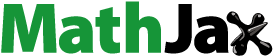
ABSTRACT
Three-dimensional topological insulators feature unconventional two-dimensional surface states, the carriers in which are helical Dirac fermions and protected from backscattering. Thus, they exhibit novel electronic response upon illuminate ultrashort and intense laser light. We briefly reviewed recent studies on ultrafast phenomena from the surface of the topological insulators driven by laser pulse ranging from visible to THz frequency. Ultrafast dynamics of Dirac fermions can be excited by helical photons and driven by strong light field. Many unique nonlinear behaviors have been demonstrated, such as the excitation of helicity-dependent photocurrent, the formation of Floquet-Bloch bands, lightwave-driven Dirac currents and the generation of optical high-harmonic emission. This review aimed at understanding the microscopic mechanism of the ultrafast charge and spin dynamics in topological surface states and its prospects for coherent manipulation of Dirac fermions by laser light.
GRAPHICAL ABSTRACT
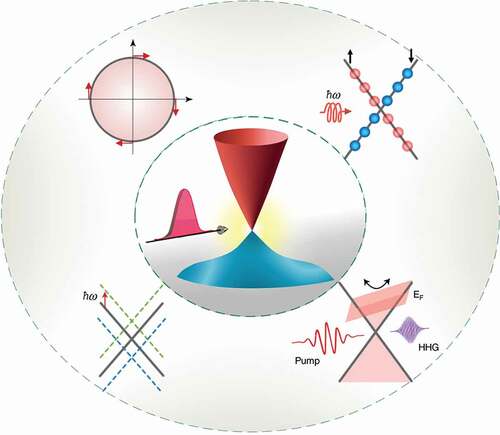
1. Introduction
Ever since its prediction and experimental demonstration, three-dimensional (3D) topological insulators (TIs) attracted much research attention over the past decade [Citation1–12]. In particular, the discovery of a series of wide band gap TIs, such as archetypical tetradymites Bi2Se3, Bi2Te3 and Sb2Te3 [Citation5–9], lighting up the study on topologically protected behavior at room temperature. These wide band gap 3D TIs are stoichiometric crystals synthesized by heavy p-block elements, crystallize in a rhombohedral structure with space group (
symmetry) [Citation8,Citation9,Citation11,Citation12].
The strong spin–orbit coupling of these heavy elements can modify the electronic band structure of the tetradymite compounds, in which the conduction and valence band are inverted in energy near the Γ point of the surface Brillouin zone [Citation9,Citation11,Citation12]. At the terminated surface of 3D TIs, the metallic surface states characterized by Dirac cone dispersion are developed in the inverted band gap ()). The fermi velocity of the quasi-relativistic surface band can reach to 5 × 106 m/s [Citation9,Citation12], close to that of graphene [Citation13]. In addition, the strong spin–orbit interaction can split the spin degeneracy of the Dirac bands, except for the Dirac point due to Kramers’ degeneracy. The spin of the Dirac fermion should lock with its momenta k, form the novel spin-momentum locking pattern () [Citation5,Citation14–17], due to the protection of time-reversal symmetry. Apart from that, backscattering that can reverse the crystal momentum (k to −k) is also prohibited due to topological protection [Citation7,Citation18]. Therefore, the topological surface state (TSS) that characterized by helical Dirac fermion is insensitive to nonmagnetic disorder, as long as the time-reversal symmetry is still preserved [Citation10,Citation11].
Figure 1. Topological surface states of 3D TIs. (a) Surface electronic band dispersion on Bi2Se3. BCB: bulk conduction band, BVB: bulk valence band, TSS: topological surface states and EF: Fermi energy. (b) Helical spin texture of Dirac electrons at the Fermi surface in Bi2Se3 and Bi2Te3, respectively. (c) Schematic of the Dirac cone with spin-momentum locking character. Reproduced from [Citation5, Citation10].
![Figure 1. Topological surface states of 3D TIs. (a) Surface electronic band dispersion on Bi2Se3. BCB: bulk conduction band, BVB: bulk valence band, TSS: topological surface states and EF: Fermi energy. (b) Helical spin texture of Dirac electrons at the Fermi surface in Bi2Se3 and Bi2Te3, respectively. (c) Schematic of the Dirac cone with spin-momentum locking character. Reproduced from [Citation5, Citation10].](/cms/asset/f7c136e4-4bf3-45a3-815c-3fe9c93a3f7b/tapx_a_2013134_f0001_oc.jpg)
The helical spin-polarized Dirac fermion promises unique electron transport character. For example, the charge current in TSS can convert to spin current via the Edelstein effect [Citation19–21]. Therefore, 3D TIs serves as an effective spin current source, benefit from the high charge-to-spin current conversion efficiency and large current-induced spin-transfer torque [Citation22–25]. However, to investigate and utilize the intrinsic properties of the TSS is rather challenging because the coexistence transport behavior conventional bulk carriers. To access the topological quantum transport regime, the chemical potential EF should intersect the Dirac node [Citation26,Citation27]. Under such a circumstance, the charge carriers are dominate by helical Dirac fermions.
Nevertheless, binary tetradymites Bi2Se3 and Bi2Te3 are commonly doped semiconductors, which are not bulk insulators as expected even in well stoichiometric crystals with high quality, owing to excess carriers introduced via Se or Te site defects in bulk crystals [Citation28,Citation29]. Efforts have been taken to improve the bulk insulation by well-controlled stoichiometry and defect chemistry to reduce the bulk carrier concentration. As a result, several high-crystallinity single crystals with bulk-insulation have been synthesized, such as Bi2-xSbxTe3-ySey (BSTS) and lightly Sn-doped BiSbTe2S (Sn-BSTS) [Citation30–33]. In these materials, Dirac point can be turned well separated from bulk valence and conduction bands, meanwhile the fermi energy level is located in the vicinity of the Dirac point. Therefore, it provides an ideal platform to explore the quantum transport properties in TSS.
Optical excitation on the surface 3D TIs by ultrafast laser pulse is expected to disentangle and manipulate the carrier transport from TSS and bulk band in contact free manner. Illuminated by a circularly polarized laser pulse, interband transitions in TSS are restricted by angular momentum selection rule and can lead to the generation of spin-polarized current [Citation34,Citation35]. Besides, strong laser field in mid-infrared (mid-IR) and terahertz (THz) frequency region can dominantly trigger the intraband motion of surface electrons near EF. These strong fields are capable to investigate the ultrafast transportation from TSS. Herein, we briefly review the recent theoretical and experimental progress on ultrafast dynamics of the helical Dirac fermions driven by intense laser pulses in the 3D TIs. This review article is organized as follows: in Section 2, we briefly review the excitation of laser light helicity-dependent photocurrent; in Section 3, we present the strong field dynamics of topological surface states driven by mid-IR to THz laser pulses; in Section 4, we summarize the recent advances in high-harmonic generation from 3D TIs. Finally, we will briefly summarize the further opportunities to explore the strong-field topological physics, and the prospects to construct the dissipationless electronics and spintronics based on 3D TIs.
