ABSTRACT
As a special class of stable atomic clusters, the superatom has become an exciting research topic in recent decades. They can mimic the chemistry and physics of individual atoms in the periodic table and find potential applications in a variety of fields. Traditional strategies for superatom design, however, have their own limitations. Herein, we review recent progress in the discovery of novel methodologies for superatom design, namely external-field regulated strategies (EFRS). We begin with a description of the basic concept of the superatom and the conventional electron-counting rules for superatom design, followed by a discussion of recent exploration about external-field regulated superatoms, where the oriented external electric field (OEEF), the ligand field, and the solvent field are presented. In the concluding section, we discuss the benefits and challenges of the EFRS together with some future research topics.
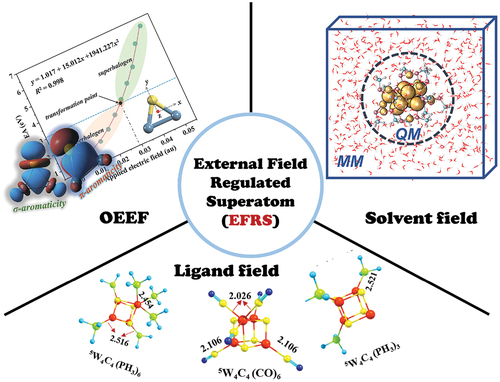
1. Introduction to superatoms
The past 40 years have witnessed the rapid development of cluster science in both the gas-phase and condensed-phase research about the atomically precise syntheses and potential applications [Citation1–22]. As a bridge between atoms and bulk materials, atomic clusters are special species consisting of several to hundreds of atoms, whose diverse properties and functionalities are found to be related with their adjustable composition, shape, size, and quantum confinement effect [Citation23–34]. This unique discovery has paved the way for the development of novel building blocks for the cluster assembly nanomaterials with tailored properties.
In this realm, a new era began when it was realized that some specific clusters featuring definite composition and size can mimic the physicochemical properties of individual elements in the periodic table. These clusters are termed as ‘superatoms’, which have potential in broadening the traditional two-dimensional (2D) periodic table to the three-dimensional (3D), replacing noble metals or significantly increasing the dimensionality of building blocks in the material design. In principle, the possibilities for designing superatoms are boundless, thereby rendering the 3D periodic table potentially infinite [Citation22]. Following the suggestion of the theoretical superatom concept by Khanna and Jena [Citation35] and the experimental discoveries by Castleman et al. [Citation36], different kinds of superatoms, including superhalogens, superalkalis, rare earth superatoms, magnetic superatoms, non-noble metal superatoms etc., have been successively proposed and identified. Al13 featuring the 1S21P61D102S21F142P5 Jellium shell is a typical and famous superatom. The electron affinity (EA) of Al13 (3.62 eV) [Citation37] is similar to that of the Cl atom, which can be considered as a superhalogen. Particularly, in the superatomic framework, superhalogen and superalkali are the two most explored categories owing to their clear and simple criteria. Superalkalis are clusters featuring lower adiabatic ionization potentials (AIPs) than alkali atoms (3.89–5.39 eV) [Citation38], while superhalogens represent a class of clusters whose adiabatic electron affinities (AEAs) are higher than those of halogen elements (3.06–3.62 eV) [Citation39] in the periodic table. These two kinds of superatoms have been the subject of extensive research over the past several decades, and the family of them has also been remarkably expanded due to their fundamental significance and potential applications as super-oxidizers and super-deoxidizers.
This concise review highlights recent advancements in the construction of superhalogens and superalkalis, with a specific focus on novel strategies known as external-field regulated strategies (EFRS). Initially, traditional methods for designing various superatoms are presented. Subsequently, we delved into the discussion of several external-field methodologies, including the oriented external electric field (OEEF), ligand field, and solvent field, which serve as innovative approaches. Finally, a brief summary is presented with perspectives on the advantages of these EFRS and future research interests.
2. Traditional and novel regulated strategies in designing superatoms
2.1 Traditional strategies in superatom design
Back in 1981, Gutsev and Boldyrev suggested a MXk + 1 formulation for constructing superhalogens, in which M is the metal atom, X is the halogen atom, and k is the highest valence of the metal atom [Citation40]. In this case, LiF2 serves as an exemplary illustration of a halogen atom. Building upon this, the same authors proposed that a cluster featuring one more electron than the shell closure configuration, e.g., Li3O, Na3O etc., exhibit characteristics akin to alkali atoms [Citation39]. Consequently, superhalogens and superalkalis can be regarded as superatoms, as they imitate the chemical behavior of halogen and alkali atoms, respectively. The explicit concept of superatoms was first proposed by Khanna and Jena in 1992 [Citation35], and since then, a significant body of research has been conducted to elucidate the fundamental science behind such a concept [Citation1,Citation36,Citation41–57]. Various traditional electron-counting rules (ECRs) have been attempted to design superatoms, including the Jellium rule, Wade-Mingos rule, octet shell closure rule, 18-electron rule and so on. Here, we will first briefly discuss these traditional rules in the superatom design.
