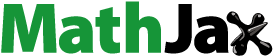
ABSTRACT
Quantum dots based on two-dimensional materials (2D-QDs) have received significant attention due to their exceptional physical, chemical, and biological properties. They have shown unprecedented performance and efficiency in many fields including electronics, spintronics, energy, water treatment, sensors, and biological applications. This article provides a critical review on the recent progress of 2D-DQs, their synthesis approaches, categories, properties, and applications. The review introduces various types of 2D-QDs, such as graphene, hBN, silicene, phosphorene, transition metal dichalcogenides, and MXenes that show a wide range of properties applicable for different fields. We describe in detail the electronic, magnetic, optical, catalytic, and biological properties of 2D-QDs and relate them to the suitable applications. Future directions for the research in 2D-QDs are given based on the novel properties provided by the newly discovered 2D materials and their heterostructures.
Graphical Abstract
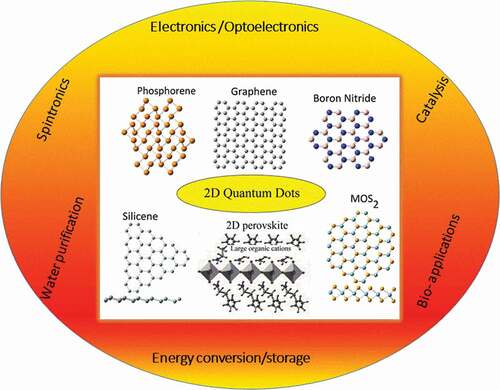
1. Introduction
Two-dimensional (2D) materials are ultrathin sheets from atomically thick single layer or few layers. They are considered as innovative materials due to their exceptional properties including excellent mechanical properties [Citation1-3], high thermal and electrical conductivity [Citation4–9], high carrier mobility [Citation10,Citation11], tunable electronic and optical properties [Citation12,Citation13], and high specific surface area [Citation14–16]. Graphene recorded the highest Young’s modulus of 1 TPa and an intrinsic strength of 130 GPa, which are significantly higher than the corresponding values in steel, namely, modulus of ~210 GPa and a strength of ~2600 MPa; thus, graphene is considered to be the strongest material ever measured [Citation1,Citation17]. Other 2D materials show enhanced mechanical properties, such as hBN/MoS2 with Young’s modulus of 865/270 GPa and intrinsic strength of 70.5/22 GPa [Citation18,Citation19]. In view of their impressive mechanical properties, 2D materials are promising candidates for flexible electronics and electromechanical devices [Citation20]. hBN exhibits an in-plane thermal conductivity as high as 585 W/mK at room temperature that is much higher than its bulk counterpart [Citation21]. This exceptional thermal conductivity, in addition to electrical insulation, makes hBN ideal candidate for next-generation thermal management applications [Citation22,Citation23]. High carrier mobility is another important property crucial for semiconductor devices to minimize power consumption and increase switching speed. Graphene has an extremely high carrier mobility (~2 × 105 cm2 V–1 s–1); however, its zero band gap hinders its application in logic gates due to the small current on/off ratio [Citation10]. Fortunately, the family of 2D materials can provide other candidates applicable for this job such as phosphorene (single layer of phosphorus) with mobility up to 2.6 × 104 cm2 V−1 s−1 and a moderate band gap of 1.51 eV [Citation24]. Decreasing the thickness of 2D materials significantly improves their optical transparency. Graphene absorbs only ~2.3% of the incident light, which means that graphene is transparent to more than 97% of the incident light. This excellent optical transparency opens the way toward graphene and similar 2D materials for high performance transparent optoelectronic devices [Citation25]. However, for other optoelectronic applications such as photodetectors, graphene and transparent 2D materials are not proper choices due to the low absorption coefficient. Therefore, other 2D materials with high absorption coefficient such as transition metal dichalcogenides (TMDCs), GaS, and 2D heterostructures have been used as efficient photodetectors [Citation26,Citation27]. The optical properties of 2D materials can be precisely controlled by applying external effects such as electric field, strain, and magnetic field [Citation28]. The refractive index of 2D materials can be tuned by applying electric field due to the change of carrier density. This effect involves alternation of the real part of the refractive index and modulation of the phase of the incident light, and therefore can be used in phase modulators [Citation29], optical switches [Citation30], and coherent optical communication [Citation31]. The large surface area, high surface-to-volume ratio, and the high interactivity of 2D materials widen their application spectrum to include supercapacitors, catalysts, energy storage, and sensors. For example, photoelectrochemical (PEC) biosensing that depends on the electron–hole generation by the light source and the subsequent changes due to the interaction of the target analytes with the recognition molecules is a powerful analytical technique for biomedical applications and environmental monitoring [Citation32]. The photoactive materials of PEC should have high surface area to absorb the maximum amount of incident light, strong absorption capacity, suitable band gap, and enhanced charge separation and transfer to ensure high photo-electricity conversion efficiency. These requirements are achieved in various 2D materials, such as graphitic carbon nitride (g-C3N4), TMDCs, and MXenes [Citation33].
Reducing the lateral size of the 2D materials below 20 nm creates 2D quantum dots (2D-QDs) with discrete energy levels due to the strong quantum confinement [Citation34]. 2D-QDs have almost all the fascinating physical and chemical properties of 2D materials in addition to the capability to precisely tune them and even introduce novel properties using different factors such as size, shape, edge termination, electric field, and chemical functionalization [Citation35–40]. 2D-QDs with quantum confinement in all three dimensions have size-dependent electronic and optical properties, thus a widely tunable photoluminescence has been observed in various 2D-QDs, such as graphene, MoS2, and hBN-QDs [Citation41–43]. They are also promising candidates for polymer reinforcement. For instance, the infusing of graphene QDs (GQDs) into various polymers greatly boosts their mechanical properties with considerable increase in the toughness, tensile strength, and Young’s modulus [Citation44,Citation45]. They have many more characteristics, such as easy functionalization, dissolvability in different solvents, high photostability, and biocompatibility [Citation46–50]. These fascinating properties make 2D-QDs promising applicants in various fields including electronics [Citation51,Citation52], optoelectronics [Citation53,Citation54], sensing [Citation55,Citation56], energy storage [Citation57,Citation58], quantum computation [Citation59,Citation60], and spintronics [Citation61,Citation62]. Here, we provide a comprehensive review of the recent progress of 2D-QDs. Their physical, chemical, and biological properties are introduced and related to different applications. Prospective research on 2D-QDs is given based on the recently discovered 2D materials and their hybrid heterostructures.
2. Synthesis methods
Synthesis of 2D-QDs has been significantly improved in recent years with controllable length, width, and edge type [Citation63,Citation64]. Two main approaches are used for the synthesis of 2D-QDs, namely top-down and bottom-up approaches. Bottom-up approach shows enhanced capabilities to synthesis 2D-QDs with atomic precision. On the other hand, attached chemical groups, dopants, or defects introduced during the top-down approach are useful for some applications, such as sensors and catalysts; therefore, the choice of the synthetic route is determined by the target application.
2.1. Top-down approach
Top-down methods prepare 2D-QDs via the cleavage of their bulk 2D materials using different approaches such as hydrothermal/solvothermal [Citation65,Citation66], thermal ablation [Citation67], sonication [Citation68], and intercalation [Citation69]. shows the graphical representation of selected approaches used for synthesis of MXenes QDs (MQDs) which in general can be used for the fabrication of other 2D-QDs. As shown in , the hydrothermal production of MQDs achieved in two steps: (a) the conversion of Ti3AlC2 Max to Ti3C2Tx MXenes by etching using hydrofuoric acid (HF) (b) transformation of Ti3C2Tx MXene into MQDs by hydrothermal treatment at 100 C for 6 h [Citation70]. To avoid the direct use of the hazardous HF in the etching process, Neupane et al. conducted the reaction LiF and HCl at ambient temperature that eventually resulted in HF. The MQDs are then obtained through the chemical solution method at room temperature following a magnetic stirring process for 24 h to etch Al from Ti3AlC2 powder [Citation71]. The intercalation technique shows high capability to fabricate MQDs with high-yield (60%) and well-defined fluorescent via intercalation of tetramethylammonium hydroxide (TMAOH) between the sheets of Ti3C2Tx at vigorous conditions [Citation72]. The extreme conditions (high temperature, harsh chemicals, etc.) involved in the conventional top-down synthesis of 2D-QDs may lead to a significant degradation of the prepared samples due to oxidization. Recently, H. Alijani, et al. developed a new method to fabricate MQDs based on acoustomicrofluidic approach with negligible degradation [Citation73]. As shown in , the considerable surface acceleration from the high-frequency electromechanical vibrations produced on piezoelectric substrate nebulizes the layered Ti3C2Tz suspension into single layers and quantum dots.
Figure 1. (a) Hydrothermal, (b) intercalation, and (c) acoustomicrofluidic synthesis approaches. The figures were reproduced with permissions from: (a) ref. [Citation70], (b) ref. [Citation72], and (c) ref. [Citation73].
![Figure 1. (a) Hydrothermal, (b) intercalation, and (c) acoustomicrofluidic synthesis approaches. The figures were reproduced with permissions from: (a) ref. [Citation70], (b) ref. [Citation72], and (c) ref. [Citation73].](/cms/asset/9fda4e3c-8db5-45e3-9e79-c3ed11456054/tapx_a_2048966_f0001_oc.jpg)
Figure 2. Selected bottom-up methods for synthesis of hBN-QDs (a), MoS2-QDs (b), graphene quantum dots (c), and Mo2C-QDs (d). The figures are reproduced with permissions from refs. [Citation79–81,Citation84]respectively.
![Figure 2. Selected bottom-up methods for synthesis of hBN-QDs (a), MoS2-QDs (b), graphene quantum dots (c), and Mo2C-QDs (d). The figures are reproduced with permissions from refs. [Citation79–81,Citation84]respectively.](/cms/asset/3eeabe88-0d59-41cf-a41b-d3eac5efdce9/tapx_a_2048966_f0002_oc.jpg)
2.2. Bottom-up approach
Bottom-up synthetic approach is based on the concept of molecular self-assembly in which the atomic or molecular precursors assemble to form the desired 2D-QDs with well-defined structure [Citation74,Citation75]. This approach includes different preparation methods, such as hydrothermal synthesis [Citation76], chemical path deposition [Citation77], and electron beam irradiation [Citation78]. Chen et al. reported a facile bottom-up synthesis of hBN-QDs via hydrothermal reaction between boric acid and melamine [Citation79], see ). The hydrothermal method is also employed to synthesize MoS2-QDs () using Molybdenum trioxide (MoO3) powder, as molybdenum source, and l-cysteine as sulfur source [Citation80]. Electron beam irradiation method was used to synthesize graphene QDs (GQDs) where 1,3,6-trinitropyrene and hydrazine hydrate were used as molecular precursors to prepare crystalline GQDs as schematically presented in ) [Citation81]. MQDs have also been synthesized using different bottom-up methods [Citation82,Citation83]. For instance, as shown in ), the molybdenum carbide quantum dots (Mo2C-QDs)/carbon nanosheet composites were synthesized via molten synthesis strategy using Molybdenum acetylacetonate, sucrose, and NaCl as the molecular precursors [Citation84].
3. Categories of 2D-QDs
The family of 2D materials has been expanded to include hundreds of materials with an unprecedentedly wide spectrum of mechanical, electronic, optical, and chemical properties [Citation85–87]. 2D materials can be categorized into single, double, and multi-element 2D materials according to the number of elements they contain [Citation88,Citation89]. The single-elemental 2D materials include planar and buckled 2D materials consisting of only one building atom such as graphene [Citation90], graphyne [Citation91], borophenes [Citation92], silicene [Citation93], stanene [Citation94], phosphorene [Citation95], and antimonene [Citation96]. In this group, 2D materials have planar structure with sp2/sp-sp2 hybridization as in graphene/graphyne or buckled structure with sp2-sp3/sp3 hybridization as in silicene/antimonene; see –). The double-element 2D materials contain hBN [Citation97], boron phosphide [Citation98], graphitic carbon nitride (g-C6N7) [Citation99], bismuth nitride [Citation100], TMDs [Citation101], and metal oxides [Citation102].
Figure 3. Structures of different 2D-QDs: (a) graphene, (b) silicene, (c) antimonene, (d) g-C3N4, (e) hBN, (f) MoS2, (g) Si2BN, and (h) perovskite. The 2D perovskite is reproduced with permission from [Citation107].
![Figure 3. Structures of different 2D-QDs: (a) graphene, (b) silicene, (c) antimonene, (d) g-C3N4, (e) hBN, (f) MoS2, (g) Si2BN, and (h) perovskite. The 2D perovskite is reproduced with permission from [Citation107].](/cms/asset/e5aca09b-3578-4f17-849f-65f9e678ce35/tapx_a_2048966_f0003_oc.jpg)
Boron phosphide and hBN have the same planar structure with strong in-plane sigma bonds like graphene; see ). Graphitic carbon nitride also has a planar structure but with uniformly distributed nanopores where tri-s-triazine (C3N3) rings connect to each other by C-N bonds to form the triangular nanopores structure shown in ). The monolayer TMDCs (such as MoS2 in ) consist of three sublayers, and the central atomic layer is a transition metal which is sandwiched between two chalcogen layers. The third category is the group of 2D materials containing three or more elements, such as Si2BN [Citation103], MXenes [Citation104], transition metal oxyhalides [Citation105], and perovskites [Citation106]. Si2BN is a planar structure like graphene but consists of three elements, Si, B, and N, as shown in . Another example of this group is the 2D perovskites, which can be represented by the formula: (A’)2An-1BnX3n+1, where A’ is a large organic spacer, A is a small organic cation, B is a metal cation, n is the number of metal cation layers between the two layers of large organic cation. ) shows the simplest 2D perovskite that has n = 1 and the formula (A’)2BnX4 [Citation107]. In this article, we mention a few number of 2D materials as representative examples. Recently, Mounet et al. reported theoretically that 1825 3D materials have weak interlayer interactions and thus are potential candidates to be exfoliated into ultrathin 2D materials [Citation108]. An easy access to all possible 2D materials directly from the periodic table can be found in materials cloud [Citation109]. Of course, 2D-QDs from all these 2D materials have not been investigated yet; however, we introduce a huge family of 2D materials because they are, in principle, building blocks for the current and future 2D-QDs. Also, we categorized the 2D-QDs into three categories similar to 2D materials. It is worth noting that currently hundreds of 2D-QDs have been investigated both theoretically and experimentally, such as GQDs [Citation110], Silicene QDs (SiQDs) [Citation111], Phosphorene QDs (PQDs) [Citation112], antimonene QDs (SbQDs) [Citation113], g-C3N4-QDs [Citation114], hBN-QDs [Citation115], TMDCs-QDs [Citation116], MXenes-QDs [Citation117], and Perovskite-QDs [Citation118].
4. Properties
The exceptional physical and chemical properties of 2D-QDs originate from the ultra-high specific surface area, tunable surface chemistry, the quantum size effect, and the superior mechanical properties. Additionally, these advantages can be controlled precisely using different factors such as size, edge type, electric field, chemical functionalization, doping of heteroatoms, and heterostructure formation. Following, we discuss the main properties of 2D-QDs, namely electronic, optical, catalytic, and biological properties.
4.1. Electronic properties
A controllable energy gap is one of the main advantages that quantum dots can add to a material. For instance, graphene with all its unique properties cannot be used in semiconductor devices due to its zero band gap, while GQDs with size-dependent energy gap can solve this problem [Citation35,Citation119]. The energy gap can be increased by decreasing the size of the 2D material as a result of the quantum confinement effect [Citation120]. shows a qualitative representation of the increase in the energy gap by decreasing the size of the GQDs [Citation121]. As for the quantitative values of the energy gap, Eda et al. reported theoretically that the energy gap of a single aromatic ring is as high as ~7 eV and decreases by increasing the number of aromatic rings as presented in [Citation122].