2. Helicity-dependent photocurrent from topological insulators
Circularly polarized light can couple to the spin of the surface electrons, owing to the spin-momentum-locking feature of the TSS, where a specific spin is possessed for a certain electron momentum. A net spin polarization will be built up after the asymmetric optical excitation of helical laser light via angular momentum selection rules [Citation36]. This character brought a way to control the directional photocurrent by light helicity, which was proposed by P. Hosur in 2011 [Citation37]. They predicted that the ultrafast photocurrent can be produced through light-induced circular photogalvanic effect (CPGE) [Citation38,Citation39] on 3D TI surface. CPGE is a second-order nonlinear optical (NLO) processes, which is forbidden in inversion symmetric bulk of 3D TIs, such as Bi2Se3 and Bi2Te3. Thus, the photogalvanic currents should emerge from the crystal surface with broken inversion symmetry. They recognized that the nonvanishing Berry curvature and breaking of the threefold rotational symmetry are dispensable in the formation of surface photocurrent. The constraints can be satisfied by hexagonal warping effect of the TI [Citation40,Citation41] and external in-plane magnetic field or a strain.
J. W. McIver et al. [Citation34] first demonstrated the experimental observation of the photocurrents that originates from the nonequilibrium distribution of the topological helical Dirac fermions, when illuminate by circularly polarized light. They show the ability to control the direction of the photocurrents through manipulate the helicity of the laser light. In advance, they discriminated two microscopic mechanisms of photocurrents generation, that is, photogalvanic effect and photon drag effect [Citation42], which can stem from both the TSS and bulk states. Moreover, the unbalanced carrier distribution in Dirac cone will induce spin-polarized photocurrent owing to the helical spin texture. The spin-polarized photocurrent can also be generated and manipulated by linearly polarized light, which has been confirmed in spin-ARPES studies by Z.-H. Zhu et al. [Citation43]. They demonstrated a completely reversal of the spin polarization upon the rotation of light polarization and change of the photon energy. Furthermore, surface currents can also be produced from Dirac fermions that transitions into higher-lying Dirac cones [Citation44–46] and asymmetric scattering process under the acceleration of the THz electric field [Citation47]. For example, H. Soifer et al. [Citation46] demonstrated the photocurrents excitation via resonant optical transitions between the occupied and unoccupied TSS with helical spin texture, by using circularly polarized laser pulse.
On that basis, C. Kastl et al. [Citation35] shown the ability to control the ultrafast surface photocurrents on picosecond timescale with near-unity fidelity. They verified that the ultrafast time resolution provides an effective means to separate the currents produced from spin-polarized surface and spin-degenerate bulk. Through time-of-flight analysis, they found that the photogenerated hot electrons can transport in a speed comparable to the Fermi velocity of the Dirac cone in Bi2Se3, which lay the foundation of high-speed electronics based on TIs.
To further look inside the formation of surface photocurrents, time resolution down to femtosecond timescale is needed. L. Braun et al. [Citation48] studied the ultrafast evolution of surface photocurrents in Ca-doped Bi2Se3 through broadband THz spectroscopy, driven by ultrashort laser pulses. They found that the photocurrent response was dominated by an ultrafast charge displacement along the Se–Bi bonds that spatially confined in about first two quintuple layers (~2 nm) [Citation11, Citation12]. This shift current [Citation49] decays very fast from the initial asymmetric excitation to final isotropic distribution, on the timescale of 20 fs. Hopefully, the local transient THz field emitted from the shift current can be used as an alternative tool in manipulate the spin-polarized current in 3D TIs.
It should be noted that the above discussions of the observed photocurrents are based on the in-plane spin texture of TSS. For the Dirac fermions already exist or excite away from the Γ point in surface Brillouin zone, the surface current generated from the out-of-plane spin components that tilted by the hexagonal warping effect should be considered [Citation41]. Y. Q. Huang et al. [Citation50] studied the surface photocurrents originate from out-of-plane spin texture. They found that the spin-polarized currents generate from in-plane and out-of-plane spin polarization have different directionality. In this scenario, photocurrents from out-of-plane spin texture can be manipulated by both the laser light helicity and external magnetic field.
Recently, X. Sun et al. [Citation51] showed that the circular photogalvanic response can be enhanced in artificial nanostructured TIs surface at room temperature. They observed a giant enhancement of helicity-dependent photocurrents by structural design. In the experiments, laser pulse with controlled polarization incident on to a Bi1.5Sb0.5Te1.8Se1.2 (BSTS) sample patterned with square ring metamaterial array (). The increased absorption of the irradiate laser photons in the resonant nanostructure greatly enhances the photoexcitation of spin-polarized electrons, as shown in ). Hence, the surface states are asymmetrically depopulated in k-space due to the spin-selective photoexcitation, lead to the giant enhancement of photocurrents from the CPGE effect. They observed more than one order magnitude increase of the photocurrent from BSTS metamaterial than unstructured BSTS surface, as shown in . More importantly, they verified the potential ability to manipulate the spin transport in 3D TIs by metamaterial design.
Figure 2. Helicity-dependent photocurrent generation from CPGE. (a) Experimental setup of the helicity-dependent photocurrent generation, right: the image of the BSTS metamaterial patterned with square ring array. (b) Illustration of the structural enhanced CPGE. (c),(d) Photocurrents measured on an unstructured BSTS surface (c), and BSTS metamaterial (d). Reproduced from [Citation51].
![Figure 2. Helicity-dependent photocurrent generation from CPGE. (a) Experimental setup of the helicity-dependent photocurrent generation, right: the image of the BSTS metamaterial patterned with square ring array. (b) Illustration of the structural enhanced CPGE. (c),(d) Photocurrents measured on an unstructured BSTS surface (c), and BSTS metamaterial (d). Reproduced from [Citation51].](/cms/asset/c2b77141-6000-47b1-ae15-49a0c221349b/tapx_a_2013134_f0002_oc.jpg)
In the above studies, laser pulses at visible and near-infrared (near-IR) range can intrigue successive interband excitations from both lower lying and high lying bands far away from fermi surface, EF, regardless of the electronic state is submerge in the bulk or confined near the surface [Citation45]. Besides, surface-bulk coupling will result an unavoidable dissipative channel, due to strong scattering between Dirac electrons and bulk carriers or phonons [Citation52, Citation53]. It will reduce the transport lifetime of the Dirac electrons. Therefore, the photoexcited currents may not directly manifest the unique transport properties of TSS, because of the complex surface and bulk entanglement discussed above. Therefore, ultrashort laser pulses with frequency below the bulk band gap of 3D TIs, should be adopted, which are capable of the exclusive study of the transport properties of Dirac fermions.
3. Strong field dynamics of topological surface states
Laser pulses ranging from mid-IR to THz frequency are suitable for excites intraband or interband motion of surface electrons near the fermi level, EF, while suppress the interband excitation from bulk valence to conduction bands and scatteration between bulk and TSS. In this scenario, the surface transport should be enhanced because of the reduced population transfer between surface and bulk bands.
3.1 Mid-IR to THz-driven nonlinearity
Surface states in TIs, characterized by quasi-relativistic Dirac dispersion, are expected to possess strong nonlinear response driven by intense laser fields. Such the exceptional nonlinear behavior was predicted and demonstrated in graphene, which also possess massless Dirac fermions [Citation54–57]. F. Giorgianni et al. [Citation58] studied the THz frequency nonlinear absorption of the Dirac electrons in prototypical TI Bi2Se3. They observed apparent increase of the overall transmittance of the THz amplitude when strong THz field irradiate on a Bi2Se3 film. They ascribed the reduced absorption to THz field-induced transparency. The field-induced transparency displays highly nonlinear dependence on THz field, which will saturate when the field strength reached to about 1 MV/cm. Besides, they proved that the electromagnetic transparency is directly related to transportation in TSS of Bi2Se3, while not been observed in trivial band insulator (Bi0.9In0.1)2Se3, which shares the common rhombohedral structure with Bi2Se3 but exhibit trivial topology without the presence of massless Dirac fermions on its surface [Citation59,Citation60]. The strong nonlinear optical response of the Dirac fermions, hence, responsible for the reduced absorption of strong THz fields.
