Bull. 2006;41:359–366.
- Lee H, Kim TJ, Li C, et al. Flame aerosol synthesis of carbon-supported Pt–Ru catalysts for a fuel cell electrode. Int J Hydrogen Energy. 2014;39:14416–14420.
- Gockeln M, Glenneberg J, Busse M, et al. Flame aerosol deposited Li4Ti5O12 layers for flexible, thin film all-solid-state Li-ion batteries. Nano Energy. 2018;49:564–573.
- Abram C, Shan J, Yang X, et al. Flame aerosol synthesis and electrochemical characterization of Ni-rich layered cathode materials for Li-ion batteries. ACS Appl Energy Mater. 2019;2:1319–1329.
- Bo R, Taheri M, Liu B, et al. Hierarchical metal‐organic framework films with controllable meso/macroporosity. Adv Sci. 2020;7:2002368.
- Sun X, Radovanovic PV, Cui B. Advances in spinel Li 4 Ti 5 O 12 anode materials for lithium-ion batteries. New J Chem. 2015;39:38–63.
- Sazali N, Wan Salleh WN, Jamaludin AS, et al. New perspectives on fuel cell technology: a brief review. Membranes (Basel). 2020;10:99.
- Sel S, Duygulu O, Kadiroglu U, et al. Synthesis and characterization of nano-V2O5 by flame spray pyrolysis, and its cathodic performance in Li-ion rechargeable batteries. Appl Surf Sci. 2014;318:150–156.
- Stepien M, Saarinen JJ, Teisala H, et al. Adjustable wettability of paperboard by liquid flame spray nanoparticle deposition. Appl Surf Sci. 2011;257:1911–1917.
- Aromaa M, Arffman A, Suhonen H, et al. Atmospheric synthesis of superhydrophobic TiO2 nanoparticle deposits in a single step using Liquid Flame Spray. J Aerosol Sci. 2012;52:57–68.
- Bedi TS, Kumar S, Kumar R. Corrosion performance of hydroxyapatite and hydroxyapatite/titania bond coating for biomedical applications. Mater Res Express. 2019;7:015402.
- Wong WS, Tricoli A. Multiscale engineering and scalable fabrication of super (de) wetting Coatings. Adv Coat Mater. 2018;393.
- Jia Y, Liu G, Wu X, et al. Anti-fogging and anti-reflective silica nanofibrous film fabricated by seedless flame method. Mater Lett. 2013;108:200–203.
- Tuominen M, Teisala H, Aromaa M, et al. Creation of superhydrophilic surfaces of paper and board. J Adhesi Sci Technol. 2014;28:864–879.
- De Falco G, Ciardiello R, Commodo M, et al. TiO2 nanoparticle coatings with advanced antibacterial and hydrophilic properties prepared by flame aerosol synthesis and thermophoretic deposition. Surf Coat Technol. 2018;349:830–837.
- Wong WS, Tricoli A. Cassie-levitated droplets for distortion-free low-energy solid–liquid interactions. ACS Appl Mater Interfaces. 2018;10:13999–14007.
- Deng X, Mammen L, Butt H-J, et al. Candle soot as a template for a transparent robust superamphiphobic coating. Science. 2012;335:67–70.
- Stepien M, Chinga-Carrasco G, Saarinen JJ, et al. Wear resistance of nanoparticle coatings on paperboard. Wear. 2013;307:112–118.
- Mäkelä JM, Haapanen J, Aromaa M, et al. Roll-to-roll coating by liquid flame spray nanoparticle deposition. MRS Online Proc Lib (OPL). 2015;1747:37-42.
- Wong WS, Liu G, Tricoli A. Superamphiphobic bionic proboscis for contamination‐free manipulation of nano and core–shell droplets. Small. 2017;13:1603688.
- Vial S, Reis RL, Oliveira JM. Recent advances using gold nanoparticles as a promising multimodal tool for tissue engineering and regenerative medicine. Curr Opin Solid State Mater Sci. 2017;21:92–112.
- Ataol S, Tezcaner A, Duygulu O, et al. Synthesis and characterization of nanosized calcium phosphates by flame spray pyrolysis, and their effect on osteogenic differentiation of stem cells. J Nanopart Res. 2015;17:1–14.
- Rubio L, Pyrgiotakis G, Beltran-Huarac J, et al. Safer-by-design flame-sprayed silicon dioxide nanoparticles: the role of silanol content on ROS generation, surface activity and cytotoxicity. Part Fibre Toxicol. 2019;16:1–15.
- Strobel L, Hild N, Mohn D, et al. Novel strontium-doped bioactive glass nanoparticles enhance proliferation and osteogenic differentiation of human bone marrow stromal cells. J Nanopart Res. 2013;15:1–9.