Figure 4. (a) Qualitative representation of the effect of size on the energy gap of GQDs. (b) the DFT-based values of the energy gap calculated for hexagonal GQDs as a function of the number of aromatic rings. (c) Bisanthrene and its hexaradical Clar goblet, (d) the corresponding spin density difference distribution with red cubs represent spin-up and blue cubs for spin-down density. (e) Triangular 2D-QDs and its non Kekule structure, the red and blue spots represent the A and B suplattices. The spin density difference of triangular hydrogenated GQDs (f), double hydrogenated (g), and SiQDs with hydrogenated surface (h). Side view of the 2D chromium triiodide (CrI3, gray/purple balls represent Cr/ I atoms) with the arrows representing the Ising spin direction. The plots are reproduced with permission from ref. [Citation122] (a), [Citation123] (b), [Citation152] (c, d), [Citation162] (e), [Citation153] (f, g), [Citation62] (h), and [Citation165] (i).
![Figure 4. (a) Qualitative representation of the effect of size on the energy gap of GQDs. (b) the DFT-based values of the energy gap calculated for hexagonal GQDs as a function of the number of aromatic rings. (c) Bisanthrene and its hexaradical Clar goblet, (d) the corresponding spin density difference distribution with red cubs represent spin-up and blue cubs for spin-down density. (e) Triangular 2D-QDs and its non Kekule structure, the red and blue spots represent the A and B suplattices. The spin density difference of triangular hydrogenated GQDs (f), double hydrogenated (g), and SiQDs with hydrogenated surface (h). Side view of the 2D chromium triiodide (CrI3, gray/purple balls represent Cr/ I atoms) with the arrows representing the Ising spin direction. The plots are reproduced with permission from ref. [Citation122] (a), [Citation123] (b), [Citation152] (c, d), [Citation162] (e), [Citation153] (f, g), [Citation62] (h), and [Citation165] (i).](/cms/asset/5fee7b93-9582-4895-b102-00439c0316c6/tapx_a_2048966_f0004_oc.jpg)
The size of GQDs can be controlled experimentally by adjusting the preparationconditions, such as reaction time, temperature, pH level, and reactant concentration [Citation123–126]. In addition to the size effect, the energy gap is also controlled by shape, edge termination, and chemical functionalization. In , the effects of edge type, shape, and chemical functionalization on the energy gaps of different 2D-QDs are listed based on DFT calculations. GQD with hexagonal shape and armchair termination (AHEX-GQDs, similar to the one shown in ) has energy gap equals 3.68 eV while for triangular GQD with almost the same number of atoms the energy gap becomes 1.82 eV [Citation127]. The large energy gap in hexagonal-armchair GQD decreases to half its value by functionalizing the edges with O-atoms or moderately decreases to 3.01 eV by attaching four nitro groups to the edges [Citation128]. De Alwis et al. reported that edge functionalization of rectangular (see Rect in ) PQDs with different chemical groups (e.g. OH, SH, NH2, and OCN) can shift the HOMO and LUMO, which in turn change the energy gap [Citation129].
Table 1. The energy gaps of various 2D-QDs subject to the effects of edge, shape and chemical functionalization
Some applications require, in addition to a suitable energy gap, other properties such as hardness, interactivity, anticorrosion, thermal stability, and so on. In such cases, mixing the controllable electronic properties with other physical and chemical properties depending on the type of the material will significantly widen the application field of these materials. hBN-QDs have a large insulating energy gap ~6 eV in addition to the high anticorrosion property [Citation130,Citation131], Si2BN-QDs have a narrow semiconducting energy gap of 0.85 eV with high interactivity due to the active pi-electrons from Si atoms [Citation132,Citation133]. Phosphorene quantum dots have energy gap of 3.82 eV in addition to strong linear dichroism due to their buckled structure [Citation134,Citation135]. Quantum dots from TMDCs are characterized by strong spin-orbit coupling in addition to semiconducting properties with energy gaps ranging from 2 to 3.2 eV [Citation136,Citation137].
Moreover, Van der Waals heterostructures formed by vertical or lateral stacking of different 2D materials create materials not only with the combined properties of the constituting materials but also with novel properties [Citation138–140]. Heterostructures based on 2D-QDs have shown exciting electronic properties. For instance, donor-acceptor heterojunction from the same material (PQDs) suitable for solar cells [Citation141] and efficient modulation of electron transfer by forming 0D/2D Van der Waals heterostructure from GQDs/MoS2 monolayer [Citation142]. PQDs significantly improve the light absorption of MoS2 monolayer in the mixed-dimensional heterostructure from PQDs-MoS2 [Citation143]. Recently, Zhou et al. constructed novel 0D/0D heterostructures from g-C3N4 quantum dots/anatase-TiO2/routile-TiO2 quantum dots through mixing of TiO2-P25 and g-C3N4 quantum dots followed by thermal treatment [Citation144]. These exceptional type-II/type-II heterojunctions boost the spatial charge separation and transfer of the photo-generated carriers. Additionally, their 0D nature provides them with abundant active sites for efficient photocatalytic reaction.
4.2. Magnetic properties
Graphene is characterized by a long spin relaxation time and diffusion length as a result of the small spin-orbit coupling between C-atoms in the planar structure. This property makes graphene a promising candidate in spintronics [Citation145]. However, graphene is a non-magnetic material due to the delocalized distribution of π-bonds on the periodic lattice [Citation146,Citation147], which is a serious drawback that hinders its applications in spintronic devices [Citation148]. For instance, a spin-valve based on graphene shows a very small magnetoresistance of 10% [Citation149]. Cutting graphene into nanoribbons or quantum dots with zigzag edges is an effective solution to control the magnetic state of graphene, which in turn significantly improves the valves magnetoresistance [Citation150,Citation151]. For example, the nonmagnetic nature of graphene transforms to antiferromagnetic localized states at the zigzag edges of bisanthrene, see ) [Citation152]. This is the smallest polycyclic aromatic hydrocarbon representing spin polarized nanoribbons from graphene [Citation152]. Additionally, triangular QDs from graphene, silicene, and/or any 2D material have frustrated edge atoms ()) with unpaired electrons on the zigzag edges. These electrons cannot form delocalized bonds with neighbor atoms and instead form half-filled molecular orbitals that create a ferromagnetic spin ordering [Citation153–156]. It is important to note that not all bipartite systems with zigzag termination have magnetic properties. For instance, hBN is a bipartite lattice; however, its electrons are hybridized to form only three sigma bonds with no unpaired pz-orbital electrons unlike graphene or silicene [Citation157,Citation158]. The net spin (S) due to the unpaired electrons can be obtained from Lieb’s theorem of bipartite lattices [Citation159] using the following simple equation: S = (NA-NB)/2, where NA and NB represent the number of atoms of A and B sub-lattices, respectively. Using this equation, the net spin of triangular graphene or silicene with 5-edge hexagons equals 2 with higher spin-up density on the edge atoms; see ). Ahin et al. [Citation153] indicated that double hydrogenation of the edge atoms of triangular-zigzag GQDs removes the spin states at the edges due to passivation of all edge electrons and induces frustrated surface atoms with half-filled molecular orbital and a net spin increased to S = 4 ()). On the other hand, Abdelsalam et al. reported that surface hydrogenation of triangular-zigzag SiQDs significantly increase the net spin to S = 7.5 with a perfect spin density difference distributing on edge atoms as shown in ) [Citation62]. Despite the multiple difficulties of synthesizing these materials due to their high chemical reactivity, however, the recent advance in the on-surface synthetic approach allows for the fabrication of triangular-zigzag GQDs with permanent high-spin [Citation160–162]. Recently, Liu et al. showed that triangular-zigzag GQDs can be linked together using various linkages (e.g. small GQDs, magnetic metal atoms, and molecules) to form 2D magnetic semiconductors with enhanced Curie temperature up to 472 K [Citation163].
The rich family of 2D materials also offers intrinsic 2D magnetic materials (regardless of the shape, edge termination, or any external effects) with magnetism originating from the half-field d-orbitals of the transition metal. Such as the observed ferromagnetism in Fe3GeTe2 [Citation164] and chromium triiodide (CrI3) [Citation165] at low temperature, while monolayer vanadium selenide (VSe2) shows strong ferromagnetic ordering at room temperature and higher [Citation166]. shows the graphical representation of the spin orientation in the 2D-CrI3. In addition to ferromagnetism, 2D magnetic materials include insulating antiferromagnets such as MnPS3 [Citation167] and materials with large magnetic anisotropy such as α-RuCl3 [Citation168]. These 2D magnetic layered materials represent an ideal platform to study and control magnetism in 2D limits [Citation169–172]. Quantum dots from these 2D magnets have not been investigated yet, according to the best of our knowledge, due to the very recent discovery of these 2D materials. Regarding the conventional QDs or the magnetic nanoparticles, it is known that Curie temperature drastically decreases by decreasing the size of the nanoparticles [Citation173,Citation174]. Also, decreasing the thickness of 2D magnetic materials decreases the Curie temperature [Citation175]. Thus, decreasing the size of 2D magnets to the quantum regime may decrease the Curie temperature in the resultant 2D-QDs. This may not occur and the Curie temperature increases similarly to the anomalous increase in Curie Temperature by decreasing the thickness of the 2D metallic 1 T-CrTe [Citation176]. Therefore, prospective works on magnetic 2D-QDs are crucial for both fundamental understanding of 2D magnets and also for building efficient 2D spintronics.
4.3. Optical properties
The optical properties of 2D-QDs are tunable and cover a broadband range of wavelengths starting from ultraviolet to infrared [Citation177–182] due to the size-dependent quantum confinement [Citation117,Citation183], edge type [Citation184,Citation185], chemical functionalization [Citation186,Citation187], and doping [Citation188,Citation189]. According to the quantum confinement effect, the energy gap of a quantum system is approximately proportional to 1/L where L is the size of the quantum dot. Then, changing the size of QDs changes the energy gap and consequently the optical properties. It has been reported that the absorption and photoluminance spectra of GQDs strongly depend on the size where the absorption and emission spectra are blue shifted by decreasing the size of the GQDs [Citation190,Citation191]. Regarding the edge type, the photoluminance (PL) of GQDs shows peculiar behavior when the average size becomes higher than 17 nm where the energy of the PL peak increases by increasing the average size [Citation190]. This behavior, which does not follow the quantum confinement effect, arises due to the change in the edge type from a mixed zigzag and armchair terminated edges to edges with mostly armchair terminations. The effect of edge type on the optical properties of SiQDs was also investigated theoretically using tight binding model [Citation192]. The calculations show that triangular SiQDs with zigzag termination have a reach and widely controllable infrared absorption peak originates due to transitions from or to edge states. This absorption peak disappears in triangular SiQDs with armchair termination due to the disappearance of the low energy edge states. With respect to chemical modification, Ding et al. managed to modulate the photoluminescence of hBN-QDs to include full -color emission (420–610 nm) by attaching amino ligands to the edges [Citation185]. As shown in a full-color photoluminescence emission and UV-Vis spectra can be achieved in hBN-QDs by attaching specific amine ligands in different solvents. Namely, blue hBN-QDs (B-QD) is obtained by attaching urea to N-methylpyrrolidone, green hBN-QDs (G-QD) by attaching p-phenylenediamine to sulfuric acid. Green-yellow, yellow, and red hBN-QDs (GY-, Y-, and R-QD) are obtained by passivation with thiourea ligand in acetone, p-phenylenediamine in dimethylformamide, and p-phenylenediamine in ethanol, respectively. Therefore, good thermal dissipation, high transparency, and full-color emission make hBN-QDs promising applicants for thermostable, transparent, and flexible displays.
Figure 5. (a) Illumination photographs of chemically functionalized hBN-QDs under 360 UV-irradiation. (b, c) Emission and absorption spectra of hBN-QDs. (d) Absorption spectra of MQDs with different concentrations. (e) Normalized absorbance intensity divided by the characteristic length of the cell (A/L) for varied MQDs concentrations. (f) MQDs photothermal heating curves at different power densities. The plots (a-c) and (d-f) are reproduced with permission from [Citation185] and [Citation199], respectively.
![Figure 5. (a) Illumination photographs of chemically functionalized hBN-QDs under 360 UV-irradiation. (b, c) Emission and absorption spectra of hBN-QDs. (d) Absorption spectra of MQDs with different concentrations. (e) Normalized absorbance intensity divided by the characteristic length of the cell (A/L) for varied MQDs concentrations. (f) MQDs photothermal heating curves at different power densities. The plots (a-c) and (d-f) are reproduced with permission from [Citation185] and [Citation199], respectively.](/cms/asset/9e6f119d-f416-45be-8e79-b8753bb163f5/tapx_a_2048966_f0005_oc.jpg)
In addition to these effects, plasmonic effect of metallic QDs can significantly enhance the absorption of light [Citation193]. In plasmonic effect, conduction electrons of the metallic QDs oscillate collectively when their frequency matches that of the incident electromagnetic wave causing strong absorption of light. Plasmons were observed in various 2D-QDs, such as GQDs [Citation194], MoS2-QDs [Citation195], and MQDs [Citation196]. Neupane, et al. reported highly enhanced photoluminescence in MQDs-WS2 heterojunction due to the plasmonic behavior of MQDs and the suspension state of WS2[Citation196]. Another interesting property found in MQDs is their high capability to absorb light and convert it to different forms of energy [Citation197,Citation198]. For instance, Yu et al. reported the synthesis of biocompatible (fluorine-free) Ti3C3-QDs with not only strong absorption in the near-infrared region and mass extinction coefficient equal to 52.8 Lg−1 cm−1, but also high photothermal conversion efficiency of up to 52.2% [Citation199]. The UV-Vis-IR spectra at different concentrations of MQDs in show strong and broadband absorption in the near-IR region. In addition to the quantum confinement effect, this enhanced absorption is suggested to be a result of the localized surface plasmon resonance improved by the Al oxoanions (Al(OH)4−) on the surface formed by the fluorine-free synthetic approach [Citation199,Citation200]. The calculated value of mass extinction coefficient, a tool to evaluate the light absorption efficiency, shown in is very high compared to other photothermal based on 2D materials [Citation201–203]. In addition to the strong absorption capability, MQDs show promising photothermal conversion efficiency, where the temperature of a solution of 10 ppm MQDs solution increases by 14.1°C after laser irradiation for 5 min as seen in , while irradiating pure water at the same condition produces negligible temperature increase.
4.4. Catalytic properties
The abundant active sites, in addition to the tunable physical properties previously discussed, make 2D-QDs promising candidates for efficient catalysts [Citation58,Citation204,Citation205]. Reducing the size of 2D sheets to QDs strongly enhances the effect of edges where the density of states is strongly enhanced at the zigzag edges that form high-density active sites [Citation206–209]. The unsaturated atoms formed at the edges of the nanodots are highly interactive. They can easily interact with surrounding atoms or molecules, such as the strong electrocatalysis observed at the edges of WS2- and VS2-QDs [Citation210–212]. The high surface area and defects also increase the active sites at the surface [Citation213,Citation214]. Moreover, doping, chemical functionalization, and heterostructure formation strongly boost the catalytic performance. For example, N-doped GQDs have superior electrocatalytic properties compared to their N-free counterparts [Citation215]. N-atoms are widely used in doping carbon-materials due to their comparable atomic size to C-atoms with significantly enhanced electronic and catalytic properties [Citation216,Citation217]. The electronegativity of the substitutional doped N-atom is higher than the neighbor C-atoms leading to electron transfer from C to N. Thus, the positively charged C-atoms will be the active site with high density of states around the Fermi level [Citation218]. This charge redistribution significantly improves the catalytic performance of graphene from being poor electrocatalyst with low electron transfer and poor electrochemical activity [Citation219,Citation220] to efficient electrocatalyst after doping [Citation221,Citation222]. Additionally, hBN-QDs show superior catalytic properties toward hydrogen evolution reaction (HER), with free energy change equal to 0.11 eV [Citation131] comparable to 0.09 eV for the benchmarking platinum catalyst [Citation223]. However, pristine hBN-QDs show low overall catalytic performance due to their wide insulating energy gap that decreases the electron transport and eventually affects the Tafel slope. This problem can be solved by doping or by attaching chemical groups to tailor the electronic properties and improve the electron transport [Citation131,Citation224]. Based on this idea, doping GQDs with heteroatoms (nitrogen, boron, sulfur, and phosphorus) can help construct highly efficient C-based metal-free catalysts [Citation225,Citation226]. With respect to TMDCs, doping enhances the electrocatalytic HER by decreasing the change in the free energy and introducing more active sites [Citation227,Citation228].