2.1.1 The Jellium rule
In the Jellium rule, a metal cluster can be conceptualized as two interconnected subsystems: the valence electrons and a positively charged ionic core. In the ordinary Jellium model framework, the ionic structure of the cluster is substituted with a uniform and spherically symmetrical distribution of positive charge. The electronic states of metal clusters correspond to delocalized orbitals 1S21P61D102S21F14 … , and the clusters demonstrate enhanced stability when the number of the valence electrons corresponds to a filled electronic shell [Citation22]. The first metal cluster system that adheres to the Jellium rule is the Na clusters. In the Knight and co-workers’ experiments [Citation58], the clusters with 8, 20, 40, 58, … Na atoms exhibit stronger abundance in the mass spectrum, which are termed as ‘magic’ clusters. The enhanced stability of these magic numbers was attributed to the electronic shell closure in the spherical Jellium model. Another classical example, which conforms to the Jellium model, is the Al13¯ cluster featuring the 1S21P61D102S21F142P6 electronic shell. The increased stability of this star cluster was originally observed by Castleman et al. [Citation59]. Correspondingly, the neutral Al13 cluster with 39 valence electrons needs one more electron to fill its 2P subshell, just like a halogen atom needs one more electron to close its np5 atomic shell. Hence, Al13, whose EA was measured to be about 3.62 eV [Citation37], behaves as a halogen atom, and can be considered as a typical superhalogen. Moreover, the superatomic characteristics of other clusters, like Au20 [Citation60], ligated clusters [Citation54,Citation57,Citation61], Ti@Si16 [Citation62,Citation63] and so on, can also be attributed to the Jellium rule.
2.1.2 The Wade-Mingos rule
The Wade-Mingos rule is another effective strategy in designing superatoms, which is originally proposed by Wade and Mingos, and works well in the polyhedral borane clusters (BnHm) [Citation64–66]. In this rule, the valence electrons are separated into skeletal and external electrons, where the former form the cage bonding while the latter are responsible for covalent bonds with external ligands. Herein, 2n + 2, 2n + 4, and 2n + 6 electrons are needed to form closo, nido, and arachno boranes, in which n is the number of vertices in the borane polyhedron. B12H122- is a prime illustration of a closo-borane cluster, exhibiting a perfect icosahedral structure and ranking among the most stable dianions. Another example is the CB11H12 cluster. This cluster should mimic the chemistry of a halogen because it requires one more electron to satisfy the Wade-Mingos rule. Further theoretical study demonstrates its superhalogen characteristics because the EA of CB11H12 was calculated to be about 5.39 eV [Citation67]. Similar phenomena also exist in the MB12H12 (M = Li, Na, K, Rb, and Cs) clusters, which are all superhalogens [Citation67]. In addition, other clusters, such as Al4H6, Sn122-, and Pb122-, are also found to obey the Wade-Mingos rule [Citation68–70].
2.1.3 The octet shell closure rule
The octet rule is a simple electron-counting rule, which describes the chemistry of elements with a low atomic number (<20) [Citation71,Citation72]. Noble gas atoms exhibit chemical inertness as a result of their complete outer s2 and p6 electron shells. Conversely, halogen and alkali atoms are chemically reactive due to their deviation from the octet rule, with halogens having one electron less and alkalis having one electron more than required to satisfy this rule. The formula proposed by Gutsev and Boldyrev for designing superhalogens and superalkalis made use of such an octet electron-counting rule [Citation39,Citation40]. An example of the superhalogen is the LiF2 cluster with an EA value of 5.45 eV [Citation73], which is much larger than that of the F atom (3.40 eV). Multiple halogen atoms can also be attached to the metal atom to form superhalogens. As for AuF6, its EA is as high as 8.6 eV [Citation74]. In addition to metal clusters, the usage of the octet rule in constructing superhalogens can be extended to non-metallic clusters too. BH4 and CN are typical superhalogens with VDEs of 4.42 and 3.86 eV, respectively [Citation75,Citation76]. Similarly, a cluster, which has one extra electron than needed to meet the octet rule, should behave as an alkali atom. The Li3O cluster is just this case, and possesses an IP of 3.54 eV, which is lower than that of the Li atom (5.39 eV) [Citation77]. More intriguingly, a kind of cluster featuring higher EA values than the superhalogen can be formed by decorating a metal atom of valence k with (k +1) superhalogens, which is named as hyperhalogens [Citation78].