Due to the narrow band gap character of 3D TIs, the electron transport properties of TSS are inevitably entangled with that of bulk band. Therefore, how to decouple the two contributions, selectively excite and control the intrinsic topological transport, is an important issue. It has been proved that time-resolved THz spectroscopy is an effective tool in investigate ultrafast dynamics of the hot Dirac electrons in 3D TIs [Citation61,Citation62]. For example, by using optical-pump terahertz-probe technique. But the high photon energy of optical pump used in these studies can complicate the transitions between the bulk bands and surface states. For this reason, L. Luo et al. [Citation63] provided a perspective to enhance and manipulate the ultrafast surface transport by adopting intense mid-IR and THz pulses to drive the ultrafast dynamics in Bi2Se3 (). A broadband THz probe was used to extract the pump-induced conductivity spectra in THz range at certain pump-probe delays. In the pump-probe experiments they found that the decay of the photoinduced THz conductivity varies for different THz photon energy, which will result a frequency-dependent carrier cooling times, as shown in ). By fitting the dynamical THz conductivity using Drude model, they shown that the contributions from the Dirac electrons and bulk carriers are markedly different (), thus, provide a means to differentiate them. They demonstrated that topological enhancement of the surface transport is because of the suppression of surface-bulk charge transfer, and thus reduced the scattering rate of the Dirac electrons in comparison with the bulk states. The enhanced surface transport provides circumstantial evidence for the topological protection of TSS that forbids the back scattering.
Figure 3. Ultrafast manipulation surface transportation driven by mid-IR and THz pulses in Bi2Se3. (a) Experimental schematics of the mid-IR and THz pump-THz probe process in Bi2Se3 film. (b) Frequency-dependent THz conductivity induced by strong mid-IR pump pulse at various pump-probe delays. (c) is extract from (b) at pump-probe delay of 1 ps, the solid and dashed lines are the fitted curves including surface and bulk contributions. (d) surface transport lifetimes as a function of THz driving field strength, which shows a clear transition at ~180 kV/cm. Inset: THz field induced bulk transport lifetime. Reproduced from [Citation63,Citation64].
![Figure 3. Ultrafast manipulation surface transportation driven by mid-IR and THz pulses in Bi2Se3. (a) Experimental schematics of the mid-IR and THz pump-THz probe process in Bi2Se3 film. (b) Frequency-dependent THz conductivity induced by strong mid-IR pump pulse at various pump-probe delays. (c) is extract from (b) at pump-probe delay of 1 ps, the solid and dashed lines are the fitted curves including surface and bulk contributions. (d) surface transport lifetimes as a function of THz driving field strength, which shows a clear transition at ~180 kV/cm. Inset: THz field induced bulk transport lifetime. Reproduced from [Citation63,Citation64].](/cms/asset/fed0690e-3993-467f-9754-1deee79753f2/tapx_a_2013134_f0003_oc.jpg)
On this basis, X. Yang et al. [Citation64] also proposed a scheme to disentangle the surface-bulk coupling via the THz vibration coherence in 3D TI. This can be realized through driven coherent phonon oscillations of the mode in the Bi2Se3 surface by an intense single-cycle THz pulse. The presence of periodically coherent lattice vibration can reduce the scattering of surface Dirac fermion with the bulk state, via the so called ‘phase space contraction’ mechanism assisted by the strong spin–orbit coupling. The THz-field-driven phonon-dressed topological protected states thus prolong the surface transport distance. Besides, the coherent phonon excitations display a threshold-like behavior, the emergence of which requires the THz field exceeds ~180 kV/cm, as shown in ).
3.2 Floquet-Bloch states on the surface of topological insulators
Coherent light and matter interaction has the potential of manipulate or engineer novel quantum states of condense matter. When a strong laser field irradiate on the solid-state systems, the photons will hybrid with the electronic bands and lead to the formation of Floquet-Bloch states. The Floquet-Bloch state possess both the characters of time periodic Floquet state [Citation65], the energies of which are evenly spaced by the drive photon energy, and the spatial translation periodicity of the crystalline lattice.
The hybrid of time-periodic potential of the driven field with the surface Dirac fermions of 3D TIs is a rather intriguing issue. Y. H. Wang et al. [Citation66] first reported the Floquet-engineered topological phase excited on the surface of Bi2Se3. By adopting polarization tunable strong mid-IR pulse with photon energy smaller than the bulk band gap (~ 300 meV), they observed the emergence of replicas of the linear Dirac bands appear above and below the original Dirac cone, which is a clear signature of Floquet-Bloch states in TSS, as shown in –(d). This phenomenon was recorded by time- and angle-resolved photoemission spectroscopy (tr-ARPES) [Citation67]. For linearly polarized mid-IR pulse, several small band gaps equal to 62 meV were displayed centered at the momenta ±ω/2vF, vF is the Fermi velocity. However, the spin degeneracy at the Dirac is still preserved, where the upper- and lower-branch of the surface state contact with each other, as displayed in .
Figure 4. Observation of Floquet-Bloch bands on the surface of Bi2Se3. (a) Photoemission spectra measured pumped by s-polarized mid-IR pulse, red arrows indicate the avoided crossings between neighboring Floquet states. (b) Photoemission spectrum subtracted from the laser-field-driven spectrum in (a) and the unperturbed spectrum before the advent of mid-IR pulse, dashed lines illustrate the avoid crossing gaps. (c) Schematic illustration of the experimentally observed Floquet-Bloch bands. (b)-(f) The same as in (a)-(c) but for circular polarized mid-IR pump. A band gap opening in Dirac point is depicted in (f). Reproduced from [Citation66].
![Figure 4. Observation of Floquet-Bloch bands on the surface of Bi2Se3. (a) Photoemission spectra measured pumped by s-polarized mid-IR pulse, red arrows indicate the avoided crossings between neighboring Floquet states. (b) Photoemission spectrum subtracted from the laser-field-driven spectrum in (a) and the unperturbed spectrum before the advent of mid-IR pulse, dashed lines illustrate the avoid crossing gaps. (c) Schematic illustration of the experimentally observed Floquet-Bloch bands. (b)-(f) The same as in (a)-(c) but for circular polarized mid-IR pump. A band gap opening in Dirac point is depicted in (f). Reproduced from [Citation66].](/cms/asset/1c4b8de7-c4bb-4df3-ad03-45eca5536067/tapx_a_2013134_f0004_oc.jpg)
When the pump pulse polarization changed from linear to circular, a dynamical band gap opening (2κ = 53 meV) around Γ Point was clearly seen (). This is a clear signature of time-reversal symmetry breaking and can attribute to the coupling between circularly polarized laser field and the TSS. The broken time-reversal symmetry together with the nontrivial band topology can lead to an exotic quantum Hall state in TSS – the quantum anomalous Hall effect (QAHE) [Citation26]. The QAHE is characterized by a quantum Hall effect without the presence of external magnetic field. H. Xu et al. [Citation68] theoretically studied the QAHE in gapless surface states of 3D TIs driven by circular polarized laser pulse. The metallic surface states are naturally gapped as a consequence of broken time-reversal symmetry. The Chern number of the light-dressed surface electron system can be tuned by the laser light helicity [Citation69,Citation70]. The light-induced anomalous Hall effect has been recently observed from the Floquet-engineered topological band in graphene [Citation70].