In , we present the catalytic performance of different 2D materials toward HER. It is observed from that doping decreases the HER free energy with the highest decrease in the N-S codoped graphene, ∆GH* = 0.23 eV, and thus the best HER catalytic performance [Citation229]. The polarization curves present an important role of doping in decreasing the value of the HER overpotential of graphene toward that of MoS2. The potential in the current density-potentials curves (polarization curves) represents not only the thermodynamic potential for HER plus the overpotential but also ohmic potential originated from the internal resistance of the system [Citation233,Citation234]. Interestingly, combining both the N-S doping and the defects in porous graphene introduces an additional improvement to the HER with ∆GH* = 0.12 eV [Citation235] which is lower than ∆GH* = 0.15 eV for the metal-based MoS2 catalysts. Another interesting idea that may introduce further decrease in the overpotential is the addition of the effects of quantum confinement and shape that appear in QDs to the effects of defects and doping. The volcano plot and the polarization curves in shows that metal-doped MoS2 sheets are exceptional HER electrocatalyst with extremely low overpotential down to 0.06 eV in Pt-doped MoS2 [Citation230]. Ding et al. reported that the role of Pt-dopants is to boost the activity of the neighbor S-atoms, where they found that the ∆GH* = 0.06 eV on the in-plane S-atoms, while ∆GH* = 0.1 eV on edge S-atoms [Citation230]. Since there are few studies on HER using 2D-QDs, we represent only in the effect of reducing size on the HER catalytic performance of N-doped MoS2. It is seen from the plot that reducing the size significantly enhances the catalytic properties, namely by lowering the overpotential from ∆GH* = 0.095 eV in N-MoS2 nanosheets to ∆GH* = 0.082 eV in N-MoS2 QDs [Citation231]. Moreover, chemically modified MXenes (e.g. Ti2C and V2C) have remarkable catalytic properties; Gao et al. show that oxygen-atoms at the surface provide active sites for HER with exceptional overpotential equals −0.04 eV [Citation232]. Therefore, chemical modification and decreasing the size of 2D sheets to the quantum regime may lead to the construction of efficient catalysis that can replace the highly expensive Pt-based catalysts.
Figure 6. (a) HER free energy diagram and (b) polarization curves of graphene before and after doping; reproduced with permission from [Citation229]. (c) The Volcano plot of metal-doped MoS2 and in (d) the corresponding polarization curves for selected dopants are shown, with permission from [Citation230]. (e) Comparison of the polarization curves of N-doped MoS2 quantum dots and nanosheets, reproduced with permission from [Citation231]. (f) DFT calculations of the Volcano plot of various 2D MXene with and without termination by O-atoms reproduced with permission from [Citation232].
![Figure 6. (a) HER free energy diagram and (b) polarization curves of graphene before and after doping; reproduced with permission from [Citation229]. (c) The Volcano plot of metal-doped MoS2 and in (d) the corresponding polarization curves for selected dopants are shown, with permission from [Citation230]. (e) Comparison of the polarization curves of N-doped MoS2 quantum dots and nanosheets, reproduced with permission from [Citation231]. (f) DFT calculations of the Volcano plot of various 2D MXene with and without termination by O-atoms reproduced with permission from [Citation232].](/cms/asset/6025f32c-ec36-4671-88de-9194040f989b/tapx_a_2048966_f0006_oc.jpg)
4.5. Biological properties
Due to its high biocompatibility, easy chemical functionalization, good solubility, degradability, small size, and the exceptional physicochemical properties, 2D-QDs are excellent candidates for biological applications. Tabish et al. reported excellent biological properties of GQDs such as exceptional photoluminance, high water solubility, excellent corrosion resistance, high photo/pH-stability, and good in vitro and in vivo biocompatibility [Citation236]. Other 2D-QDs such as hBN-, g-C3N4-, antimonene-, TMDC-, M-QDs exhibit remarkable biological properties [Citation237–241]. An important biological application is drug delivery, where we show its mechanism using GQDs in . In that respect, chemical functionalization, on the surface and/or the edges, is crucial to obtain an efficient 2D drug delivery system [Citation242]. Chemical groups or elements attached to the GQDs form the active sites through which different biomolecules, e.g. drugs and targeting legends, can be loaded onto the GQDs. Biomolecules can also be loaded on the surface by electrostatic bonding, π-π interactions, hydrogen bonding, which in addition to the active groups on the surface make GQDs ideal drug carriers. The active chemical groups on the surface can also improve the quantum yield and the photoluminescence. For instance, Qian et al. showed that functionalized GQDs have better bioimaging characteristics compared to pristine-graphene due to their high quantum yield [Citation243]. Iannazzo et al. indicated that GQDs could then be used as an efficient drug delivery system capable of delivering the therapeutic drug to cancer cells in selective way [Citation244]. Namely, the drug (e.g. doxorubicin) is adsorbed on the surface of GQDs through the π-π interactions and the tumor-targeting module (e.g. biotin that is able to recognize cancer biotin receptors) is covalently bound to the carboxylic group on the edge of GQDs. Additionally, the exceptional photoluminescence of functionalized GQDs allows for the precise tracing of the drug release. Alongside GQDs, MoS2-QDs have demonstrated great potential in drug delivery due to their excellent biocompatibility, tunable photoluminescence, and high cellular uptake [Citation245,Citation246]. Liu et al. demonstrated that MoS2 nanosheets functionalized with polyethylene glycol are promising multifunctional drug delivery systems due to their high capacity for drug loading and the strong near-infrared absorbance [Citation247].
Figure 7. Mechanism of drug delivery using GQDs, reproduced with permission from [Citation242] .
![Figure 7. Mechanism of drug delivery using GQDs, reproduced with permission from [Citation242] .](/cms/asset/2289df8d-b35e-4e5f-a0c5-ee5f5588a9a8/tapx_a_2048966_f0007_oc.jpg)
5. Applications
The unique physical, chemical, and biological properties of 2D-QDs make them attractive for a wide range of applications. In this work, we will discuss the potential applications of 2D-QDs in the following fields, electronics/optoelectronics, spintronics, energy, water treatment, sensors, and biological applications.
5.1. Electronics/optoelectronics
2D-QDs with tunable electronic properties found their applications in various electronic devices, such as transistors, light-emitting diodes (LEDs), and memory devices. 2D transistors with ultrahigh mobility, exceptional flexibility, and high environmental stability are expected to form the future of high-speed flexible electronics that could outperform organic semiconductors [Citation248]. Chen et al. reported the best-performing 2D-MoS2 transistors to date with an on/off ratio higher than 107, an intrinsic gain ~30, an intrinsic cut-off frequency of 42 GHz in addition to a maximum power gain of 50 GHz. As shown in , graphene single-electron transistor (SET) can be achieved by connecting GQDs to source and drain electrodes through two graphene constrictions with four side gates that tune the electronic properties [Citation35,Citation249,Citation250]. Therefore, 2D-QDs provide stable and conductive SET with one-by-one electron transfer at the molecular scale [Citation251].
Figure 8. (a) The scanning electron image of the constructed single electron transistor (SET) based on graphene. The dark areas in the image are the gaps in the photoresist mask that are created by removing graphene from these areas by plasma etching. The central GQD is connected to the contacts through narrow constraints (nanoribbons) and four side gates to control the electronic properties. (b) Schematic representation of SET based on GQD containing few numbers of atoms, reproduced with permission from [Citation250].
![Figure 8. (a) The scanning electron image of the constructed single electron transistor (SET) based on graphene. The dark areas in the image are the gaps in the photoresist mask that are created by removing graphene from these areas by plasma etching. The central GQD is connected to the contacts through narrow constraints (nanoribbons) and four side gates to control the electronic properties. (b) Schematic representation of SET based on GQD containing few numbers of atoms, reproduced with permission from [Citation250].](/cms/asset/5a308a1d-a1e1-4af3-81bb-48006a36421d/tapx_a_2048966_f0008_oc.jpg)
Phototransistors are another interesting application of 2D materials and their hybrid systems due to high sensitivity, suitable energy gap, and high carrier mobility [Citation252–254]. Seo et al. reported an ultrasensitive avalanche and broadband light-detection phototransistors based on MoS2 monolayer [Citation255]. 2D-QDs with tunable emission spectra, improved quantum yield, and long lifetime are vital for the development of light-emitting diodes with enhanced efficiency, brightness, compactness, and energy saving [Citation256–262].
In the field of data storage 2D-QDs show good stability, multilevel data storage, high on/off ratio such as PQDs [Citation263,Citation264] and TMDCs-QDs [Citation265,Citation266]. Flash memory is an example of memory devices, in which data are stored as electric charges in discrete charge traps such as quantum dots. Joo et al. have prepared a charge-trap flash memory based on GQDs, see , with controllable memory properties depending on the size of GQDs [Citation267]. A schematic representation of the memory capacitor is shown in such that GQDs are between the tunnel and the control SiO2 layers, located on a p-type Si wafer. The write/erase processes were performed by applying voltage pulses on the Al electrode. In the writing process, under a positive voltage applied to the control gate, electrons tunnel from the substrate (Si) through the tunnel barrier (SiO2) to the conduction band of GQD and are trapped in its continuum states, see the energy band diagram in for the writing process. Applying a negative bias, during the erase process, causes electrons tunneling from GQDs to the Si-conduction band through the SiO2 tunnel barrier.
Figure 9. (a) Schematic representation of GQDs flash-memory capacitors. (b) Image of the memory capacitor. (c) Energy band graph through writing and erasing process. Reproduced with permission from [Citation267].
![Figure 9. (a) Schematic representation of GQDs flash-memory capacitors. (b) Image of the memory capacitor. (c) Energy band graph through writing and erasing process. Reproduced with permission from [Citation267].](/cms/asset/b0addfd5-73cd-46ee-bb83-96e664519b29/tapx_a_2048966_f0009_oc.jpg)
5.2. Spintronics
The interesting magnetic properties of 2D-QDs open the way toward their applications in various semiconductor spin-based applications, including, spin transistors, spin logic switches, spin valves, magnetic tunnel junctions, magnetic field sensors, valleytronic and quantum information technology [Citation268,Citation269]. These spin-based devices have higher performance, lower power consumption, and higher stability than traditional electronics based only on electronic charges. 2D materials with permanent spin such as Rashba spin-orbit coupling in silicene can solve some problems of the spin field-effect transistors with injected spin such as mismatch and spin lifetime [Citation270]. Efficient spin-field switch devices can be achieved in graphene-MoS2 heterostructure with undisturbed spin transport over a long distance due to the weak spin-orbit coupling of graphene and at the same time precise control over the electron spin by the strong spin-orbit coupling of MoS2 [Citation271].
Magnetic tunnel junction (MTJ) is a device consisting of ferromagnetic layers separated by a very thin insulating layer. The resistance of this device depends on the magnetization direction in ferromagnetic layers that can be controlled by an external magnetic field. In the parallel orientation, the resistance is lowest while in the antiparallel orientation the resistance is highest. MTJ is a basic principle on which many important applications work, such as magnetic field sensors, magnetoresistive random access memory (MRAM), and read heads for hard drives. 2D materials, especially those with intrinsic ferromagnetism and high-spin polarization, bring many opportunities for the future-generation of MTJ [Citation272].
TMDCs have strong spin-valley coupling due to inversion symmetry breaking and spin-orbit coupling [Citation273]. The strength of the coupling can be further increased by decreasing the size. For instance, the spin-valley coupling of 400 meV in WS2 monolayer can be increased to 570 meV in WS2-QDs (size ~8–15 nm) or even to 821 meV in smaller WS2-QDs (size ~1.8–3.8 nm) [Citation274,Citation275]. Therefore, WS2-QDs with giant spin-valley coupling due to quantum confinement are excellent candidates for valleytronics. Regarding quantum information processing, the spin of an electron in QDs can be used to form the two-level system of a qubi, and then an array of such quantum dots with controllable coupling can work as spin-based quantum computers [Citation276,Citation277]. The tunable properties provided by 2D-QDs such as graphene and TMDCs quantum dots motivate many researchers to investigate their use as spin qubits with long coherence times and fast operation times [Citation278–281].
5.3. Energy
The energy applications of 2D-QDs can be divided into two categories, (a) energy storage and (b) energy conversion.
5.3.1. Energy storage
Supercapacitors are examples of widely studied energy storage devices due to their high power density, ultrafast charging/discharging rate, long cycle lifetime, and flexibility [Citation282–284]. The ultimate surface area, interactive edge states, defects, and doping of 2D-QDs can help construct highly efficient supercapacitors [Citation285]. For instance, GQDs doped with nitrogen and MoS2-QDs present outstanding electrochemical activity as negative and positive electrodes, respectively. Namely, the prepared N-GQDs/MoS2-QDs supercapacitor exhibits high operating voltage of 1.5 V, extremely fast frequency response, high energy density of 0.55 mWh cm−3, and long-term cycling stability [Citation286]. Moreover, supercapacitors from Ti3C2Tx/Ti3C2Tx QDs /reduced graphene oxide have high capacitance and excellent flexibility. The constructed device shows a volumetric capacitance of 53.1 F cm–3 and good cycle stability of 96.6% after 5000 cycles. Additionally, it has an operating voltage of up to 1.5 V and a volumetric energy density of 16.6 mWh cm–3 [Citation287]. Another energy storage example is batteries with higher energy storage compared to supercapacitors, but they can take hours to be recharged. This difference is mainly due to the operating mechanisms in both devices, where batteries employ chemical reactions to create and store electrical energy, while supercapacitors store electrical energy using the mechanism of electric double-layer effect. In recent years, tremendous efforts in the field of energy storage have been dedicated to explore new materials that can combine the high energy density of batteries and short charging time of supercapacitors [Citation288]. 2D materials have the potential to improve the power and rate capabilities of supercapacitors and at the same time enhance the energy density up to that of batteries [Citation289–291]. Pan et al. have fabricated a 3D doped porous-graphene hydrogel as electrodes for ultrahigh energy density supercapacitors. The designed supercapacitor with a commercial-level graphene mass loading (150 µm, ~10 mg cm−2) can deliver an ultrahigh energy density of 38.5 Wh kg−1 that is comparable to that of lead-acid batteries (35–40 Wh kg−1) but with an ultrahigh power density of 83 kW kg−1 and a long cycle life [Citation292].
5.3.2. Energy conversion
Photovoltaic cells, by which sunlight is converted to electricity, are an important application of 2D-QDs in the field of energy conversion that has grasped significant attention in recent years [Citation65,Citation293–296]. Due to the wide range of light absorption, high quantum yield, generation of multiple exactions, and ultrafast carrier transfer, 2D materials are expected to construct the next-generation solar cells [Citation297–301]. Graphene with its high transparency and conductivity can be used as electrodes (anode or cathode) in the solar cells. In contrast, TMDCs with direct band gap and strong light–matter interaction can be used as the active semiconductor materials. 2D-QDs can be used in organic or perovskite solar cells as electrodes, electron/hole extraction, and interfacial layers [Citation302]. The working mechanism of organic or heterojunction-based solar cells is shown in . The absorbed sunlight photon generates electron –hole pairs (excitons) in the donor material. The excitons with moderate binding energy are then dissociated into free electrons and holes at the interface of the donor-acceptor. Finally, the electrons and holes move through the electron transport layer (ETL) and hole transport layer (HTL), respectively, toward the electrodes and operate at the external circuit. The perovskite solar cell shown in has a slightly different operating principle, where the perovskite active material absorbs the sunlight to generate excitons. The dissociation of electrons and holes occurs at the interface between the perovskite material and the charge transporting layers, as seen in ).