2.1.4 The 18-electron rule
The 18-electron rule is usually employed to design stable ‘magic’ superatoms involving the transition-metal (TM) atoms. Analogous to noble gas atoms, the 18-electron TM complexes are considered to achieve the electronic shell closure [Citation79]. Some examples are Cr(C6H6)2, Mo(CO)6, [Fe(CN)6]4- and so on. This rule also works in the charged clusters, like TaAu12¯ [Citation80]. This cluster is very stable, and its EA is 3.76 eV, which is an all metal superhalogen.
Note that, due to the word limitation, we have not included other rules, such as the aromatic rule, isovalent rule, and multiple electron-counting rule etc., in this concise review. However, despite their successful application in constructing superatoms over the past few decades, these conventional strategies have clear limitations, as discussed above. That is, these traditional methodologies focus on changing the compositions or the number of valence electrons of parent clusters, representing methods of altering the clusters’ intrinsic properties in the superatom design. Therefore, they are relatively complicated and inconvenient to manipulate, especially in experiments. Given this fact, it would be a significant advancement if one could conveniently regulate the electronic properties of clusters without changing their intrinsic properties. This has promoted researchers, including our own group, to examine the potential of various external fields in modulating the electronic properties for the purpose of designing novel superatoms, which may also boost the potential applications of superatoms.
2.2 EFRS in superatom design
2.2.1 The OEEF in the superhalogen design
Lately, OEEF has captured more spotlight as a potential candidate for future green and smart reagents in synthetic chemistry and chemical biology [Citation81]. Theoretically, the Shaik group has made great contributions in exhibiting the strong power of the OEEF in breaking chemical bonds, controlling reaction activity and so on [Citation82,Citation83]. Recent advanced STM technique employed by Aragonés et al. demonstrated the theoretically predicted catalytic capability of the OEEF in the Diels-Alder reaction, in which the OEEF strength generated by STM is relatively strong ranging from 0.3–1.0 V/Å3 [Citation84]. Moreover, OEEF was also introduced into the field of cluster science very recently to regulate the electronic and optical properties of metal clusters [Citation85,Citation86]. It was observed that the first hyperpolarizability of some cluster compounds, i.e., (NLi4)(BF4) and (BLi6)X (X = BeF3 and BF4), can be significantly increased by applying the OEEF with the intensity from 0 to 121 × 10−4 au [Citation85]. Additionally, Li et al. found that some all-metal electrides can act as infrared (IR) nonlinear optical (NLO) switches upon the introduction of the OEEF that can drive a long-range excess electron transfer, in which the switching mechanism is a distinct nonbonding evolution named electronic structure isomerization [Citation86].
The capability of the OEEF in regulating the electronic structures of metal clusters and designing superatoms was first attempted by our group theoretically [Citation87]. The naked Au19 and Au20 ()) clusters were taken as model systems, and density functional theory (DFT) was employed to examine the effect of OEEF on their electronic structures and properties. The EA values of these two model clusters were observed to continuously increase with the increment of OEEF ()). As for Au20, its EA value lifts to 3.75 eV at F+z = 0.018 au, enabling it to behave as a superhalogen (). Moreover, the average Au-Au distances () and the gaps between the highest occupied molecular orbital and the lowest unoccupied molecular orbital (HOMO-LUMO gap) in Au19 are almost invariant under different OEEF intensities, indicating that the geometrical and energetic stabilities can be well maintained during the application of OEEF. These findings demonstrate that the OEEF can indeed regulate the electronic properties of metal clusters while maintaining their stability, providing a new way to design novel superhalogens.
Figure 1. Geometrical and electronic properties of model Au clusters under OEEF [Citation87]. (a) Optimized geometry of Au19 without OEEF, (b) variation of different Au-Au distances (a, b, c, and d in Å) in Au19 under OEEF, and (c) and (d) theoretical EA values (in eV) of Au19 and Au20 as a function of different OEEF intensities (in au). Adapted with permission from ref 87. Copyright 2002 American Chemical Society.
![Figure 1. Geometrical and electronic properties of model Au clusters under OEEF [Citation87]. (a) Optimized geometry of Au19 without OEEF, (b) variation of different Au-Au distances (a, b, c, and d in Å) in Au19 under OEEF, and (c) and (d) theoretical EA values (in eV) of Au19 and Au20 as a function of different OEEF intensities (in au). Adapted with permission from ref 87. Copyright 2002 American Chemical Society.](/cms/asset/405e4105-f2db-4110-8382-4a557e133782/tapx_a_2244541_f0001_oc.jpg)
Apart from the regulation effect on the clusters’ electronic properties, the OEEF can also lead to the oriented charge transfer along the electric field direction (), which further influences the reaction activity. It was observed that the OEEF works like a hand pushing electrons from the bottom of Au19 to the top, which makes the top Au3 moiety experience the largest electron-withdrawing process (). Such an electron aggregation process on Au3 can further accelerate the CO oxidation reaction on it since it was found that the activation energy of the rate-determining step (Ea1, the OCOO moiety formation process) in the LH mechanism of the CO oxidation reaction significantly decreases following the monotonic enhancement of the OEEF intensities ()). The 0.36 eV activation barrier without the OEEF drops to 0.10 eV upon the introduction of a 0.008 au OEEF, making it possible to oxidize CO at a low temperature. Interestingly, there exists an excellent linear relationship between OEEF intensities and Ea (), implying that the applied OEEF can precisely alter the reaction barrier at will.