In ARPES experiments, the free electrons released from the TSS can be dressed by the near surface mid-IR field and form the so-called Volkov states. The Volkov states can coexists with the Floquet-Bloch states, both characterized by replica bands spaced by the pump photon energy. F. Mahmood et al. [Citation71] demonstrated a scheme to separate these two effects, by discriminating the polarization dependence and avoided-crossing gaps signatures of the Floquet-Bloch states in photoelectron spectra. They shown the capability to selectively enhance the Floquet-Bloch states, meanwhile, inhabit the Volkov states, by simply tuning the polarization direction of the mid-IR field.
3.3 THz-field-driven Dirac currents
As mentioned above, laser pulses with photon energy larger than the bulk band gap of 3D TIs are capable of excite the direct interband transition in the TSS, from the occupied to the unoccupied states across the Dirac point [Citation72]. The transition may induce unbalanced distribution of electron states in momentum (k) space. K. Kuroda et al. [Citation73] has observed asymmetrical redistribution of surface electrons in Sb2Te3 by using tr-ARPES, because of the coupling between mid-IR pulse and surface state. The largest population asymmetry was observed for p-polarized mid-IR pulses that parallel to along Γ-K direction of the surface Brillouin. The k-space population asymmetry directly results in a transient photocurrent that flow in TSS [Citation74].
If the driving pulse is further extended to THz frequency, the intraband electron transport in TSS will be dominant. The slow varying carrier of the THz field make it possible to look inside the ultrafast dynamics of the surface states within a fraction of a laser cycle. In the strong field regime, the motion of electrons can be treated in semiclassical equation of motion, the group velocity of the electron wave packet in an electronic band is
where k is the crystal momentum, ε(k) and Ω(k) are the energy dispersion and Berry curvature, respectively. The time-dependent crystal momentum takes the from where
is the vector potential of the laser field. This equation describes the acceleration of Dirac fermions in the presence of driving field, in which the first term describes motion of electrons parallel to the driven field polarization, which is proportional to the group velocity,
,
is the Fermi velocity of surface Dirac cone. The second term describes the anomalous velocity that perpendicular to the driven field polarization, associate with the Berry curvature.
The transport of the electron wave packet is described by Boltzmann equation
where μ is the Fermi level, is a phenomenological relaxation time. For small relaxation times, the electron distribution can be simplified as
where kB is the Boltzmann constant. Therefore, the strong redistribution of the electronic states can induce measurable macroscopic current. If we only consider the group velocity (only the first term in EquationEq. (1(1)
(1) )) of the Dirac fermions, the current density in Dirac type dispersion is written as
How to steer the unique dynamics of Dirac fermions in 3D TIs by strong laser field is a rather intriguing topic. It has been demonstrated that in graphene the current flow in Dirac cone can be controlled by the carrier wave of the phase-stabilized few-cycle laser pulses [Citation75,Citation76]. Recently, J. Reimann et al. [Citation77] has realized the observation of the THz field-driven transient electron distribution in Bi2Te3 surface (). The fermi surface of the Bi2Te3 located 200 meV above the Dirac point but below the conduction band minimum. Thus, the redistribution of surface electrons in subcycle timescale can be clearly seen without the coupling between surface and bulk bands. An intense THz pulse together with an ultrashort UV pule in combine were adopted as pump and probe. The THz field modulated photoelectrons that released from the Bi2Te3 surface were imaged by an tr-ARPES. The instantaneous image of the surface electron occupation for three different pump-probe delays are displayed in –(d). The out-of-equilibrium redistribution of the accelerated surface electrons can be reflected from the dynamical slanted fermi surface in momentum space. As shown in –(d), the Dirac cone branches are obviously tilt alternately, for the interaction of positive and negative THz field amplitude, respectively, in compare with the electron distribution before the advent of the THz transient ().
Figure 5. THz-field-driven Dirac currents in the TSS of Bi2Te3. (a) Schematic of the experimental layout, THz-field-driven Dirac electrons measured by UV pulse excited photoemission spectroscopy. (b)-(d) Acceleration of the surface electrons in Dirac band driven by THz field at different delay times. (e) Dirac current density evolve with the THz pump-UV probe delay. Reproduced from [Citation77].
![Figure 5. THz-field-driven Dirac currents in the TSS of Bi2Te3. (a) Schematic of the experimental layout, THz-field-driven Dirac electrons measured by UV pulse excited photoemission spectroscopy. (b)-(d) Acceleration of the surface electrons in Dirac band driven by THz field at different delay times. (e) Dirac current density evolve with the THz pump-UV probe delay. Reproduced from [Citation77].](/cms/asset/0217d56a-9b93-47d6-ac03-53789a912fb4/tapx_a_2013134_f0005_oc.jpg)
The dynamical motion of surface electrons in quasi-relativistic electronic bands can inevitably lead to significant current surge in subcycle timescale of the THz field. The ballistic motion of electrons in TIs surface can reach to several hundred nanometers benefit from the special features of character – spin–momentum locking and near linear band dispersion in TSS, which leads to an inertia-free charge currents follows in TSS. The retrieved current density has extremely large value, with maximum peak density reached up to 2 A/cm (). The observation of the marvelous ballistic Dirac current on the two-dimensional surface of the 3D TI in subcycle timescale, should facilitate the development of extremely fast and dissipation less electronics devices.
Very recently, P. Di Pietro et al. [Citation78] shown that the tunability of the Dirac plasmon in TSS can be achieved by strong THz field. In their experiments an intense THz pulse, with field strength in MV/cm range, was irradiate on an artificially patterned Bi2Se3 ribbon. The plasmon excited in Dirac bands can be strongly renormalized by the strong THz field, lead to obvious shift of the characteristic plasmon excitation frequency.
4. High-harmonic generation from topological surface states
The strong laser-field-driven ultrafast electron dynamics in solid systems can bring may novel nonlinear optical phenomena, such as the high-harmonic generation (HHG). The generated high-harmonic spectrum is characterized by a series of frequency combs spaced by single or twice of the pump photon energy [Citation79–86]. HHG is rather sensitive to the electronic structure of solids, the underlying mechanisms mainly attribute to the varieties of band structure of solids and therein nonlinear electronic response of inter- and intra-band dynamics [Citation81,Citation83,Citation86]. For example, in solid systems with broken inversion symmetry, the roles of interband quantum interference [Citation80], Berry phase [Citation84], complex transition dipole momentum [Citation87–89], shift vector [Citation90] and the ‘smooth-periodic’ gauge based on band theory [Citation91] are important in studying the laser-field-driven HHG.
HHG from the graphene, a typical 2D Dirac fermion system, have been reported with unconventional property [Citation57,Citation92,Citation93], such as, abnormal pump ellipticity dependence of the HHG yield [Citation92], and HHG with extreme efficiency driven by strong THz field [Citation93]. One of the import reasons is that for electrons transport in Dirac cone-shape energy dispersion, the group velocity is strongly dependent on the slope of band dispersion and the sign of k vector. Especially, when an electron accelerates across the Dirac point, the group velocity should change direction sharply. Therefore, this can lead to strongly nonlinear electromagnetic response and the results in the emission of high-harmonics.