Figure 10. Band alignment of different layers in organic (a) and perovskite solar cells (b) presenting their working principles, reproduced with permission from ref [Citation301]. Heterojunction solar cell based on 2D-QDs (c), its band diagram (d), and the resultant PCE (e), reproduced with permission from [Citation313].
![Figure 10. Band alignment of different layers in organic (a) and perovskite solar cells (b) presenting their working principles, reproduced with permission from ref [Citation301]. Heterojunction solar cell based on 2D-QDs (c), its band diagram (d), and the resultant PCE (e), reproduced with permission from [Citation313].](/cms/asset/4c4c1127-0189-4ee9-b469-fb2239cc8551/tapx_a_2048966_f0010_oc.jpg)
Perovskite solar cells are characterized by a low exciton binding energy (~0.05 eV), which facilitates exciton dissociation with minimal energy loss associated with the dissociation and migration [Citation303]. They also have a suitable band gap and absorption coefficient higher than 104 cm−1 in the visible region, making them superior to all other solar cells [Citation304].
We now turn to the integration of 2D-QDs into the solar cell devices and their capabilities to boost the power conversion efficiency (PCE). Chen et al. found that PQDs with high hole mobility over 100 cm2/(V.s) integrated at the anode side of hybrid perovskite solar cells improve the performance (PCE increases from 14.10% to 16.69%) by enhancing the hole-extraction and suppression of recombination at the anode [Citation305]. The performance of bulk heterojunction solar cells can be improved by adding GQDs, where the functional groups on the surface of GQDs enhance the electron extraction and light absorption [Citation306]. TMDCs-QDs have been used as ETL, interfacial layer, and HTL in organic solar cells [Citation307,Citation308]. For instance, the performance of inorganic solar cells is boosted by manipulating the size of MoS2-QDs as an HTL [Citation309]. All-2D heterojunction solar cells have also been investigated which are vertically/laterally aligned 2D materials with type-II band alignment suitable for solar cells. Different 2D-based heterojunction solar cells were constructed, such as Phosphorene/MoS2 [Citation310], MoS2/WSe2 [Citation311], and Te/WTe2 [Citation312]. Heterojunction solar cells based on 2D-QDs have also been reported with superior PCE [Citation295,Citation313]. We recently designed a heterojunction solar cell from silicene and graphene QDs, see , and proved that the shape, size, and edge termination are crucial factors for boosting the PCE up to 23.34% [Citation313].
Water splitting is a chemical process in which water decomposes into hydrogen and oxygen. In this process, 2D-QDs can be used as photocatalysts or electrocatalysts that enhance the conversion of solar energy or electrical energy into chemical energy (hydrogen). Water splitting is divided into two main reactions: oxygen evolution reaction (OER) at the anode and hydrogen evolution reaction (HER) at the cathode as given by the following equations.
Where * represents the catalyst. The significant enhancement of HER using 2D-QDs as catalysts has been previously discussed in section (4.4), thus we will discuss only OER here. OER is very important for renewable energy conversion and storage devices, such as fuel cells and researchable metal-air batteries [Citation314–316]. Efficient electrocatalysts for OER can be characterized by low overpotential, low Tafel slope, high stability, and cost-effective. So far, precious-metal oxides, e.g., RuO2 and IrO2, have been benchmarked as OER electrocatalysts; however, they have limited stability and high cost. Therefore, it is highly important to search for OER electrocatalysts based on new materials that are efficient, low cost and stable. 2D-QDs with abundant active sites, defect-rich, highly exposed surface area, and functionalized surface showed enhanced OER activity such as functionalized PQDs [Citation39], MoS2-QDs [Citation317], GQDs [Citation318], MQDs [Citation319]. Prasannachandran et al. reported that chemically functionalized PQDs are efficient OER electrocatalysts with an overpotential of 1.66 V, a low Tafel slope of 48 mV dec−1, and excellent stability [Citation39]. The reach crystal defects in T3C2 MQDs significantly lower the OER overpotential to 0.37 V [Citation319]. Moreover, PQDs/MXenes heterostructures showed exceptional bifunctional electrocatalytic performance for both OER and HER. Namely, an overpotential of 360 mV and a Tafel slope of 64.3 mV dec−1 have been achieved for OER, for HER, the overpotential equals 190 mV and the Tafel slope equals 83 mV dec−1[Citation320].
5.4. Water purification
2D membranes capture a great attention due to their potential applications in water purification [Citation321–325]. 2D materials open the way toward new approaches for the improvement of membranes that bulk materials cannot achieve, for examples, controllable pore size, non-corrosive property, high mechanical strength, tunable surface chemistry, ultrathin structure, high adsorption, and high permeation rate [Citation326–328]. We showed that graphene, hexagonal boron nitride, and Si2BN QDs are capable of removing various toxic metals from wastewater with high adsorption energy and negligible deformation [Citation131,Citation133,Citation329]. Regarding the controllable pore size, Jacobse et al. demonstrated a novel bottom-up approach to fabricate nanoporous graphene with precise nanopores topologies using dihalide monomers as seen in [Citation330]. The fabrication process consists of three steps: (a) aromatic dihalide monomers are covalently coupled to form polymer chain, (b) aromatization of polymer chain by dehydrogenation of the cyclic compounds to form 2D nanoribbons, and (c) the final step is the lateral binding of nanoribbons through selective dehydrogenative coupling. Based on this idea, the pore size can have additional control by using different monomers such as Hexakis(4-bromophenyl)benzene (HBPB, )) to synthesize porous graphene, which looks like laterally connected hexagonal GQDS [Citation331]. Layer stacking can also enhance the adsorption and sieving properties of 2D membranes. As shown in , in monolayer membranes some metal ions can sieve through the nanopores; however, by formation of multilayer 2D membranes, these ions are blocked between the layers [Citation332]. Therefore, 2D membranes are bifunctional materials for wastewater treatment due to high adsorption energy and surface area, as well as for seawater desalination due to their ability to permute/block water/salt molecules.
Figure 11. (a) On surface bottom-up synthesis of chevron-type nanoporous graphene from molecular precursors 1 or 2, reproduced with permission from ref [Citation330]. (b) Schematic representation of surface-assist bottom-up synthesis of nanoporous graphene using HBPB as the molecular precursor, reproduced with permission from ref [Citation331]. (c) An illustration of pollutant rejection by nanoporous graphene for molecules larger than the pore size. (d) Rejection of various pollutant through nanopores and interlayer blocking in multilayer nanoporous graphene, at the same time easy permeation of water molecules.
![Figure 11. (a) On surface bottom-up synthesis of chevron-type nanoporous graphene from molecular precursors 1 or 2, reproduced with permission from ref [Citation330]. (b) Schematic representation of surface-assist bottom-up synthesis of nanoporous graphene using HBPB as the molecular precursor, reproduced with permission from ref [Citation331]. (c) An illustration of pollutant rejection by nanoporous graphene for molecules larger than the pore size. (d) Rejection of various pollutant through nanopores and interlayer blocking in multilayer nanoporous graphene, at the same time easy permeation of water molecules.](/cms/asset/62806371-8e51-41f3-b316-0e1e3bb974e0/tapx_a_2048966_f0011_oc.jpg)
5.5. Sensors
The high sensitivity, selectivity, tunable photoluminescence, and non-toxicity of 2D-QDs show great potential for both chemosensors and biosensors [Citation333–336]. Chemosensors are sensory receptors that can interact with the analyte and produce detectable signals [Citation337]. Biosensors have the same working principle with the only difference of attaching biological recognition receptors to the nanomaterials such as antibodies, and enzymes, DNA etc [Citation338]. Emissive chemosensors based on 2D-QDs with noticeable change in the PL, because of interaction with the analyte, are used as efficient fluorescent probes for various analytes including metal ions [Citation339–344], gases [Citation345–349], and organic molecules [Citation350–354]. Electrochemical sensors from 2D-QDs have also been investigated [Citation355–358]. With respect to biosensors, the 2D-QDs need to be modified with recognition receptors, GQDs modified with antibodies form immunosensors with high sensitivity and specificity toward Yersinia enterocolitica [Citation359]. Ge et al. constructed a highly sensitive fluorescence biosensor for microRNA detection by coupling MoS2-QDs with nicking-enhanced rolling circle amplification [Citation360]. Moreover, a naked eye fluorescence probe for uric acid has been developed based on the fluorescence quenching of glutathione functionalized Ti3C2 QDs and uricase/HRP enzymes [Citation361].
5.6. Biological applications
In addition to drug delivery and biosensing discussed in sections 4.5 and 5.5, respectively, 2D-QDs show promising properties for other biological applications including bioimaging and phototherapy [Citation362]. Photoluminescent 2D-QDs such as GQDs [Citation363,Citation364], g-C3N4-QDs [Citation365], PQDs [Citation366], MoS2-QDs [Citation367], and MQDs [Citation368] have drawn significant interest in the field of bioimaging due to their intriguing optical emission properties, stability, and biocompatibility. Fluorescence imaging, which is a non-invasive tool that visualizes biological processes using the photons emitted from a fluorescence probe, provides a highly versatile platform for molecular imaging [Citation369]. Recently, M. Shi et al. reported a bottom-up synthesis of MoS2-QDs with excellent fluorescence properties and biocompatibility that enabled fluorescence imaging of SW480 tumor cells in vitro and in vivo [Citation370]. Other types of bioimaging based on 2D-QDs such as magnetic resonance, computed tomography, and photoacoustic imaging have also been investigated [Citation203].
Phototherapy based on 2D-QDs such as GQDs [Citation371], g-C3N4 [Citation372], MoS2 [Citation373], and MQDs [Citation374] demonstrated high capability to treat various diseases [Citation375]. The phototherapy process starts by directing the phototherapeutic agent to the diseased part and then irradiated with light. In photodynamic therapy, the absorbed light generates reactive oxygen species that induces irreversible damage to tumor cells [Citation376]. The second type of phototherapy is the photothermal therapy in which the absorbed infrared light by the agent is converted to heat that causes cells ablations as schematically shown in for Mo2C-QDs as the photothermal agent [Citation377].
Figure 12. Schematic representation of Mo2C-QDs for photothermal therapy of cancer, reproduced with permission from ref [Citation377].
![Figure 12. Schematic representation of Mo2C-QDs for photothermal therapy of cancer, reproduced with permission from ref [Citation377].](/cms/asset/6225630c-1501-4bcd-880d-059007b6823a/tapx_a_2048966_f0012_oc.jpg)
6. Outlook
Motivated by their outstanding properties, 2D-QDs are expected to form a cornerstone in building future nanodevices. Significant advances in experimental techniques facilitate the synthesis of various 2D-QDs including graphene, silicene, TMDCs, MXenes, etc. with enhanced and tunable physical and chemical properties. However, atomic precision synthesis of 2D-QDs with definite shape, edge termination, layer number, and nanopores has been achieved only in GQDs. Therefore, future work should focus on atomic precision synthesis of different 2D-QDs such as triangular shapes with zigzag termination that show exceptional magnetic and catalytic properties. There are a huge number of 2D materials, either theoretically predicted or synthesized. Since 2D-QDs have improved properties, such as electronic energy gap, photoluminescence, electron density around Fermi level and active edge states, with respect to their bulk 2D counterpart, it is highly important to investigate QDs derived from them. For instance, the recently fabricated 2D magnets (e.g. CrI3 and VS2) are expected to show many interesting properties in the quantum regime. Heteroatom doping of 2D-QDs, which is an important tool to control the physical and chemical properties of a material, is still in its infancy. Systematic investigations on the effect of doping with single atoms (such as S, N, P, or metal-atoms) or co-doping (N-S, N-P, N-Pt, etc.) on 2D-QDs based on TMDCs, MXenes, etc. are required because it can open up the way for applications in catalysis and energy. Hybrid heterostructures based on 2D-QDs, e.g. 0D/2D (GQDs/2D-metals or metal-QDs/WS2) and 0D/0D (GQDs/VSe2-QDs or PQDs/MXenes), worth more investigations due to their capability to combine the privileges of different materials in one heterostructure.
Acknowledgments
This work is supported by the National Natural Science Foundation of China (No. 11774178), the Natural Science Foundation of Jiangsu Province (BK20211361, BX2021054), and College Natural Science Research Project of Jiangsu Province (20KJA430004).
Disclosure statement
No potential conflict of interest was reported by the author(s).
Additional information
Funding
References
- Lee C, Xiaoding W, Kysar JW, et al. Measurement of the elastic properties and intrinsic strength of monolayer graphene. Science. 2008;321:385.
- Akinwande D, Brennan CJ, Bunch JS, et al. A review on mechanics and mechanical properties of 2D materials—graphene and beyond. Extreme Mech Lett. 2017;13:42.
- Androulidakis C, Zhang K, Robertson M, et al. Tailoring the mechanical properties of 2D materials and heterostru`ctures. 2D Mater. 2018;5:032005.
- Balandin AA, Ghosh S, Bao W, et al. Superior thermal conductivity of single-layer graphene. Nano Lett. 2008;8:902.
- Zheng J-C, Tsuruda K, Lightfoot CB, et al. High thermal conductivity of hexagonal boron nitride laminates. 2D Mater J Med Imaging (Bellingham, Wash). 2016;3:011004.
- Mortazavi B, Javvaji B, Shojaei F, et al. Exceptional piezoelectricity, high thermal conductivity and stiffness and promising photocatalysis in two-dimensional MoSi2N4 family confirmed by first-principles. Nano Energy. 2021;82:105716.
- Cao M, Xiong D-B, Yang L, et al. Ultrahigh Electrical conductivity of graphene embedded in metals. Adv Funct Mater. 2019;29:180679.
- Jiao Y, Hafez AM, Cao D, et al. Metallic MoS2 for high performance energy storage and energy conversion. Small. 2018;14:1800640.
- Guo Z, Gao L, Xu Z, et al. High electrical conductivity 2D MXene serves as additive of perovskite for efficient solar cells. Small. 2018;14:1802738.
- Mayorov AS, Gorbachev RV, Morozov SV, et al. Micrometer-scale ballistic transport in encapsulated graphene at room temperature. Nano Lett. 2011;11:2396.
- Mir SH, Yadav VK, Singh JK. Recent advances in the carrier mobility of two-dimensional materials: a theoretical perspective. ACS Omega. 2020;5:14203.
- Wang QH, Kalantar-Zadeh K, Kis A, et al. Electronics and optoelectronics of two-dimensional transition metal dichalcogenides. Nat Nanotechnol. 2012;7:699.
- Weng Q, Li G, Feng X, et al. Electronic and optical properties of 2D materials constructed from light atoms. Adv Mater. 2018;30:1801600.
- Stoller MD, Park S, Zhu Y, et al. Graphene-based ultracapacitors. Nano Lett. 2008;8:3498.
- Yang W, Gan L, Li H, et al. Two-dimensional layered nanomaterials for gas-sensing applications. Inorg Chem Front. 2016;3:433.
- Zhang H. Ultrathin Two-DimensionalNanomaterials. ACS nano. 2015;9:9451.
- “MatWeb – the online materials information resource”. Archived from the original on 15 December 2013. Retrieved 20 February 2015.
- Falin A, Cai Q, Santos EJG, et al. Mechanical properties of atomically thin boron nitride and the role of interlayer interactions. Nat Commun. 2017;8.
- Bertolazzi S, Brivio J, Kis A. Stretching and breaking of ultrathin MoS2. ACS Nano. 2011;5:9703.
- Lemme MC, Wagner S, Lee K, et al. Nanoelectromechanical sensors based on suspended 2D materials. AAAS Res.Volume 2020; Article ID 8748602; . DOI:10.34133/2020/8748602
- Yuan C, Li J, et al. Modulating the thermal conductivity in hexagonal boron nitride via controlled boron isotope concentration. Commun Phys. 2019;2:1.