- Sotiriou GA, Teleki A, Camenzind A, et al. Nanosilver on nanostructured silica: antibacterial activity and Ag surface area. Chem Eng J. 2011;170:547–554.
- Brobbey KJ, Haapanen J, Gunell M, et al. One-step flame synthesis of silver nanoparticles for roll-to-roll production of antibacterial paper. Appl Surf Sci. 2017;420:558–565.
- De Falco G, Porta A, Petrone A, et al. Antimicrobial activity of flame-synthesized nano-TiO 2 coatings. Environ Sci Nano. 2017;4:1095–1107.
- Tricoli A, Righettoni M, Teleki A. Semiconductor gas sensors: dry synthesis and application. Angew Chem. 2010;49:7632–7659.
- Wetchakun K, Samerjai T, Tamaekong N, et al. Semiconducting metal oxides as sensors for environmentally hazardous gases. Sens Actuators B Chem. 2011;160:580–591.
- Righettoni M, Tricoli A, Gass S, et al. Breath acetone monitoring by portable Si: WO3 gas sensors. Anal Chim Acta. 2012;738:69–75.
- Righettoni M, Tricoli A. Toward portable breath acetone analysis for diabetes detection. J Breath Res. 2011;5:037109.
- Mädler L, Sahm T, Gurlo A, et al. Sensing low concentrations of CO using flame-spray-made Pt/SnO2 nanoparticles. J Nanopart Res. 2006;8:783–796.
- Righettoni M, Tricoli A, Pratsinis SE. Si: WO3 sensors for highly selective detection of acetone for easy diagnosis of diabetes by breath analysis. Anal Chem. 2010;82:3581–3587.
- Samerjai T, Tamaekong N, Liewhiran C, et al. Selectivity towards H2 gas by flame-made Pt-loaded WO3 sensing films. Sens Actuators B Chem. 2011;157:290–297.
- Chen Z, Xu Z, Zhao H. Flame spray pyrolysis synthesis and H2S sensing properties of CuO-doped SnO2 nanoparticles. Proc Combust Inst. 2020;38:6743-6751.
- Chen H, Bo R, Shrestha A, et al. NiO–ZnO nanoheterojunction networks for room‐temperature volatile organic compounds sensing. Adv Opt Mater. 2018;6:1800677.
- Li H, Zhang L. Photocatalytic performance of different exposed crystal facets of BiOCl. Current Opin Green Sustainable Chem. 2017;6:48–56.
- Homola J. Surface plasmon resonance sensors for detection of chemical and biological species. Chem Rev. 2008;108:462–493.
- Sotiriou GA, Sannomiya T, Teleki A, et al. Non‐toxic dry‐coated nanosilver for plasmonic biosensors. Adv Funct Mater. 2010;20:4250–4257.
- Fusco Z, Rahmani M, Bo R, et al. High‐temperature large‐scale self‐assembly of highly faceted monocrystalline au metasurfaces. Adv Funct Mater. 2019;29:1806387.
- Fusco Z, Bo R, Wang Y, et al. Self-assembly of Au nano-islands with tuneable organized disorder for highly sensitive SERS. J Mater Chem C. 2019;7:6308–6316.
- Fusco Z, Rahmani M, Tran‐Phu T, et al. Photonic fractal metamaterials: a metal–semiconductor platform with enhanced volatile‐compound sensing performance. Adv Mater. 2020;32:2002471.
- Tran-Phu T, Daiyan R, Fusco Z, et al. Multifunctional nanostructures of Au–Bi 2 O 3 fractals for CO 2 reduction and optical sensing. ?J Mater Chem A. 2020;8:11233–11245.
- Tran‐Phu T, Daiyan R, Fusco Z, et al. Nanostructured β‐Bi2O3 fractals on carbon fibers for highly selective CO2 electroreduction to formate. Adv Funct Mater. 2020;30:1906478.
- Nasiri N, Bo R, Chen H, et al. Structural engineering of nano‐grain boundaries for low‐voltage UV‐photodetectors with gigantic photo‐to dark‐current ratios. Adv Opt Mater. 2016;4:1787–1795.
- Nasiri N, Bo R, Hung TF, et al. Tunable band‐selective UV‐photodetectors by 3D self‐assembly of heterogeneous nanoparticle networks. Adv Funct Mater. 2016;26:7359–7366.
- Nasiri N, Bo R, Fu L, et al. Three-dimensional nano-heterojunction networks: a highly performing structure for fast visible-blind UV photodetectors. Nanoscale. 2017;9:2059–2067.