As mentioned above, the TSS is derive from the bulk states that features strong spin-orbit coupling and time-reversal symmetry, which is confined in atomic thin surface layer. In momentum space the surface states are connect to the bulk bands across the insulating band gap. The inevitable entanglement of the TSS and bulk states makes the resultant high-harmonic emission much more complicated, compared with topologically trivial materials. Hence, how to disentangle HHG from surface and bulk states is a crucial issue that prior to the study of the unique properties of HHG from TSS.
Several studies have focused on the studies of HHG from 3D TIs. H. K. Avetissian et al. proposed that high harmonics can be generated via multiphoton excitation process in topological insulator [Citation94]. L. Jia et al. theoretically investigated the HHG from pristine undoped and magnetically doped topological insulators and revealed that crystal symmetry operation and laser polarization played indispensable role in shaping the high-harmonic spectra [Citation95].
Very recently, experimental progresses have also brought a surprise in demonstrated the observation of HHG on 3D TIs. In these studies, pump pulses with photon energy ranging from mid-IR to multi-THz range are adopted to intrigue the electron dynamics in TSS. Y. Bai et al. [Citation96] demonstrated the observation of HHG from intrinsic topological insulator material, BiSbTeSe2 (BSTS) [Citation31,Citation82,Citation97] (). High-harmonic spectra of up to ninth order were observed, when linearly polarized mid-IR pulse was adopted to illuminate on the BSTS surface, as shown in ). The presence of even-order harmonics is a direct consequence of the broken inversion symmetry in BSTS surface. Besides, we measured the polarization components as a function of the crystal orientation. We found that the magnitudes and polarizations of the even- and odd-order harmonics, modulated by the crystal azimuth orientation, behaves differently. The polarization of even-order harmonics is switched from parallel to perpendicular direction as crystal orientation changed from to
, which is restricted by surface mirror symmetry. However, the odd-order harmonics dose not subject to such symmetry requirements (), reflect their bulk origin.
Figure 6. HHG from intrinsic TI BSTS. (a) Schematic of the experimental set-up. A mid-infrared pulse is focused onto the BSTS surface at a small angle of incidence, the HHG are measured in reflection geometry. (b) Typical high-harmonic spectrum generated from BSTS surface. (c) Orientation dependence of the parallel components of H4 and H5, respectively. (d) Shows the perpendicular components of H4 and H5. Reproduced from [Citation96].
![Figure 6. HHG from intrinsic TI BSTS. (a) Schematic of the experimental set-up. A mid-infrared pulse is focused onto the BSTS surface at a small angle of incidence, the HHG are measured in reflection geometry. (b) Typical high-harmonic spectrum generated from BSTS surface. (c) Orientation dependence of the parallel components of H4 and H5, respectively. (d) Shows the perpendicular components of H4 and H5. Reproduced from [Citation96].](/cms/asset/64ddc979-81dd-4f54-ae89-1a5e830740c7/tapx_a_2013134_f0006_oc.jpg)
In addition, we also verified other source of even-order harmonic generation. Because the well-known two-dimensional electron gasses (2DEG) can be developed from the inversion asymmetric surface due to band bending [Citation98–100]. The 2DEG can coexist with TSS on TI surface. We have examined the contribution from TSS and 2DEG through measure the time evolution of HHG from cleaved BSTS surface. Through compare the time evolution signature of harmonic yield for three kinds of crystals with different topology and bulk carrier density, namely, BSTS the intrinsic TI, Bi2Se3 the TI with large bulk conduction, and (Bi0.8In0.2) 2Se3 normal band insulator [Citation59,Citation60]. We conclude that the recorded even-order harmonic spectra from the freshly cleaved BSTS surface are produced from TSS.
As indicated in Section 3.3, the dynamical asymmetric redistribution of the Dirac fermions, driven by strong laser field, inevitably lead to electrical current. More importantly, due to the spin-momentum locking of TSS, the asymmetric flow of electrons in momentum space can leads to a spin current. Through the theoretical model based on the semiclassical spin transport theory. We found that the spin current in the helical surface band is expressed as
where is the
th Fourier coefficient of the topological surface band, a is the lattice constant
is the Bloch frequency and
is the derivative of Bessel function of the first kind. Hence, the even-order harmonics along the pump polarization stem from the spin current in the helical surface states. Whereas the perpendicular components of the HHG should attribute to anomalous transverse current due to nonvanishing Berry curvature, as shown in the second term of EquationEq. (1)
(1)
(1) . The Berry curvature of TSS can be written as
where is the out-of-plane spin polarization,
. We found that the perpendicular components of even-order harmonics originate from the out-of-plane spin polarization related to the hexagonal wrapping effect [Citation40,Citation41].
C. P. Schmid et al. [Citation101] demonstrate a scheme to separate the HHG from bulk and surface. By tuning the frequency of few-cycle pump pulse, ranging from 25–42 THz, they observed HHG with distinct spectra signature, as shown in . For pump frequency larger than 33 THz, harmonic orders spaced by twice the driving frequency is clearly shown, that is odd-order harmonics (). Whereas for pump frequency below the absorption edge, e.g. 28 and 25 THz, even-order and odd-order harmonics co-occurrences in a same HH spectrum, with each harmonic peak spaced by a single driven frequency (). In the latter case with smaller photon energy, pump field is not sufficient for resonant interband transitions between bulk bands, therefore, field-driven acceleration of pre-existed Dirac electrons in TSS dominate the nonlinear dynamics. The emitted high-harmonics is produced from lightwave-driven scenario in TSS.
Figure 7. HHG from 3D TI Bi2Te3. (a) Schematic of the experimental layout. High-harmonic emission were produced from TI surface driven by s-polarized multi-THz field. (b) Typical high-harmonic spectra recorded from varying pump frequency ranging from 25–42 THz. (c),(d) Show the CEP dependence of the high-harmonic spectra from TSS for pump frequency of 25 THz. (e),(f) Show the CEP dependence of the high-harmonic spectra from bulk for pump frequency of 42 THz. Reproduced from [Citation101].
![Figure 7. HHG from 3D TI Bi2Te3. (a) Schematic of the experimental layout. High-harmonic emission were produced from TI surface driven by s-polarized multi-THz field. (b) Typical high-harmonic spectra recorded from varying pump frequency ranging from 25–42 THz. (c),(d) Show the CEP dependence of the high-harmonic spectra from TSS for pump frequency of 25 THz. (e),(f) Show the CEP dependence of the high-harmonic spectra from bulk for pump frequency of 42 THz. Reproduced from [Citation101].](/cms/asset/032efe7e-532c-46a3-b0a4-41c4b704d873/tapx_a_2013134_f0007_oc.jpg)
To understanding experimental observations, they calculated the HHG through solving both the semiclassical equation of motion and time-dependent Schrödinger equation, which can simulate the quantum mechanical wave packet dynamics in TSS. Intriguingly, they show that high-harmonic peaks emitted from bulk and TSS changes differently as a function of carrier-envelope phase (CEP) of the driving pulse (). Clearly, the strong dependence of HHG on CEP appears when the driven frequency is below the bulk band gap, as shown in –(d). However, when the driven frequency switched to 42 THz, the CEP dependence of the harmonic spectra is disappeared (–(f)).