- Lin Z, Liu C, Chai Y. High thermally conductive and electrically insulating 2D boron nitride nanosheet for efficient heat dissipation of high-power transistors. 2D Mater. 2016;3:041009.
- Song H, Liu J, Liu B, et al. Two-dimensional materials for thermal management applications. Joule. 2018;2:442.
- Qiao J, Kong X, Hu Z-X, et al. High-mobility transport anisotropy and linear dichroism in few-layer black phosphorus. Nat Commun. 2014;5:4475.
- Tan C, Cao X, Wu X-J, et al. Recent advances in ultrathin two-dimensional nanomaterials. Chem Rev. 2017;117:6225.
- Xia FN, Wang H, Xiao D, et al. Two-dimensional material nanophotonics. Nat Photonics. 2014;8:899.
- Novoselov KS, Mishchenko A, Carvalho A, et al. 2D materials and van der Waals heterostructures. Science. 2016;353:aac9439.
- Ma Q, Ren G, Xu K, et al. Tunable optical properties of 2D materials and their applications. Adv Opt Mater. 2021;9:2001313.
- Liao Y, Song C, Xiang Y, et al. Recent advances in spatial self-phase modulation with 2D materials and its applications. Ann Phys (Berlin). 2020;532:2000322.
- Yao Y, Cheng Z, Dong J, et al. Performance of integrated optical switches based on 2D materials and beyond. Front Optoelectron. 2020;13:129.
- Shen Y, Harris NC, Skirlo S, et al. Deep learning with coherent nanophotonic circuits. Nat Photonics. 2017;11:441.
- Qiuab Z, Tang D. Nanostructure-based photoelectrochemical sensing platforms for biomedical applications. J Mater Chem B. 2020;8:2541.
- Wang J, Liu Z. Recent advances in two-dimensional layered materials for photoelectrochemical sensing. Trends Analyt Chem. 2020;133:1160892.
- Ramalingam G, Kathirgamanathan P, Manivannan N, et al. Quantum confinement effect of 2D nanomaterials. In: Quantum dots-fundamental and applications. London UK:IntechOpen; 2020
- Ponomarenko LA, Schedin F, Katsnelson MI, et al. Chaotic dirac billiard in graphene quantum dots. Science. 2008;356. DOI:10.1126/science.1154663.
- Peng J, Gao W, Gupta BK, et al. Graphene quantum dots derived from carbon fibers. Nano Lett. 2012;12:844.
- Abdelsalam H, Talaat MH, Lukyanchuk I, et al. Electro-absorption of silicene and bilayer graphene quantum dots. J Appl Phys. 2016;120:014304.
- Kabel J, Sharma S, Acharya A, et al. Molybdenum disulfide quantum dots: properties, synthesis, and applications. C. 2021;7:45.
- Prasannachandran R, Vineesh TV, Anil A, et al. Functionalized phosphorene quantum dots as efficient electrocatalyst for oxygen evolution reaction. ACS Nano. 2018;12:11511.
- Abdelsalam H, Saroka VA, Younis WO. Phosphorene quantum dot electronic properties and gas sensing. Phys E Low Dimens Syst Nanostruct. 2019;107:105.
- Jin SH, Kim DH, Jun GH, et al. Tuning the photoluminescence of graphene quantum dots through the charge transfer effect of functional groups. ACS Nano. 2013;7:1239.
- Yin W, Bai X, Zhang X, et al. Multicolor light-emitting diodes with MoS2 quantum dots. Part Syst Char. 2019;36:1800362.
- Nguyen V, Yan L, Zhao N, et al. Tuning photoluminescence of boron nitride quantum dots via surface functionalization by femtosecond laser ablation. J Mol Struct. 2021;1244:130922.
- Gobi N, Vijayakumar D, Keles O, et al. Infusion of graphene quantum dots to create stronger, tougher, and brighter polymer composites. ACS Omega. 2017;2:4356.
- Huang D, Zhang B, Tang H, et al. Colloids Surf. A Physicochem Eng Asp. 2018;538.
- Tao W, Ji X, Xu X, et al. Antimonene Quantum dots: synthesis and application as near infrared photothermal agents for effective cancer therapy. Angew Chem. 2017;129.
- Xu Y, Wang X, Zhang WL, et al. Recent progress in two-dimensional inorganic quantum dots. Chem Soc Rev. 2018;47:586. DOI:10.1039/c7cs00500h
- Ouyang J, Feng C, Ji X, et al. 2D monoelemental Germanene quantum dots: synthesis as robust photothermal agents for photonic cancer nanomedicine. Angew Chem. 2019;131:13539.
- Das S, Ngashangva L, Goswami P. Carbon dots: an emerging smart material for analytical applications. Micromachines. 2021;12:84.
- Yang H, Zhao Q, Wang X, et al. Facile and highly selective sensing of hypochlorous acid in aqueous solution and living cells by using as-prepared WSe2 quantum dots. Sens Actuators B Chem. 2021;337:129782.
- Zhu H, Liu A, Xu Y, et al. Graphene quantum dots directly generated from graphite via magnetron sputtering and the application in thin-film transistors. Carbon. 2015;88:225.
- Mouafo LDN, Godel F, Simon L, et al. 0D/2D heterostructures vertical single electron transistor. Adv Funct Mater. 2021;31:2008255. DOI:10.1002/adfm.202008255.
- Kufer D, Nikitskiy I, Lasanta T, et al. Hybrid 2D–0D MoS2–PbS quantum dot photodetectors. Adv Mater. 2015;27:176.
- Mahalingam S, Manap A, Omar A, et al. Functionalized graphene quantum dots for dye-sensitized solar cell: key challenges, recent developments and future prospects, renew. Sustain Energy Rev. 2021;144:110999.
- Li BL, Setyawati MI, Zou HL, et al. Emerging 0D transition-metal dichalcogenides for sensors. Biomed Clean Energy Small. 2017;13:1700527.
- Yolaa ML, Atar N. A novel detection approach for serotonin by graphene quantum dots/two-dimensional (2D) hexagonal boron nitride nanosheets with molecularly imprinted polymer. Appl Surf Sci. 2018;458:648.
- Wang H, Feng H, Li J. Graphene and graphene‐like layered transition metal dichalcogenides in energy conversion and storage. Small. 2014;10:2165.
- Wang X, Sun G, Li N, et al. Quantum dots derived from two-materials and their applications for catalysis and energy. Chem Soc Rev. 2016;45:2239.
- Trauzettel B, Bulaev DV, Loss D, et al. Spin qubits in graphene quantum dots. Nat Phys. 2007;3:192.
- Tong C, Garreis R, Knothe A, et al. Tunable valley splitting and bipolar operation in graphene quantum dots. Nano Lett. 2021;21:1068–46.
- Hu W, Huang Y, Qin X, et al. Room-temperature magnetism and tunable energy gaps in edge-passivated zigzag graphene quantum dots. npj 2D Mater Appl. 2019;3:17.
- Abdelsalam H, Yunokic S, Zhang Q. Boosted spintronic properties in triangular Si-based nanoflakes. Phys E. 2021;130:114699.
- Kevin PM, Ibrahim KH, Yavuz M. Research update: beyond graphene—synthesis of functionalized quantum dots of 2D materials and their applications. APL Mater. 2018;6:120701.
- Shaari N, Kamarudin SK, Bahru R. Carbon and graphene quantum dots in fuel cell application: an overview. Int J Energy Res. 2021;45:1396–1424.
- Chen X, Liu Q, Wu Q, et al. Incorporating graphitic carbon nitride (g-C3N4) quantum dots into bulk-heterojunction polymer solar cells leads to efficiency enhancement. Adv Funct Mater. 2016;26:1719.
- Zheng XT, Ananthanarayanan A, Luo KQ, et al. Glowing graphene quantum dots and carbon dots: properties, syntheses, and biological applications. Small. 2015;11:1620.
- Park SJ, Pak SW, Qiu D, et al. Structural and optical characterization of MoS2 quantum dots defined by thermal annealing. J Lumin. 2017;183:62.
- Yu XH, Cai X, Cui H, et al. Fluorine-free preparation of titanium carbide MXene quantum dots with high near-infrared photothermal performances for cancer therapy. Nanoscale. 2017;9:17859–17864.
- Qiao W, Yan S, Song X, et al. Luminescent monolayer MoS2 quantum dots produced by multi-exfoliation based on lithium intercalation. Appl Surf Sci. 2015;359:130.
- Mao H, Gu C, Yan S, et al. MXene quantum dot/polymer hybrid structures with tunable electrical conductance and resistive switching for nonvolatile memory devices. Adv Electron Mater. 2020;6:1900493.
- Neupane GP, Wang B, Tebyetekerwa M, et al. Highly enhanced light–matter interaction in mxene quantum dots–monolayer WS2 heterostructure. Small. 2021;17:2006309.
- Qin Y, Wang Z, Liu N, et al. High-yield fabrication of Ti3 C2Tx MXene quantum dots and their electrochemiluminescence behavior. Nanoscale. 2018;10:14000–14004.
- Alijani H, Rezk AR, Khosravi Farsani MM, et al. Acoustomicrofluidic synthesis of pristine ultrathin Ti3C2Tz MXene nanosheets and quantum dots. ACS Nano. 2021;15:12099.
- Liu R, Wu D, Feng X, et al. Bottom-up fabrication of photoluminescent graphene quantum dots with uniform morphology. J Am Chem Soc. 2011;133:15221.
- Ahmad P, Khandaker MU, Muhammad N, et al. Fabrication of hexagonal boron nitride quantum dots via a facile bottom-up technique. Ceram Int. 2019;45:22765.
- Ren X, Pang L, Zhang Y, et al. One-step hydrothermal synthesis of monolayer MoS2 quantum dots for highly efficient electrocatalytic hydrogen evolution. J Mater Chem A. 2015;3:10693–10697.
- Vikraman D, Akbar K, Hussain S, et al. Direct synthesis of thickness-tunable MoS2 quantum dot thin layers: optical, structural and electrical properties and their application to hydrogen evolution. Nano Energy. 2017;35:101.
- Kaur M, Kaura M, Sharma VK. Nitrogen-doped graphene and graphene quantum dots: a review on synthesis and applications in energy, sensors and environment. Adv Colloid Interface Sci. 2018;259:44.
- Chen Y, Xu X, Li C, et al. Bottom-up synthesis of hexagonal boron nitride nanoparticles with intensity-stabilized quantum emitters. Small. 2021;17:2008062.
- Veeramalai CP, Li F, Guoa T, et al. Highly flexible memristive devices based on MoS2 quantum dots sandwiched between PMSSQ layers. Dalton Trans. 2019;48:2422.
- Wang L, Weitao L, Wu B, et al. Room-temperature synthesis of graphene quantum dots via electron-beam irradiation and their application in cell imaging. Chem Eng J. 2017;309:374.
- Shao B, Liu Z, Zeng G, et al. Two-dimensional transition metal carbide and nitride (MXene) derived quantum dots (QDs): synthesis, properties, applications and prospects. J Mater Chem A. 2020;8:7508.
- Sharbirin AS, Akhtar S, Kim JY. Light-emitting MXene quantum dots. Opto-Electron Adv. 2021;4:200077.
- Cheng H, Ding L-X, Chen G-F, et al. Molybdenum carbide nanodots enable efficient electrocatalytic nitrogen fixation under ambient conditions. Adv Mater. 2018;30:e1803694.
- Miro P, Audiffred M, Heine T. An atlas of two-dimensional materials. Chem Soc Rev. 2014;43:6537.
- Bhimanapati GR, Lin Z, Meunier V, et al. Recent advances in two-dimensional materials beyond graphene. ACS Nano. 2015;9:11509.
- Tan C, Cao X, Wu X-J, et al. Recent advances in ultrathin two-dimensional nanomaterials. Chem Rev. 2017;117:6225.
- Xu Q, Cai W, Li W, et al. Two-dimensional quantum dots: fundamentals, photoluminescence mechanism and their energy and environmental applications. Mater Today Energy. 2018;10:222.
- Zhai W, Xiong T, He Z, et al. Nanodots Derived from layered materials: synthesis and applications. Adv Mater. 2021;33:2006661.
- Novoselov KS, Geim AK, Morozov SV, et al. Electric field effect in atomically thin carbon films. Science. 2004;306:666.
- Kang J, Wei ZG, Li J. Graphyne and Its family: recent theoretical advances. ACS Appl Mater Interfaces. 2019;11:3.
- Xie Z, Meng X, Li X, et al. Two-dimensional borophene: properties, fabrication, and promising applications. Research. 2020;2020:1. DOI:10.34133/2020/2624617
- Vogt P, De Padova P, Quaresima C, et al. Compelling experimental evidence for graphene like two-dimensional silicon. Phys Rev Lett. 2012;108:155501.
- Zhu -F-F, Chen W-J, Xu Y, et al. Epitaxial growth of two-dimensional stanene. Nat Mater. 2015;14:1020.
- Liu H, Neal AT, Zhu Z, et al. Phosphorene: an unexplored 2D semiconductor with a high hole mobility. ACS Nano. 2014;8:4033.
- Ji J, Song X, Liu J, et al. Two-dimensional antimonene single crystals grown by van der Waals epitaxy. Nat Commun. 2016;7:13352.
- Zhang K, Feng Y, Wang F, et al. Two dimensional hexagonal boron nitride (2D-hBN): synthesis, properties and applications. J Mater Chem C. 2017;5:11992.
- Zhou W, Zhang S, Cao J, et al. Modulating tunneling width and energy window for high-on-current two-dimensional tunnel field-effect transistors. Nano Energy. 2021;81:105642.
- Wang Y, Liu L, Ma T, et al. 2D graphitic carbon nitride for energy conversion and storage. Adv Funct Mater. 2021;31:2102540.
- Chen P, Zhang X-J, Liu B-G. Mechanically-controllable strong 2d ferroelectricity and optical properties of semiconducting BiN monolayer. ACS Appl Nano Mater. 2019;2:58.
- Manzeli S, Ovchinnikov D, Pasquier D, et al. 2D transition metal dichalcogenides. Nat Rev Mater. 2017;2:17033.
- Kumbhakar P, Gowda CC, Mahapatra PL, et al. Emerging 2D metal oxides and their applications. Mater Today. 2021;45.
- Andriotis AN, Richter E, Menon M. Prediction of a new graphene like Si2BN solid. Phys Rev B. 2016;93:081413.
- Anasori BK, Lukatskaya MR, Gogotsi Y. 2D metal carbides and nitrides (MXenes) for energy storage. Nat Rev Mater. 2017;2:16098.
- Deng Z, Chen D, Peng B, et al. From bulk metal Bi to two-dimensional well-crystallized BiOX (X = Cl, Br) micro- and nanostructures: synthesis and characterization. Cryst Growth Des. 2008;8:2995.
- Song J, Xu L, Li J, et al. Monolayer and few-layer all-inorganic perovskites as a new family of two-dimensional semiconductors for printable optoelectronic devices. Adv Mater. 2016;28:4861.
- Chen S, Shi G. Two-dimensional materials for halide perovskite-based optoelectronic devices. Adv Mater. 2017;29:1605448.
- Mounet N, Gibertini M, Schwaller P, et al. Two-dimensional materials from high-throughput computational exfoliation of experimentally known compounds. Nat Nanotechnol. 2018;13:246.
- Mounet N, Gibertini M, Schwaller P, et al. Two-dimensional materials from high-throughput computational exfoliation of experimentally known compounds (data download). Mater Cloud Arch. 2017; DOI:10.24435/materialscloud:2017.0008/v1.
- Chung S, Revia RA, Zhang M. Graphene quantum dots and their applications in bioimaging, biosensing, and therapy. Adv Mater. 2021;33:1904362.
- Li H, Wang Y, Dai X, et al. Tailoring the lateral size of two-dimensional silicon nanomaterials to produce highly stable and efficient deep-blue emissive silicene-like quantum dots. J Mater Chem C. 2021;9:10065.
- Prasannachandran R, Vineesh TV, Anil A, et al. Functionalized phosphorene quantum dots as efficient electrocatalyst for oxygen evolution reaction. ACS Nano. 2018;12:11511.
- Tao W, Ji X, Xu X, et al. antimonene quantum dots: synthesis and application as near-infrared photothermal agents for effective cancer therapy. Angew Chem. 2017;129:12058.
- Devia M, Das P, Boruah PK, et al. Fluorescent graphitic carbon nitride and graphene oxide quantum dots as efficient nanozymes: colorimetric detection of fluoride ion in water by graphitic carbon nitride quantum dots. J Environ Chem Eng. 2021;9:104803.
- Lin L, Xu Y, Zhang S, et al. Fabrication and luminescence of monolayered boron nitride quantum dots. small. 2014;10:60.
- Zhang J, Ling C, Zang W, et al. Boosted electrochemical ammonia synthesis by high-percentage metallic transition metal dichalcogenide quantum dots. Nanoscale. 2020;12:10964.
- Xue Q, Zhang H, Zhu M, et al. Photoluminescent Ti3C2 MXene Quantum dots for multicolor cellular imaging. Adv Mater. 2017;29:1604847.
- Chang Y-H, Lin J-C, Chen Y-C, et al. Facile synthesis of two-dimensional ruddlesden–popper perovskite quantum dots with fine-tunable optical properties. Nanoscale Res Lett. 2018;13:247.
- Wang LJ, Cao G, Tu T, et al. A graphene quantum dot with a single electron transistor as an integrated charge sensor. Appl Phys Lett. 2010;97:262113.
- Harrison P. Quantum wells, wires and dots. Wiley; 2005.
- Tian P, Tang L, Teng KS, et al. Graphene quantum dots from chemistry to applications. Mater Today Chem. 2018;10:221.
- Eda G, Lin -Y-Y, Mattevi C, et al. Blue photoluminescence from chemically derived graphene oxide. Adv Mater. 2010;22:505.
- Ye R, Peng Z, Metzger A, et al. Bandgap engineering of coal-derived graphene quantum dots. ACS Appl Mater Interfaces. 2015;7:7041.
- Yan Y, Gong J, Chen J, et al. Recent advances on graphene quantum dots: from chemistry and physics to applications. Adv Mater. 2019;31:1808283.
- Li H, Tay RY, Tsang SH, et al. Controllable synthesis of highly luminescent boron nitride quantum dots. small. 2015;11:6491.
- Tayyebi A, Ogino N, Hayashi T, et al. Size-controlled MoS2 nanosheet through ball milling exfoliation: parameter optimization, structural characterization and electrocatalytic application. Nanotechnology. 2020;31:075704.
- Osman W, Abdelsalam H, Ali M, et al. Electronic and magnetic properties of graphene quantum dots doped with alkali metals. J Mater Res Technol. 2021;11. DOI:10.1016/j.jmrt.2021.01.119.
- Abdelsalam H, Elhaes H, Ibrahim MA. Tuning electronic properties in graphene quantum dots by chemical functionalization: Density functional theory calculations. Chem Phys Lett. 2018;695:138.
- De Alwis WU, Weerawardene KD, Ellington TL, et al. Electronic structure modification of rectangular phosphorene quantum Dots Via edge passivation. J Phys Chem C. 2021;125:5029.
- Mahvash F, Eissa S, Bordjiba T, et al. Corrosion resistance of monolayer hexagonal boron nitride on copper. Sci Rep. 2017;7:42139.
- Abdelsalam H, O. Younis W, Saroka VA, et al. Interaction of hydrated metals with chemically modified hexagonal boron nitride quantum dots: wastewater treatment and water splitting. Phys Chem Chem Phys. 2020;22:2566.
- Hussain T, Singh D, Gupta SK, et al. Efficient and selective sensing of nitrogen-containing gases by Si2BN nanosheets under pristine and pre-oxidized conditions. Appl Surf Sci. 2019;469:775.
- Abdelsalam H, Ali M, Teleb NH, et al. Two-dimensional Si2BN nanoflakes for efficient removal of heavy metals. Chem Phys Lett. 2021;772:138568.
- Liu H, Su Y, Sun T, et al. Engineering the energy gap of black phosphorene quantum dots by surface modification for efficient chemiluminescence. Chem Commun. 2020;56:1891.
- Saroka VA, Lukyanchuk I, Portnoi ME, et al. Electro-optical properties of phosphorene quantum dots. Phys Rev B. 2017;96:085436.
- Cao X, Ding C, Zhang C, et al. Transition metal dichalcogenide quantum dots: synthesis, photoluminescence and biological applications. J Mater Chem B. 2018;6:8011.
- Zhou BT, Taguchi K, Kawaguchi Y, et al. Spin-orbit coupling induced valley Hall effects in transition-metal dichalcogenides. Commun Phys. 2019;2:26.
- Geim AK, Grigorieva IV. Van der Waals heterostructures. Nature. 2013;499:419.
- Novoselov KS, Mishchenko A, Carvalho A, et al. 2D materials and van der Waals heterostructures. Science. 2016;353. DOI:10.1126/science.aac9439.
- Li M-Y, Chen C-H, Shi Y, et al. Heterostructures based on two-dimensional layered materials and their potential applications. Mater Today. 2016;19:322.
- Hu W, Lin L, Yang C, et al. Edge-modified phosphorene nanoflake heterojunctions as highly efficient solar cells. Nano Lett. 2016;6.
- Shan H, Yu Y, Zhang R, et al. Electron transfer and cascade relaxation dynamics of graphene quantum dots/MoS2 monolayer mixed-dimensional van der Waals heterostructures. Mater Today. 2019;24:10.
- Qiao H, Li Z, Huang Z, et al. Self-powered photodetectors based on 0D/2D mixed dimensional heterojunction with black phosphorus quantum dots as hole acceptors. Appl Mater Today. 2020;20:100765.
- Zhou B-X, Ding -S-S, Wang Y, et al. Type-II/type-II band alignment to boost spatial charge separation: a case study of g-C3N4 quantum dots/a-TiO2/r-TiO2 for highly efficient photocatalytic hydrogen and oxygen evolution. Nanoscale. 2020;12:6037.
- Han W, Kawakami RK, Gmitra M, et al. Graphene spintronics. Nat Nanotechnol. 2014;9:794.
- Sepioni M, Nair RR, Rablen S, et al. Limits on intrinsic magnetism in graphene. Phys Rev Lett. 2010;105:207205.
- Liu Y, Tang N, Wan X, et al. Realization of ferromagnetic graphene oxide with high magnetization by doping graphene oxide with nitrogen. Sci Rep. 2013;3:2566.
- Li L, Qin R, Li H, et al. Functionalized graphene for high-performance two-dimensional spintronics devices. ACS Nano. 2011;5:2601.
- Hill EW, Geim A, Novoselov K, et al. Graphene spin valve devices. IEEE Trans Magn. 2006;42:2694.
- Kim WY, Kim KS. Prediction of very large values of magnetoresistance in a graphene nanoribbon device. Nat Nanotechnol. 2008;3:408.
- Silva PV, Saraiva-Souza A, Maia DW, et al. High efficiency spin-valve and spin-filter in a doped rhombic graphene quantum dot device. J Magn Magn Mater. 2018;451:532.
- Hod O, Barone V, Scuseria GE. Half-metallic graphene nanodots. Phys Rev B. 2008;77:035411.
- Ahin H, Senger RT, Ciraci S. Spintronic properties of zigzag-edged triangular graphene flakes. J Appl Phys. 2010;108:074301.
- Fernandez-Rossier J, Palacios JJ. Magnetism in graphene nanoislands. Phys Rev Lett. 2007;99:177204.
- Wang WL, Meng S, Kaxiras E. Graphene nanoflakes with large spin. Nano Lett. 2008;8:241.
- Abdelsalam H, Saroka VA, Ali M, et al. Stability and electronic properties of edge functionalized silicene quantum dots: a first principles study. Phys E Low Dimens Syst Nanostruct. 2019;108:339.
- Wang J, Deng S, Liu Z, et al. The rare two-dimensional materials with dirac cones. Natl Sci Rev. 2015;2:22.
- Viana GED, Silva AM, Barros da FUC, et al. Thermal stability and electronic properties of boron nitride nanoflakes. J Mol Model. 2020;26:100.
- Lieb EH. Two theorems on the Hubbard model. Phys Rev Lett. 1989;62:1201.
- Pavliček N, Mistry A, Majzik Z, et al. Synthesis and characterization of triangulene. Nat Nanotechnol. 2017;12:308.
- Li J, Sanz S, Castro-Esteban J, et al. Uncovering the triplet ground state of triangular graphene nanoflakes engineered with atomic precision on a metal surface. Phys Rev Lett. 2020;124:177201.
- Su J, Telychko M, Song S, et al. Triangulene series: from precursor design towards on-surface synthesis and characterization. Angew Chem. 2020;59:7658.
- Liu X, Qin X, Li X, et al. Designing two-dimensional versatile room-temperature ferromagnets via assembling large-scale magnetic quantum dots. Nano Lett. 2021;21:9816.
- Liu SS, Yuan X, Zou Y, et al. Wafer-scale two-dimensional ferromagnetic Fe3GeTe2 thin films were grown by molecular beam epitaxy. npj 2D Mater Appl. 2017;1:1.
- Huang B, Clark G, Navarro-Moratalla E, et al. Layer-dependent ferromagnetism in a van der Waals crystal down to the monolayer limit. Nature. 2017;546:270.
- Bonilla M, Kolekar S, Ma Y, et al. Strong room-temperature ferromagnetism in VSe2 monolayers on van der Waals substrates. Nat Nanotechnol. 2018;13:289.
- Kim K, Lim SY, Kim J, et al. Antiferromagnetic ordering in van der Waals two-dimensional magnetic material MnPS3 probed by Raman spectroscopy. 2D Mater. 2019;6:041001.
- Sears JA, Chern LE, Kim S, et al. Ferromagnetic kitaev interaction and the origin of large magnetic anisotropy in α-RuCl3. Nat Phys. 2020;16:837.
- Burch KS, Mandrus D, Park J-G. Magnetism in two-dimensional van der Waals materials. Nature. 2018;563:47.
- Gibertini M, Koperski M, Morpurgo AF, et al. Magnetic 2D materials and heterostructures. Nat Nanotechnol. 2019;14:408.
- Mak KF, Shan J, Ralph DC. Probing and controlling magnetic states in 2D layered magnetic materials. Nat Rev Phys. 2019;1:646.
- Jiang X, Liu Q, Xing J, et al. Recent progress on 2D magnets: fundamental mechanism, structural design and modification featured, Appl. Phys Rev. 2021;8:031305.
- Ling-fei C, Danb X, Ming-xing G, et al. Size and shape effects on curie temperature of ferromagnetic nanoparticles. Trans Nonferrous Met Soc China. 2007;17:1451.
- Lopez-Dominguez VR, Hernàndez JM, Tejada J, et al. Colossal reduction in curie temperature due to finite-size effects in CoFe2O4 nanoparticles. Chem Mater. 2013;25:6.
- Chen C, Chen X, Wu C, et al. Air-Stable 2D Cr5Te8 nanosheets with thickness-tunable ferromagnetism. Adv.Mater. 2022;34:2107512.
- Meng L, Zhou Z, Xu M, et al. Anomalous thickness dependence of curie temperature in air-stable two-dimensional ferromagnetic 1T-CrTe2 grown by chemical vapor deposition. Nat Commun. 2021;12. DOI:10.1038/s41467-021-21072-z.
- Zhang BY, Liu T, Meng B, et al. Broadband high photoresponse from pure monolayer graphene photodetector. Nat Commun. 2013;4:1811.
- Tang L, Ji R, Li X, et al. Deep ultraviolet to near-infrared emission and photoresponse in layered N-doped graphene quantum dots. ACS Nano. 2014;8:6312.
- Li R, Zhang L, Shi L, et al. MXene Ti3C2: an effective 2D light-to-heat conversion material. ACS Nano. 2017;11:3752.
- Yang S, Niu W, Wang A-L, et al. Ultrathin two-dimensional organic–inorganic hybrid perovskite nanosheets with bright, tunable photoluminescence and high stab ty. Angew Chem Int Ed. 2017;56:4252.
- Cao Y, Dong H, Pu S, et al. Photoluminescent two-dimensional SiC quantum dots for cellular imaging and transport. Nano Res. 2018;11:4074.
- Zhou Y, Xu Q, Ge T, et al. Accurate control of VS2 nanosheets for coexisting high photoluminescence and photothermal conversion efficiency. Angew Chem Int Ed. 2020;59:3322.
- Wang L, Wang Y, Xu T, et al. Gram-scale synthesis of single-crystalline graphene quantum dots with superior optical properties. Nat Commun. 2014;5:5357.
- Bertel R, Mora-Ramos ME, Correa JD. Electronic properties and optical response of triangular and hexagonal MoS2 quantum dots. A DFT approach. Phys E. 2019;109:201.
- Ding Y, He P, Li S, et al. Efficient full-color boron nitride quantum dots for thermostable flexible displays. ACS Nano. 2021;15:14610.
- Chen S, Ullah N, Wang T, et al. Tuning the optical properties of graphene quantum dots by selective oxidation: a theoretical perspective. J Mater Chem C. 2018;6:6875.
- Ding Y, He P, Li S, et al. Efficient full-color boron nitride quantum dots for thermostable flexible displays. ACS Nano. 2021; DOI:10.1021/acsnano.1c04321.
- Feng J, Dong H, Pang B, et al. Tuning the electronic and optical properties of graphene quantum dots by selective boronization. J Mater Chem C. 2019;7:237.
- Abdelsalam H, Saroka VA, Atta MM, et al. Tunable electro-optical properties of doped chiral graphene nanoribbons. Chem Phys. 2021;544:111116.
- Kim S, Hwang SW, Kim M-K, et al. Anomalous behaviors of visible luminescence from graphene quantum dots: interplay between size and shape. ACS Nano. 2012;6:8203.
- Liu Z, Li F, Luo Y, et al. Size effect of graphene quantum dots on photoluminescence. Molecules. 2021;26:3922.
- Abdelsalam H, Talaat MH, Lukyanchuk I, et al. Electro-absorption of silicene and bilayer graphene quantum dots. J Appl Phys. 2016;120:014304.
- Kalita D, Deuri JK, Sahu P, et al. Plasmonic nanostructure integrated two-dimensional materials for optoelectronic devices. J Phys D: Appl Phys. 2022;55:243001.
- Wang W, Apell P, Kinaret J. Edge plasmons in graphene nanostructures. Phys Rev B. 2011;84:085423.
- Marco Gibertini NM. Emergence of one-dimensional wires of free carriers in transition-metal-dichalcogenide nanostructures. Nano Lett. 2015;15:6229.
- Neupane GP, Wang B, Tebyetekerwa M, et al. Highly enhanced light–matter interaction in mxene quantum dots–monolayer WS2 heterostructure. Small. 2021;17:2006309.
- Zeng Z, Yan Y, Chen J, et al. Boosting the photocatalytic ability of cu2o nanowires for CO2 conversion by MXene quantum dots. Adv Funct Mater. 2019;29:180650.
- Mohanty B, Giri L, Jena BK. MXene-Derived quantum dots for energy conversion and storage applications. Energy Fuels. 2021;35:14304–14324.
- Yu X, Cai X, Cui H, et al. Fluorine-free preparation of titanium carbide MXene quantum dots with high near-infrared photothermal performances for cancer therapy. Nanoscale. 2017;9:17859. DOI:10.1039/C7NR05997C
- Xuan J, Wang Z, Chen Y, et al. Organic-base-driven intercalation and delamination for the production of functionalized titanium carbide nanosheets with superior photothermal therapeutic performance. Angew Chem Int Ed. 2016;55:14569–14574.
- Chou SS, Kaehr B, Kim J, et al. Chemically exfoliated mos2 as near-infrared photothermal agents. Chem Int Ed. 2013;52:4160.