Furthermore, they shown that the polarization states of odd- and even-order harmonics are evolved differently. The anomalous polarization change of even-order harmonics are attribute to the accumulate geometry phase of the Dirac fermions accelerate by the pump field along the Γ-K direction. The nonvanishing Berry curvature in TSS can bending the electron trajectory, thus lead to the emergence of perpendicular polarization component in HHG.
D. Baykusheva et al. [Citation102,Citation103] have investigated the HHG from the paradigmatic 3D TIs Bi2Se3 theoretically and experimentally. They found that both the harmonic yields from TSS and bulk states are sensitively dependent on the ellipticity of the laser field, however, their dependencies are divergent. To be specific, harmonics from TSS display an anomalous pump ellipticity behavior, which can be enhanced at high elliptical and circular polarized mid-IR fields, as shown in ). Whereas harmonics from bulk states behave similar as that from gases media, the harmonic yield drops monotonically (). The distinguishing feature predicted here is resultant from combination of the complex transition dipole elements and nonvanishing Berry connections.
Figure 8. Ellipticity dependence of HHG. (a) Dependence of high-harmonic yields on driving pulse ellipticity recorded from thin Bi2Se3 films. (b) Simulation results of HHG ellipticity dependence that originate from surface states (‘S’) and bulk states (‘B’). (c) Simulated HHG spectra produced by left-circularly polarized (LCP) laser pulses, harmonic orders (6 n + 1) and (3 n + 1) (red solid line) are corotating while (6 n-1) and (3 n-1) (blue solid line) are counterrotating with respective to pump laser helicity. Reproduced from [Citation103].
![Figure 8. Ellipticity dependence of HHG. (a) Dependence of high-harmonic yields on driving pulse ellipticity recorded from thin Bi2Se3 films. (b) Simulation results of HHG ellipticity dependence that originate from surface states (‘S’) and bulk states (‘B’). (c) Simulated HHG spectra produced by left-circularly polarized (LCP) laser pulses, harmonic orders (6 n + 1) and (3 n + 1) (red solid line) are corotating while (6 n-1) and (3 n-1) (blue solid line) are counterrotating with respective to pump laser helicity. Reproduced from [Citation103].](/cms/asset/bae868ee-a1f4-4b88-866c-d5c8f1e0061f/tapx_a_2013134_f0008_oc.jpg)
Besides, they show that the emitted harmonics driven by circularly polarized laser field should be restricted by the selection rules, because of the threefold rotational symmetry of crystalline Bi2Se3. For HHG from inversion symmetric bulk, permitted harmonic frequency is given by (). Whereas for HHG from the surface the requirement reads
, in which even-order harmonics are allowed due to breaking of inversion symmetry at surface ().
5. Conclusion and outlook
The unconventional surface electron behaviors are directly related to the topological order of the bulk electronic states. The entanglement between the massless surface electrons and massive bulk carriers may overwhelm the dynamics of the Dirac fermions. Therefore, the capability to selectively excite and manipulate the ultrafast electron transport in quasi-relativistic surface bands is in urgent demand. Coherent laser light provides an effective tool to investigate the unique topological properties in noncontact and widely tunable ways. The above observations show various avenues for optical controlling and switching the topological surface transport, ranging from light helicity excitation to lightwave-driven regime. Especially, by tuning the pump photon energy below the bulk band gap, the intraband motion in Dirac band and the interband transition across the Dirac point can selectively controlled, which makes the ultrafast dynamics of Dirac fermions dominate the surface transport in nonequilibrium. Out-of-equilibrium, the asymmetric redistribution or depopulation of the electrons in Dirac bands can produce controllable photocurrent and exhibit extremely nonlinearity.
Further increase the field strength, the helical Dirac fermions can be driven in the so-called light-field-driven (or lightwave-driven) regime, in which the electrons are excited and accelerated within a few fractions of an optical-cycle. Recent studies have shown great potential of light-field-driven ultrafast dynamics in 3D TIs surface. The ability to steer electrons on sub-cycle timescale in TSS enable the exploration of the build-up of inertia-free Dirac currents. Moreover, the ultrafast flow of Dirac electrons should lead to a net spin-polarized current, owing to spin–momentum locking. The advent of time-resolved photoemission and high-harmonic spectroscopy give an insight into the subcycle dynamics of Dirac fermions. The rapid advances in light-field-driven electron dynamics paves the way to generate fully controllable spin-polarized Dirac currents and intrigue the further understanding of strong-field physics in materials with nontrivial topological order. These studies hold promise for the construct the dissipationless TI-based spintronic devices that can be manipulated in optical frequency.
Acknowledgments
We would like to thank our collaborators sincerely for contributing to the related works presented in this review.
Disclosure statement
No potential conflict of interest was reported by the author(s).
Additional information
Funding
References
- Fu L, Kane CL. Topological insulators with inversion symmetry. Phys Rev B. 2007;76:045302.
- Fu L, Kane CL, Mele EJ. Topological insulators in three dimensions. Phys Rev Lett. 2007;98:106803.
- Hsieh D, Qian D, Wray L, et al. A topological Dirac insulator in a quantum spin Hall phase. Nature. 2008;452:970–23.
- Chen YL, Analytis JG, Chu J-H, et al. Experimental realization of a three-dimensional topological insulator, Bi2Te3. Science. 2009;325:178–181.
- Hsieh D, Xia Y, Qian D, et al. A tunable topological insulator in the spin helical Dirac transport regime. Nature. 2009;460:1101–1105.
- Hsieh D, Xia Y, Qian D, et al. Observation of time-reversal-protected single-Dirac-cone topological-insulator states in Bi2Te3 and Sb2Te3. Phys Rev Lett. 2009;103:146401.
- Hsieh D, Xia Y, Wray L, et al. Observation of unconventional quantum spin textures in topological insulators. Science. 2009;323:919–922.
- Xia Y, Qian D, Hsieh D, et al. Observation of a large-gap topological-insulator class with a single Dirac cone on the surface. Nat Phys. 2009;5:398–402.
- Zhang H, Liu C-X, Qi X-L, et al. Topological insulators in Bi2Se3, Bi2Te3 and Sb2Te3 with a single Dirac cone on the surface. Nat Phys. 2009;5:438–442.
- Chen YL, Chu J-H, Analytis JG, et al. Massive Dirac fermion on the surface of a magnetically doped topological insulator. Science. 2010;329:659–662.
- Hasan MZ, Kane CL. Colloquium: topological insulators. Rev Mod Phys. 2010;82:3045–3067.
- Qi X-L, Zhang S-C. Topological insulators and superconductors. Rev Mod Phys. 2011;83:1057–1110.
- Peres NMR. Colloquium: the transport properties of graphene: an introduction. Rev Mod Phys. 2010;82:2673–2700.
- Park SR, Han J, Kim C, et al. Chiral orbital-angular momentum in the surface states of Bi2Se3. Phys Rev Lett. 2012;108:046805.
- Cao Y, Waugh JA, Zhang XW, et al. Mapping the orbital wavefunction of the surface states in three-dimensional topological insulators. Nat Phys. 2013;9:499–504.
- Zhang H, Liu C-X, Zhang S-C. Spin-orbital texture in topological insulators. Phys Rev Lett. 2013;111:066801.
- Xie Z, He S, Chen C, et al. Orbital-selective spin texture and its manipulation in a topological insulator. Nat Commun. 2014;5:3382.
- Roushan P, Seo J, Parker CV, et al. Topological surface states protected from backscattering by chiral spin texture. Nature. 2009;460:1106–1109.