- Robinson JT, Tabakman SM, Liang Y, et al. Ultrasmall reduced graphene oxide with high near-infrared absorbance for photothermal therapy. J Am Chem Soc. 2011;133:6825.
- Huang H, Feng W, Chen Y. Two-dimensional biomaterials: material science, biological effect and biomedical engineering applications. Chem Soc Rev. 2021. DOI:10.1039/D0CS01138J
- Deng D, Novoselov KS, Fu Q, et al. Catalysis with two-dimensional materials and their heterostructures. Nat Nanotechnol. 2016;11:218.
- Tan C, Luo Z, Chaturvedi A, et al. Preparation of high-percentage 1T-phase transition metal dichalcogenide nanodots for electrochemical hydrogen evolution. Adv Mater. 2018;30:1705509.
- Nakada K, Fujita M, Dresselhaus G, et al. Edge state in graphene ribbons: nanometer size effect and edge shape dependence. Phys Rev B. 1996;54:17954.
- Jaramillo TF, Jørgensen KP, Bonde J, et al. Identification of active edge sites for electrochemical H2 evolution from MoS2 nanocatalysts. Science. 2007;317:100.
- Girit CO, Meyer JC, Erni R, et al. Graphene at the edge: stability and dynamics. Science. 2009;323:1705.
- Abdelsalam H, Saroka VA, Teleb NH, et al. Electronic and adsorption properties of extended chevron and cove-edged graphene nanoribbons. Phys E Low Dimens Syst Nanostruct. 2021;126:114438.
- Zhao X, Ma X, Sun J, et al. Enhanced catalytic activities of surfactant-assisted exfoliated WS2 nanodots for hydrogen evolution. ACS Nano. 2016;10:2159.
- Chen Z, Li Y, Wang K, et al. Scalable production of intrinsic WX2 (X = S, Se, Te) quantum sheets for efficient hydrogen evolution electrocatalysis. Nanotechnology. 2021;32:495701.
- Wang C, Jin M, Liu D, et al. VSe2 quantum dots with high-density active edges for flexible efficient hydrogen evolution reaction. J Phys D. 2021;54:214006.
- Chae HK, Siberio-Pérez DY, Kim J, et al. A route to high surface area, porosity and inclusion of large molecules in crystals. Nature. 2004;427:523.
- Ou G, Fan P, Ke X, et al. Defective molybdenum sulfide quantum dots as highly active hydrogen evolution electrocatalysts. Nano Res. 2018;11:751.
- Li Y, Zhao Y, Cheng H, et al. Nitrogen-doped graphene quantum dots with oxygen-rich functional groups. J Am Chem Soc. 2012;134:15.
- Gong KP, Du F, Xia ZH, et al. Nitrogen-doped carbon nanotube arrays with high electrocatalytic activity for oxygen reduction. Science. 2009;323:760.
- Wang H, Maiyalagan T, Wang X. Review on recent progress in nitrogen-doped graphene: synthesis, characterization, and its potential applications. ACS Catal. 2012;2:781.
- Yu L, Pan X, Cao X, et al. Oxygen reduction reaction mechanism on nitrogen-doped graphene: a density functional theory study. J Catal. 2011;282:183–190.
- Brownson DAC, Munro LJ, Kampouris DK, et al. Electrochemistry of graphene: not such a beneficial electrode material? RSC Adv. 2011;1:978.
- Mazánek V, Luxa J, Matĕjková S, et al. Ultrapure Graphene is a poor electrocatalyst: definitive proof of the key role of metallic impurities in graphene based electrocatalysis. ACS Nano. 2019;13.
- Wang X, Sun G, Routh P, et al. Heteroatom-doped graphene materials: syntheses, properties and applications. Chem Soc Rev. 2014;43:7067.
- Zhang X, Chen A, Chen L, et al. 2D materials bridging experiments and computations for electro/photocatalysis. Adv Energy Mater. 2021;2003841.
- Zheng Y, Jiao Y, Zhu Y, et al. Hydrogen evolution by a metal-free electrocatalyst. Nat Commun. 2014;5:3783.
- Feng C, Tang L, Deng Y, et al. Enhancing optical absorption and charge transfer: synthesis of S-doped h-BN with tunable band structures for metal-free visible-light-driven photocatalysis. Appl Catal B. 2019;256:117827.
- Dai L, Xue Y, Qu L, et al. Metal-free catalysts for oxygen reduction reaction. Chem Rev. 2015;115:4823.
- Liu X, Dai L. Carbon-based metal-free catalysts. Nat Rev Mater. 2016;1:16064.
- Zhang P, Xub B, Chen G, et al. Large-scale synthesis of nitrogen doped MoS2 quantum dots for efficient hydrogen evolution reaction. Electrochim Acta. 2018;270:256.
- Fu Q, Han J, Wang X, et al. 2D transition metal dichalcogenides: design, modulation, and challenges in electrocatalysis. Adv Mater. 2021;33:1907818. DOI:10.1002/adma.201907818
- Jiao Y, Zheng Y, Davey K, et al. Activity origin and catalyst design principles for electrocatalytic hydrogen evolution on heteroatom-doped graphene. Nat Energy. 2016;1:16130.
- Deng J, Li H, Xiao J, et al. Triggering the electrocatalytic hydrogen evolution activity of the inert two-dimensional MoS2 surface via single-atom metal doping. Energy Environ Sci. 2015;8:1594–1601.
- Zhang P, Xu B, Chen G, et al. Large-scale synthesis of nitrogen doped MoS2 quantum dots for efficient hydrogen evolution reaction. Electrochim Acta. 2018;270:256.
- Gao GP, P.O’Mullane A, Du AJ. 2D MXenes: a new family of promising catalysts for the hydrogen evolution reaction. ACS Catal. 2017;7:494.
- Gao Q, Zhang W, Shi Z, et al. Structural design and electronic modulation of transition-metal-carbide electrocatalysts toward efficient hydrogen evolution. Adv Mater. 2019;31:1802880.
- Zhu J, Hu L, Zhao P, et al. Recent advances in electrocatalytic hydrogen evolution using nanoparticles. Chem Rev. 2020;120:851.
- Ito Y, Cong W, Fujita T, et al. High catalytic activity of nitrogen and sulfur co-doped nanoporous graphene in the hydrogen evolution reaction. Angew Chem Int Ed. 2015;54:2131–2136.
- Tabish TA, Scotton CJ, J Ferguson DC, et al. Biocompatibility and toxicity of graphene quantum dots for potential application in photodynamic therapy. Nanomedicine (Lond). 2018;13:1923–1937.
- Chen L, Zhang X, Zhao Z, et al. Controllable preparation of boron nitride quantum dots with small size and strong blue photoluminescence. Colloids Surf A. 2021;614:126181.
- Zhan Y, Liu Z, Liu Q, et al. A facile and one-pot synthesis of fluorescent graphitic carbon nitride quantum dots for bio-imaging applications. New J Chem. 2017;41:3930.
- Tao W, Ji X, Xu X, et al. Antimonene quantum dots: synthesis and application as near-infrared photothermal agents for effective cancer therapy. Angew Chem. 2017;129:12058.
- Shang CDA, Shang M, Ma X, et al. Water-soluble VS2 quantum dots with unusual fluorescence for biosensing. Sens Actuators B Chem. 2018;255:926.
- Yang G, Zhao J, Yi S, et al. Biodegradable and photostable Nb2C MXene quantum dots as promising nanofluorophores for metal ions sensing and fluorescence imaging. sens Actuators B Chem. 2020;309:127735.
- Iannazzo D, Ziccarelli I, Pistone A. Graphene quantum dots: multifunctional nanoplatforms for anticancer therapy. J Mater Chem B. 2017;5:6471.
- Qian Z, Ma J, Shan X, et al. Surface functionalization of graphene quantum dots with small organic molecules from photoluminescence modulation to bioimaging applications: an experimental and theoretical investigation. RSC Adv. 2013;3:14571.
- Iannazzo D, Pistone A, Salamò M, et al. Graphene quantum dots for cancer targeted drug delivery. Int J Pharm. 2017;518:185.
- Zhao M, Chen A, Huang D, et al. MoS2 quantum dots as new electrochemiluminescence emitters for ultrasensitive bioanalysis of lipopolysaccharide. Anal Chem. 2017;89:8335.
- Guo Y, Li J. MoS2 quantum dots: synthesis, properties and biological applications. Mater Sci Eng C. 2020;109:110511.
- Liu T, Wang C, Gu X, et al. Drug delivery with pegylated mos2 nano-sheets for combined photothermal and chemotherapy of cancer. Adv Mater. 2014;26:3433.
- Liu Y, Duan X, Shin H-J, et al. Promises and prospects of two-dimensional transistors. Nature. 2021;591:43.
- Stampfer C, Schurtenberger E, Molitor F, et al. Tunable graphene single electron transistor. Appl Phys Lett. 2008;92:012102.
- Westervelt RM. Graphene nanoelectronics. Science. 2008;320:324.
- Ray SJ. First-principles study of MoS2, phosphorene and graphene based single electron transistor for gas sensing applications. Sens Actuators B Chem. 2016;222:492.
- Konstantatos G, Badioli M, Gaudreau L, et al. Hybrid graphene–quantum dot phototransistors with ultrahigh gain. Nat Nanotechnol. 2012;7:363.
- Chen C, Qiao H, Lin S, et al. Highly responsive MoS2 photodetectors enhanced by graphene quantum dots. Sci Rep. 2015;5:11830.
- Huo N, Konstantatos G. Recent progress and future prospects of 2D-based photodetectors. Adv Mater. 2018;30:1801164.
- Seo J, Lee JH, Pak J, et al. Ultrasensitive photodetection in MoS2 avalanche phototransistors. Adv Sci. 2021;8:2102437.
- Sun Q, Wang YA, Li LS, et al. Bright, multicoloured light-emitting diodes based on quantum dots. Nat Photonics. 2007;1:717.
- Ross JS, Klement P, Jones AM, et al. Electrically tunable excitonic light-emitting diodes based on monolayer WSe2 p–n junctions. Nat Nanotechnol. 2014;9:268.
- Luo Z, Qi G, Chen K, et al. Microwave-assisted preparation of white fluorescent graphene quantum dots as a novel phosphor for enhanced white-light-emitting diodes. Adv Funct Mater. 2016;26:2739.
- Jin H, Baek B, Kim D, et al. Effects of direct solvent-quantum dot interaction on the optical properties of colloidal monolayer WS2 quantum dots. Nano Lett. 2017;17:7471.
- Luo Z, Qi G, Chen K, et al. Microwave-assisted preparation of white fluorescent graphene quantum dots as a novel phosphor for enhanced white-light-emitting diodes. Adv Funct Mater. 2016;26:2739.
- Nguyen TP, Sohn W, Oh JH, et al. Size-dependent properties of two-dimensional MoS2 and WS2. J Phys Chem C. 2016;120:10078.
- Xu Q, Yang W, Wen Y, et al. Hydrochromic full-color MXene quantum dots through hydrogen bonding toward ultrahigh-efficiency white light-emitting diodes. Appl Mater Today. 2019;16:90.
- Zhang X, Xie H, Liu Z, et al. Black phosphorus quantum dots. Angew Chem Int Ed. 2015;54:3653.
- Han ST, Hu L, Wang X, et al. Black phosphorus quantum dots with tunable memory properties and multilevel resistive switching characteristics. Adv Sci. 2017;4:1600435.
- Wang D, Ji F, Chen X, et al. Quantum conductance in MoS2 quantum dots-based nonvolatile resistive memory device. Appl Phys Lett. 2017;110:093501.
- An H, Lee YH, Lee JH, et al. Highly stable and flexible memristive devices based on polyvinylpyrrolidone: WS2 quantum dots. Sci Rep. 2020;10:5793.
- Joo SS, Kim J, Seok Kang S, et al. Graphene-quantum-dot nonvolatile charge-trap flash memories. Nanotechnology. 2014;25:255203.
- Wolf SA, Awschalom DD, Buhrman RA, et al. Spintronics: a spin-based electronics vision for the future. Science. 2001;294. DOI:10.1126/science.1065389.
- Ahn EC. 2D materials for spintronic devices. Npj 2D Mater Appl. 2020;4:17.
- Pournaghavi N, Esmaeilzadeh M, Abrishamifar A, et al. Extrinsic Rashba spin–orbit coupling effect on silicene spin polarized field effect transistors. J Phys Condens Matter. 2017;29:145501.
- Yan W, Txoperena O, Llopis R, et al. A two-dimensional spin field-effect switch. Nat Commun. 2016;7:13372.
- Zhang L, Zhou JN, Li H, et al. Recent progress and challenges in magnetic tunnel junctions with 2D materials for spintronic applications. Appl Phys Rev. 2021;8:021308.
- Xiao D, Liu G-B, Feng W, et al. Coupled spin and valley physics in monolayers of mos2 and other group-VI dichalcogenides. Phys Rev Lett. 2012;108:196802.
- Lin L, Xu Y, Zhang S, et al. Fabrication of luminescent monolayered tungsten dichalcogenides quantum dots with giant spin-valley coupling. ACS Nano. 2013;7:8214.
- Zhang K, Fu L, Zhang W, et al. Ultrasmall and monolayered tungsten dichalcogenide quantum dots with giant spin–valley coupling and purple luminescence. ACS Omega. 2018;3:12188.
- Loss D, DiVincenzo DP. Quantum computation with quantum dots. Phys Rev A. 1998;57:120.
- Cerletti V, Coish WA, Gywat O, et al. Recipes for spin-based quantum computing. Nanotechnology. 2005;16:R27.
- Trauzettel B, Bulaev DV, Loss D, et al. Spin qubits in graphene quantum dots. Nature Phys. 2007;3:192.
- Trauzettel B, Borhani M, Trif M, et al. Theory of spin qubits in nanostructures. J Phys Soc Jpn. 2008;77:031012.
- Pawłowski J, Z˙ebrowski D, Bednarek S. Valley qubit in a gated MoS2 monolayer quantum dot. Phys Rev B. 2018;97:155412.
- Liu X, Hersam MC. 2D materials for quantum information science. Nat Rev Mater. 2019;4:669.
- Kou L, Huang T, Zheng B, et al. coaxial wet-spun yarn supercapacitors for high-energy density and safe wearable electronics. Nat Commun. 2014;5:3754.
- Shao Y, El-Kady MF, Wang LJ, et al. Graphene-based materials for flexible supercapacitors. Chem Soc Rev. 2015;44:3639.
- Wang Y, Chen F, Liu Z. A Highly elastic and reversibly stretchable all-polymer supercapacitor, ngew. Chem Int Ed. 2019;58:15707.
- Liu W, Li M, Jiang G, et al. Graphene quantum dots-based advanced electrode materials: design, synthesis and their applications in electrochemical energy storage and electrocatalysis. Adv Energy Mater. 2020;10:2001275.
- Liu W, Zhang M, Li M, et al. Advanced electrode materials comprising of structure-engineered quantum dots for high-performance asymmetric micro-supercapacitors. Adv Energy Mater. 2020;10.
- Zhou X, Qin Y, He X, et al. Ti3C2Tx nanosheets/ Ti3C2Tx quantum dots/RGO (reduced graphene oxide) fibers for an all-solid-state asymmetric supercapacitor with high volume energy density and good flexibility. ACS Appl Mater Interfaces. 2020;12:10.
- Simon P, Gogotsi Y, Dunn B. Where do batteries end and supercapacitors begin. Science. 2014;343:1210.
- Horn M, MacLeod J, Liu M, et al. Supercapacitors: a new source of power for electric cars? Econ Anal Policy. 2019;61:93.
- Simon P, Gogotsi Y. Perspectives for electrochemical capacitors and related devices. Nat Mater. 2020;19:1151.
- Nakhanivej P, Dou Q, Xiong P, et al. Two-dimensional pseudocapacitive nanomaterials for high-energy- and high-power-oriented applications of supercapacitors, acc. Mater Res. 2021;2:86.
- Pan Z, Zhi H, Qiu Y, et al. Achieving commercial-level mass loading in ternary-doped holey graphene hydrogel electrodes for ultrahigh energy density supercapacitors. Nano Energy. 2018;46:266.
- Tsai ML, Wei W-R, Tang L, et al. Si hybrid solar cells with 13% efficiency via concurrent improvement in optical and electrical properties by employing graphene quantum dots. ACS Nano. 2016;10:815.
- Wang YM, Ding K, Sun BQ, et al. Two-dimensional layered material/silicon heterojunctions for energy and optoelectronic applications. Nano Res. 2016;9:72.
- Hu W, Lin L, Yang C, et al. Edge-modified phosphorene nanoflake heterojunctions as highly efficient solar cells. Compr Physiol. 2016;6:1675.
- Chen W, Li K, Wang Y. Black phosphorus quantum dots for hole extraction of typical planar hybrid perovskite solar cells. J Phys Chem Lett. 2017;8:591.
- Bernardi M, Palummo M, Grossman JC. Extraordinary sunlight absorption and one nanometer thick photovoltaics using two-dimensional monolayer materials. Nano Lett. 2013;13:3664.
- Amani M, Lien D-H, Kiriya D, et al. Near-unity photoluminescence quantum yield in MoS2. Science. 2015;350:1065–1068.
- Tetsuka H, Nagoya A, Fukusumi T, et al. Molecularly designed, nitrogen-functionalized graphene quantum dots for optoelectronic devices. Adv Mater. 2016;28:4632.
- Jariwala D, Davoyan AR, Wong J, et al. Van der Waals materials for atomically-thin photovoltaics: promise and outlook. ACS Photonics. 2017;4:2962.
- Das S, Pandey D, Thomas J, et al. The role of graphene and other 2D materials in solar photovoltaics. Adv Mater. 2019;31:1802722.
- Chen M, Wang J, Yin F, et al. Strategically integrating quantum dots into organic and perovskite solar cells. J Mater Chem A. 2021;9:4505.
- Marinova N, Valero S, Delgado JL. Organic and perovskite solar cells: working principles. Mater Interf J Coll Interf Sci. 2017;488:373.
- Park N-G. Perovskite solar cells: an emerging photovoltaic technology. Mater Today. 2015;18:65.
- Chen W, Li K, Wang Y, et al. Black phosphorus quantum dots for hole extraction of typical planar hybrid perovskite solar cells. J Phys Chem Lett. 2017;8:591.
- Kim JK, Park MJ, Kim SJ, et al. Balancing light absorptivity and carrier conductivity of graphene quantum dots for high-efficiency bulk heterojunction solar cells. ACS Nano. 2013;7:7207.
- Le QV, Nguyen TP, Choi KS, et al. Dual use of tantalum disulfides as hole and electron extraction layers in organic photovoltaic cell. Phys Chem Chem Phys. 2014;16:25468.
- Ibrahem MA, Lan T-W, Huang JK, et al. High quantity and quality few-layers transition metal disulfide nanosheets from wet-milling exfoliation. RSC Adv. 2013;3:13193.
- Park KH, Jung S, Kim J, et al. Boosting photovoltaic performance in organic solar cells by manipulating the size of MoS2 quantum dots as a hole-transport material. Nanomaterials. 2021;11:1464.
- Dai J, Zeng XC. Bilayer phosphorene: effect of stacking order on band gap and its potential applications in thin-film solar cells. J Phys Chem Lett. 2014;5:1289.
- Huo N, Kang J, Wei Z, et al. Novel and enhanced optoelectronic performances of multilayer MoS2-WS2 heterostructure transistors. Adv Funct Mater. 2014;24:7025.
- Wu K, Ma H, Gao Y, et al. Highly-efficient heterojunction solar cells based on two-dimensional tellurene and transition metal dichalcogenides. J Mater Chem A. 2019;7:7430. DOI:10.1039/C9TA00280D
- Abdelsalam H, Atta MM, Osman W, et al. Two-dimensional quantum dots for highly efficient heterojunction solar cells. J Colloid Interface Sci. 2021;603:48.
- Suen NT, Hung S-F, Quan Q, et al. Electrocatalysis for the oxygen evolution reaction: recent development and future perspectives. Chem Soc Rev. 2017;46:337.
- Tahir M, Pan L, Idrees F, et al. Electrocatalytic oxygen evolution reaction for energy conversion and storage: a comprehensive review. Nano Energy. 2017;37:136–157.
- Pan J, Tian XL, Zaman S, et al. Recent progress on transition metal oxides as bifunctional catalysts for lithium-air and zinc-air batteries, batter. Supercaps. 2018;2:336.
- Mohanty B, Ghorbani-Asl M, Kretschmer S, et al. MoS2 quantum dots as efficient catalyst materials for the oxygen evolution reaction. ACS Catal. 2018;8:1683.
- Li X, Duan X, Han C, et al. Chemical activation of nitrogen and sulfur co-doped graphene as defect-rich carbocatalyst for electrochemical water splitting. Carbon. 2019;148:540.
- Wang P, Zhao D, Hui X, et al. Bifunctional catalytic activity guided by rich crystal defects in Ti3C2 MXene quantum dot clusters for Li–O2 batteries. Adv Energy Mater. 2021;11:2003069.
- Zhu D, Xie Y, Liu T. Exploring the synergy of 2D MXene-supported black phosphorus quantum dots in hydrogen and oxygen evolution reactions. J Mater Chem A. 2018;6:21255.
- Remanan S, Padmavathy N, Ghosh S, et al. Porous graphene-based membranes: preparation and properties of a unique two-dimensional nanomaterial membrane for water purification. Sep Purif Rev. 2021;50:262.
- Ihsanullah I. Boron nitride-based materials for water purification: progress and outlook. Chemosphere. 2021;263:127970.
- Cui Y, An X, Zhang S, et al. Emerging graphitic carbon nitride-based membranes for water purification. Water Res. 2021;200:117207.
- Zhang S, Liao S, Qi F, et al. Direct deposition of two-dimensional MXene nanosheets on commercially available filter for fast and efficient dye removal. J Hazard Mater. 2020;384:121367.
- Liu Y, Zhao Y, Zhang X, et al. MoS2-based membranes in water treatment and purification. Chem Eng J. 2021;422:130082.
- Goh PS, Ismail AF. Graphene-based nanomaterial: the state-of-the-art material for cutting edge desalination technology. Desalination. 2015;356:115–128.
- Mi B. Scaling up nanoporous graphene membranes. Science. 2019;364:1033–1034.
- Xu G-R, Feng HJ, Das R, et al. Two-dimensional (2D) nanoporous membranes with sub-nanopores in reverse osmosis desalination: latest developments and future directions. Desalination. 2019;451:18.
- Abdelsalam H, Teleb NH, Yahia IS, et al. First principles study of the adsorption of hydrated heavy metals on graphene quantum dots. J Phys Chem Solids. 2019;130:32.
- Jacobse PH, McCurdy RD, Jiang J, et al. Bottom-up assembly of nanoporous graphene with emergent electronic states. J Am Chem Soc. 2020;142:13507.
- Wang D, Lu X, Yang M, et al. On-surface synthesis of variable bandgap nanoporous graphene, ChemRxiv. Cambridge: Cambridge Open Engage; 2021.
- Nair R, Wu HA, Jayaram PN, et al. Unimpeded permeation of water through helium-leak–tight graphene-based membranes. Science. 2012;335:442.
- Sun H, Wu L, Wei W, et al. Recent advances in graphene quantum dots for sensing. Mater Today. 2013;16:433.
- Musselman KP, Ibrahim KH, Yavuz M. Research update: beyond graphene—synthesis of functionalized quantum dots of 2D materials and their applications editors-pick. APL Mater. 2018;6:120701.
- Kalkal A, Kadian S, Pradhan R, et al. Recent advances in graphene quantum dot-based optical and electrochemical (bio)analytical sensor. Mater Adv. 2021;2:5513.
- Rohaizad NA, Mayorga-Martinez CC, Fojtů M, et al. Two-dimensional materials in biomedical, biosensing and sensing applications. Chem Soc Rev. 2021;50:619.
- Zhang KY, Lo KK-W. 8.18 - Chemosensing and diagnostics. Reedijk J, Poeppelmeier K. editors, Comprehensive Inorganic chemistry II Second, Elsevier. 2013. 657–732. DOI:10.1016/B978-0-08-097774-4.00804-4
- Mansuriya BD, Altintas Z. Applications of graphene quantum dots in biomedical sensors. Sensors. 2020;20:1072.
- Wang D, Wang L, Dong X, et al. Chemically tailoring graphene oxides into fluorescent nanosheets for Fe3+ ion detection. Carbon. 2012;50:2147.
- Dong Y, Li G, Zhou N, et al. Graphene quantum dot as a green and facile sensor for free chlorine in drinking water. Anal Chem. 2012;84:8378.
- Chakraborti H, Sinha S, Ghosh S, et al. Interfacing water soluble nanomaterials with fluorescence chemosensing: graphene quantum dot to detect Hg2+ in 100% aqueous solution. Mater Lett. 2013;97:78.
- Ou JZ, Chrimes AF, Wang Y, et al. Ion-driven photoluminescence modulation of quasi-two-dimensional MoS 2 nanoflakes for applications in biological systems. Nano Lett. 2014;14:857.
- Huo B, Liu B, Chen T, et al. One-step synthesis of fluorescent boron nitride quantum dots via a hydrothermal strategy using melamine as nitrogen source for the detection of ferric ions. Langmuir. 2017;33:10673.
- Yang G, Zhao J, Yi S, et al. Biodegradable and photostable Nb2C MXene quantum dots as promising nanofluorophores for metal ions sensing and fluorescence imaging. Sens Actuators B. 2020;309:127735.
- Schedin F, Geim AK, Morozov SV, et al. Detection of individual gas molecules adsorbed on graphene. Nat Mater. 2007;6:652.
- Yue N, Weicheng J, Rongguo W, et al. Hybrid nanostructures combining graphene–MoS2 quantum dots for gas sensing. J Mater Chem A. 2016;4:8198.
- Arunragsa S, Seekaew Y, Pon-On W, et al. Hydroxyl edge-functionalized graphene quantum dots for gas-sensing applications. Diamond Related Mater. 2020;105:107790.
- Yadav S, Chaudhary P, Uttam KN, et al. Facile synthesis of molybdenum disulfide (MoS2) quantum dots and its application in humidity sensing. Nanotechnology. 2019;30:295501.
- Bhati VS, Kumar M, Banerjee R. Gas sensing performance of 2D nanomaterials/metal oxide nanocomposites: a review. J Mater Chem C. 2021;9:8776.
- Liuab -J-J, Chen Z-T, Tang D-S, et al. Graphene quantum dots-based fluorescent probe for turn-on sensing of ascorbic acid. Sens Actuators B. 2015;212:214.
- Huang H, Feng Z, Li Y, et al. Highly sensitive detection of bisphenol A in food packaging based on graphene quantum dots and peroxidase. Anal Methods. 2015;7:2928.
- Wang Y, Ni Y. Molybdenum disulfide quantum dots as a photoluminescence sensing platform for 2,4,6-trinitrophenol detection. Anal Chem. 2014;86:7463.
- Wang X, Wu Q, Jiang K, et al. One-step synthesis of water-soluble and highly fluorescent MoS2 quantum dots for detection of hydrogen peroxide and glucose. Sens Actuators B Chem. 2017;252:183.
- Yan F, Sun J, Zang Y, et al. Solvothermal synthesis of nitrogen-doped MXene quantum dots for the detection of alizarin red based on inner filter effect. Dyes Pigm. 2021;195:109720.
- Faridbod F, Sanati AL. Graphene quantum dots in electrochemical sensors/biosensors. Curr Anal Chem. 2019;15:103.
- Aswathia R, Sandhya KY. Ultrasensitive and selective electrochemical sensing of Hg(ii) ions in normal and sea water using solvent exfoliated MoS2: affinity matters. J Mater Chem A. 2018;6:14602.
- Lin D, Su Z, Wei G. Three-dimensional porous reduced graphene oxide decorated with MoS2 quantum dots for electrochemical determination of hydrogen peroxide. Mater Today Chem. 2018;7:76e8377.
- Valappil MO, Mohamed J, Alwarappan S, et al. Electrochemical transformation of black phosphorous to phosphorene quantum dots: effect of nitrogen doping. Mater Res Express. 2020;7:014005.
- Savas S, Altintas Z. Graphene quantum dots as nanozymes for electrochemical sensing of Yersinia enterocolitica in milk and human serum. Materials. 2019;12:2189.
- Ge J, Hu Y, Deng R, et al. Highly sensitive microRNA detection by coupling nicking-enhanced rolling circle amplification with MoS2 quantum dots. Anal Chem. 2020;92:13588.
- Liu M, He Y, Zhou J, et al. A”naked-eye” colorimetric and ratiometric fluorescence probe for uric acid based on Ti3C2 MXene quantum dots. Anal Chim Acta. 2020;1103:134.
- Niu Y, Li J, Gao J, et al. Two-dimensional quantum dots for biological applications. Nano Res. 2021;14:3820.
- Zhu S, Zhang J, Qiao C, et al. Strongly green-photoluminescent graphene quantum dots for bioimaging applications. Chem Commun. 2011;47:6858.
- Li L, Wu G, Yang G, et al. Focusing on luminescent graphene quantum dots: current status and future perspectives. Nanoscale. 2013;5:4015.
- Zhang X, Xie X, Wang H, et al. Enhanced photoresponsive ultrathin graphitic-phase C3N4 nanosheets for bioimaging. J Am Chem Soc. 2013;135:18.
- Li Y, Liu Z, Hou Y, et al. Multifunctional nanoplatform based on black phosphorus quantum dots for bioimaging and photodynamic/photothermal synergistic cancer therapy. ACS Appl Mater Interfaces. 2017;9:30.
- Lin H, Wang C, Wu J, et al. Colloidal synthesis of MoS2 quantum dots: size-dependent tunable photoluminescence and bioimaging. New J Chem. 2015;39:8492.
- Huang M, Gu Z, Zhang J, et al. MXene and black phosphorus based 2D nanomaterials in bioimaging and biosensing: progress and perspectives. J Mater Chem B. 2021;9:5195.
- Pysz MA, Gambhir SS, Willmann JK. Molecular imaging: current status and emerging strategies. Clin Radiol. 2010;65:500.
- Shi M, Dong L, Zheng S, et al. “bottom-up” preparation of MoS2 quantum dots for tumor imaging and their in vivo behavior study biochem. Biophys Res Commun. 2019;516:1090–1096.
- Nurunnabi M, Khatun Z, Reeck GR, et al. Photoluminescent graphene nanoparticles for cancer phototherapy and imaging. ACS Appl Mater Interfaces. 2014;6:15.
- Wu X, Yang L, Luo L, et al. Engineered g-C3N4 quantum dots for tunable two-photon imaging and photodynamic therapy. ACS Appl Bio Mater. 2019;2:1998–2005.
- Liu M, Zhu H, Wang Y, et al. Functionalized MoS2 -based nanomaterials for cancer phototherapy and other biomedical applications. ACS Materials Lett. 2021;3:462.
- Shao J, Zhang J, Jiang C, et al. Biodegradable titanium nitride MXene quantum dots for cancer phototheranostics in NIR-I/II biowindows. Chem Eng Sci. 2020;400:126009.
- Cheng L, Wang C, Feng L, et al. Functional nanomaterials for phototherapies of cancer. Chem Rev. 2014;114:10869.
- Zhu H, Ni N, Govindarajan S, et al. Phototherapy with layered materials derived quantum dots. Nanoscale. 2020;12:43.
- Dai W, Dong H, Zhang X. A Semimetal-like molybdenum carbide quantum dots photoacoustic imaging and photothermal agent with high photothermal conversion efficiency. Materials. 2018;11:1776.