- Edelstein VM. Spin polarization of conduction electrons induced by electric current in two-dimensional asymmetric electron systems. Solid State Commun. 1990;73:233–235.
- Pesin D, MacDonald AH. Spintronics and pseudospintronics in graphene and topological insulators. Nat Mater. 2012;11:409–416.
- Soumyanarayanan A, Reyren N, Fert A, et al. Emergent phenomena induced by spin–orbit coupling at surfaces and interfaces. Nature. 2016;539:509–517.
- Li CH, van ‘T Erve OMJ, Robinson JT, et al. Electrical detection of charge-current-induced spin polarization due to spin-momentum locking in Bi2Se3. Nat Nanotechnol. 2014;9:218–224.
- Mellnik AR, Lee JS, Richardella A, et al. Spin-transfer torque generated by a topological insulator. Nature. 2014;511:449–451.
- Wang Y, Deorani P, Banerjee K, et al. Topological surface states originated spin-orbit torques in Bi2Se3. Phys Rev Lett. 2015;114:257202.
- Kondou K, Yoshimi R, Tsukazaki A, et al. Fermi-level-dependent charge-to-spin current conversion by Dirac surface states of topological insulators. Nat Phys. 2016;12:1027–1031.
- Qi X-L, Hughes TL, Zhang S-C. Topological field theory of time-reversal invariant insulators. Phys Rev B. 2008;78:195424.
- Fu L, Kane CL. Probing neutral Majorana fermion edge modes with charge transport. Phys Rev Lett. 2009;102:216403.
- Noh HJ, Koh H, Oh SJ, et al. Spin-orbit interaction effect in the electronic structure of Bi2Te3 observed by angle-resolved photoemission spectroscopy. EPL (Europhysics Letters). 2008;81:57006.
- Hor YS, Richardella A, Roushan P, et al. P-type Bi2Se3 for topological insulator and low-temperature thermoelectric applications. Phys Rev B. 2009;79:195208.
- Ren Z, Taskin AA, Sasaki S, et al. Optimizing Bi2-xSbxTe3-ySey solid solutions to approach the intrinsic topological insulator regime. Phys Rev B. 2011;84:165311.
- Arakane T, Sato T, Souma S, et al. Tunable Dirac cone in the topological insulator Bi2-xSbxTe3-ySey. Nat Commun. 2012;3:636.
- Kushwaha SK, Pletikosić I, Liang T, et al. Sn-doped Bi1.1Sb0.9Te2S bulk crystal topological insulator with excellent properties. Nat Commun. 2016;7:11456.
- Heremans JP, Cava RJ, Samarth N. Tetradymites as thermoelectrics and topological insulators. Nat Rev Mater. 2017;2:17049.
- McIver JW, Hsieh D, Steinberg H, et al. Control over topological insulator photocurrents with light polarization. Nat Nanotechnol. 2012;7:96–100.
- Kastl C, Karnetzky C, Karl H, et al. Ultrafast helicity control of surface currents in topological insulators with near-unity fidelity. Nat Commun. 2015;6:6617.
- Hsieh D, Mahmood F, McIver JW, et al. Selective probing of photoinduced charge and spin dynamics in the bulk and surface of a topological insulator. Phys Rev Lett. 2011;107:077401.
- Hosur P. Circular photogalvanic effect on topological insulator surfaces: berry-curvature-dependent response. Phys Rev B. 2011;83:035309.
- Ganichev SD, Ivchenko EL, Danilov SN, et al. Conversion of spin into directed electric current in quantum wells. Phys Rev Lett. 2001;86:4358–4361.
- Ganichev SD, Prettl W. Spin photocurrents in quantum wells. J Phys Condens Matter. 2003;15:R935–R983.
- Fu L. Hexagonal warping effects in the surface states of the topological insulator Bi2Te3. Phys Rev Lett. 2009;103:266801.
- Kuroda K, Arita M, Miyamoto K, et al. Hexagonally deformed Fermi surface of the 3D topological insulator Bi2Se3. Phys Rev Lett. 2010;105:076802.
- Goff JE, Schaich WL. Theory of the photon-drag effect in simple metals. Phys Rev B. 2000;61:10471–10477.
- Zhu ZH, Veenstra CN, Zhdanovich S, et al. Photoelectron spin-polarization control in the topological insulator Bi2Se3. Phys Rev Lett. 2014;112:076802.
- Sobota JA, Yang SL, Kemper AF, et al. Direct optical coupling to an unoccupied Dirac surface state in the topological insulator Bi2Se3. Phys Rev Lett. 2013;111:136802.
- Wang MC, Qiao S, Jiang Z, et al. Unraveling photoinduced spin dynamics in the topological insulator Bi2Se3. Phys Rev Lett. 2016;116:036601.
- Soifer H, Gauthier A, Kemper AF, et al. Band-resolved imaging of photocurrent in a topological insulator. Phys Rev Lett. 2019;122:167401.
- Olbrich P, Golub LE, Herrmann T, et al. Room-temperature high-frequency transport of Dirac fermions in epitaxially grown Sb2Te3- and Bi2Te3-based topological insulators. Phys Rev Lett. 2014;113:096601.
- Braun L, Mussler G, Hruban A, et al. Ultrafast photocurrents at the surface of the three-dimensional topological insulator Bi2Se3. Nat Commun. 2016;7:13259.
- Nastos F, Sipe JE. Optical rectification and shift currents in GaAs and GaP response: below and above the band gap. Phys Rev B. 2006;74:035201.
- Huang YQ, Song YX, Wang SM, et al. Spin injection and helicity control of surface spin photocurrent in a three dimensional topological insulator. Nat Commun. 2017;8:15401.
- Sun X, Adamo G, Eginligil M, et al. Topological insulator metamaterial with giant circular photogalvanic effect. Sci Adv. 2021;7:eabe5748.
- Steinberg H, Laloë JB, Fatemi V, et al. Electrically tunable surface-to-bulk coherent coupling in topological insulator thin films. Phys Rev B. 2011;84:233101.
- Saha K, Garate I. Theory of bulk-surface coupling in topological insulator films. Phys Rev B. 2014;90:245418.
- Mikhailov SA, Ziegler K. Nonlinear electromagnetic response of graphene: frequency multiplication and the self-consistent-field effects. J Phys Condens Matter. 2008;20:384204.
- Hendry E, Hale PJ, Moger J, et al. Coherent nonlinear optical response of graphene. Phys Rev Lett. 2010;105:097401.
- Ishikawa KL. Electronic response of graphene to an ultrashort intense terahertz radiation pulse. New J Phys. 2013;15:055021.
- Baudisch M, Marini A, Cox JD, et al. Ultrafast nonlinear optical response of Dirac fermions in graphene. Nat Commun. 2018;9:1018.
- Giorgianni F, Chiadroni E, Rovere A, et al. Strong nonlinear terahertz response induced by Dirac surface states in Bi2Se3 topological insulator. Nat Commun. 2016;7:11421.
- Brahlek M, Bansal N, Koirala N, et al. Topological-metal to band-insulator transition in (Bi1−xInx)2Se3 thin films. Phys Rev Lett. 2012;109:186403.
- Wu L, Brahlek M, Valdés Aguilar R, et al. A sudden collapse in the transport lifetime across the topological phase transition in (Bi1−xInx)2Se3. Nat Phys. 2013;9:410–414.
- Sim S, Brahlek M, Koirala N, et al. Ultrafast terahertz dynamics of hot Dirac-electron surface scattering in the topological insulator Bi2Se3. Phys Rev B. 2014;89:165137.
- Valdés Aguilar R, Qi J, Brahlek M, et al. Time-resolved terahertz dynamics in thin films of the topological insulator Bi2Se3. Appl Phys Lett. 2015;106:011901.
- Luo L, Yang X, Liu X, et al. Ultrafast manipulation of topologically enhanced surface transport driven by mid-infrared and terahertz pulses in Bi2Se3. Nat Commun. 2019;10:607.
- Yang X, Luo L, Vaswani C, et al. Light control of surface–bulk coupling by terahertz vibrational coherence in a topological insulator. Npj Quantum Mater. 2020;5:13.
- Faisal FHM, Kamiński JZ. Floquet-Bloch theory of high-harmonic generation in periodic structures. Phys Rev A. 1997;56:748–762.
- Wang YH, Steinberg H, Jarillo-Herrero P, et al. Observation of Floquet-Bloch states on the surface of a topological insulator. Science. 2013;342:453–457.
- Sobota JA, He Y, Shen Z-X. Angle-resolved photoemission studies of quantum materials. Rev Mod Phys. 2021;93:025006.
- Xu H, Zhou J, Li J. Light-induced quantum anomalous Hall effect on the 2D surfaces of 3D topological insulators. Adv Sci. 2021;8:2101508.
- Haldane FDM. Model for a quantum Hall effect without landau levels: condensed-matter realization of the “parity anomaly”. Phys Rev Lett. 1988;61:2015–2018.
- McIver JW, Schulte B, Stein FU, et al. Light-induced anomalous Hall effect in graphene. Nat Phys. 2020;16:38–41.
- Mahmood F, Chan C-K, Alpichshev Z, et al. Selective scattering between Floquet–Bloch and Volkov states in a topological insulator. Nat Phys. 2016;12:306–310.
- Iyer V, Chen YP, Xu X. Ultrafast surface state spin-carrier dynamics in the topological insulator Bi2Te2Se. Phys Rev Lett. 2018;121:026807.
- Kuroda K, Reimann J, Güdde J, et al. Generation of transient photocurrents in the topological surface state of Sb2Te3 by direct optical excitation with midinfrared pulses. Phys Rev Lett. 2016;116:076801.
- Kuroda K, Reimann J, Kokh KA, et al. Ultrafast energy- and momentum-resolved surface Dirac photocurrents in the topological insulator Sb2Te3. Phys Rev B. 2017;95:081103.
- Higuchi T, Heide C, Ullmann K, et al. Light-field-driven currents in graphene. Nature. 2017;550:224–228.
- Heide C, Higuchi T, Weber HB, et al. Coherent electron trajectory control in graphene. Phys Rev Lett. 2018;121:207401.
- Reimann J, Schlauderer S, Schmid CP, et al. Subcycle observation of lightwave-driven Dirac currents in a topological surface band. Nature. 2018;562:396–400.
- Di Pietro P, Adhlakha N, Piccirilli F, et al. Terahertz tuning of Dirac plasmons in Bi2Se3 topological insulator. Phys Rev Lett. 2020;124:226403.
- Ghimire S, DiChiara AD, Sistrunk E, et al. Observation of high-order harmonic generation in a bulk crystal. Nat Phys. 2011;7:138–141.
- Schubert O, Hohenleutner M, Langer F, et al. Sub-cycle control of terahertz high-harmonic generation by dynamical Bloch oscillations. Nat Photon. 2014;8:119–123.
- Vampa G, McDonald CR, Orlando G, et al. Theoretical analysis of high-harmonic generation in solids. Phys Rev Lett. 2014;113:073901.
- Xu Y, Miotkowski I, Liu C, et al. Observation of topological surface state quantum Hall effect in an intrinsic three-dimensional topological insulator. Nat Phys. 2014;10:956–963.
- Vampa G, Hammond TJ, Thiré N, et al. Linking high harmonics from gases and solids. Nature. 2015;522:462–464.
- Liu H, Li Y, You YS, et al. High-harmonic generation from an atomically thin semiconductor. Nat Phys. 2017;13:262–265.
- Langer F, Hohenleutner M, Huttner U, et al. Symmetry-controlled temporal structure of high-harmonic carrier fields from a bulk crystal. Nat Photon. 2017;11:227–231.
- Ghimire S, Reis DA. High-harmonic generation from solids. Nat Phys. 2019;15:10–16.
- Jiang S, Chen J, Wei H, et al. Role of the transition dipole amplitude and phase on the generation of odd and even high-order harmonics in crystals. Phys Rev Lett. 2018;120:253201.
- Jiang S, Wei H, Chen J, et al. Effect of transition dipole phase on high-order-harmonic generation in solid materials. Phys Rev A. 2017;96:053850.
- Jiang SC, Gholam-Mirzaei S, Crites E, et al. Crystal symmetry and polarization of high-order harmonics in ZnO. J Phys B. 2019;52:225601.
- Qian C, Yu C, Jiang S, et al. The role of shift vector in high harmonic generation from non-centrosymmetric topological insulators under strong laser fields. arXiv Preprint. 2021; arXiv:2106.14263.
- Jiang S, Yu C, Chen J, et al. Smooth periodic gauge satisfying crystal symmetry and periodicity to study high-harmonic generation in solids. Phys Rev B. 2020;102:155201.
- Yoshikawa N, Tamaya T, Tanaka K. High-harmonic generation in graphene enhanced by elliptically polarized light excitation. Science. 2017;356:736–738.
- Hafez HA, Kovalev S, Deinert J-C, et al. Extremely efficient terahertz high-harmonic generation in graphene by hot Dirac fermions. Nature. 2018;561:507–511.
- Avetissian HK, Avetissian AK, Avchyan BR, et al. Multiphoton excitation and high-harmonics generation in topological insulator. J Phys Condens Matter. 2018;30:185302.
- Jia L, Zhang Z, Yang DZ, et al. High harmonic generation in magnetically-doped topological insulators. Phys Rev B. 2019;100:125144.
- Bai Y, Fei F, Wang S, et al. High-harmonic generation from topological surface states. Nat Phys. 2021;17:311–315.
- Zhang S, Pi L, Wang R, et al. Anomalous quantization trajectory and parity anomaly in Co cluster decorated BiSbTeSe2 nanodevices. Nat Commun. 2017;8:977.
- Bianchi M, Guan D, Bao S, et al. Coexistence of the topological state and a two-dimensional electron gas on the surface of Bi2Se3. Nat Commun. 2010;1:128.
- King PDC, Hatch RC, Bianchi M, et al. Large tunable Rashba spin splitting of a two-dimensional electron gas in Bi2Se3. Phys Rev Lett. 2011;107:096802.
- Bahramy MS, King PDC, de La Torre A, et al. Emergent quantum confinement at topological insulator surfaces. Nat Commun. 2012;3:1159.
- Schmid CP, Weigl L, Grössing P, et al. Tunable non-integer high-harmonic generation in a topological insulator. Nature. 2021;593:385–390.
- Baykusheva D, Chacón A, Kim D, et al. Strong-field physics in three-dimensional topological insulators. Phys Rev A. 2021;103:023101.
- Baykusheva D, Chacón A, Lu J, et al. All-optical probe of three-dimensional topological insulators based on high-harmonic generation by circularly polarized laser fields. Nano Lett. 2021;21:8970–8978.