Figure 2. The effect of the OEEF on charge transfer and CO oxidation reaction [Citation87]. (a) NBO charge analysis for different regions under OEEF in Au19, the reaction pathway of CO oxidation in the LH mechanism on Au19 (b) without OEEF and (c) in different OEEF intensities, and (d) the reaction energy barriers under different OEEF intensities (in au). Adapted with permission from ref 87. Copyright 2002 American Chemical Society.
![Figure 2. The effect of the OEEF on charge transfer and CO oxidation reaction [Citation87]. (a) NBO charge analysis for different regions under OEEF in Au19, the reaction pathway of CO oxidation in the LH mechanism on Au19 (b) without OEEF and (c) in different OEEF intensities, and (d) the reaction energy barriers under different OEEF intensities (in au). Adapted with permission from ref 87. Copyright 2002 American Chemical Society.](/cms/asset/62543499-d01e-4414-bd9c-c5157fdefcd4/tapx_a_2244541_f0002_oc.jpg)
Similar situations also exist in the Ag19 cluster [Citation88]. That is, the non-superhalogen Ag19 cluster can behave as a superhalogen upon the application of the OEEF because the original 3.00 eV EA value of Ag19 in the absence of OEEF can go up to 3.88 eV at a 0.024 au F+z (). It is known that one extra electron occupies the LUMO of the neutral cluster to form the corresponding anion. Therefore, the LUMO level of the neutral cluster governs the capability of attracting electrons of the cluster, relating with the cluster’s EA value. Thus, based on the calculations about the one-electron energy levels of the neutral cluster under different OEEF intensities (), it is suggested that the enhancement of the EA values of Ag19 stems from the downward shift of the electronic spectrum caused by the introduction of the OEEF. Also, the calculated correlation between the EA values and the LUMO energy levels in Ag19 under different OEEF intensities, which has a perfect linear relationship (), provides further evidence about the nature of the observed increment of the EA values of Ag19 under OEEF. More importantly, for both the Au19 and Ag19 clusters, theoretical results show that a well-fitted polynomial formula could be obtained by fitting the calculated EA values with the OEEF intensities (), which means that one can obtain any desired EAs on the fitted curve by adjusting suitable OEEF intensities. This finding intriguingly exhibits the precise and continuous nature of the OEEF on the regulation effect of the electronic properties of metal clusters.
Figure 3. The effect of OEEF on the electronic properties of Ag19 [Citation88]. (a) Calculated EA values, (b) one-electron energy levels (in eV), and (c) correlation between the EA values and the LUMO energy levels of the neutral Ag19 cluster under different OEEF intensities (in au). Adapted with permission from ref 88. Copyright 1993 Elsevier.
![Figure 3. The effect of OEEF on the electronic properties of Ag19 [Citation88]. (a) Calculated EA values, (b) one-electron energy levels (in eV), and (c) correlation between the EA values and the LUMO energy levels of the neutral Ag19 cluster under different OEEF intensities (in au). Adapted with permission from ref 88. Copyright 1993 Elsevier.](/cms/asset/aad3bffb-0365-4362-b6cf-3c110348b6b8/tapx_a_2244541_f0003_oc.jpg)
Additionally, the regulation effect of the OEEF in the superhalogen construction also works in other cluster systems including the tungsten carbide cluster (W4C4) [Citation89], the zirconium oxide cluster (Zr3O3) [Citation90], the niobium nitride cluster (Nb2N2) [Citation38], and the manganese boride cluster (MnB6) [Citation91], which demonstrates the universality of this external field in the superatom design.
2.2.2 The ligand field in the superalkali and superhalogen design
The liquid-phase atomically precise cluster synthesis has experienced a rapid development during the past decade. Various metal clusters have been synthesized experimentally, including gold clusters, silver clusters, and other transition metal clusters etc [Citation22]. However, due to their high surface Gibbs free energy, naked metal clusters are always highly reactive, which are easy to agglomerate forming bigger nanoparticles. Thus, the ligation strategy is a universal approach in the chemical synthesis of metal clusters, which can stabilize the naked clusters. Except for the stabilization effect, various ligands are utilized to regulate the electronic properties of metal clusters recently, and even employed to construct novel superhalogens and superalkalis.
The electron-donating ligands PH3 and PEt3 were attached to an experimentally synthesized Ni9Te6 cluster, in which eight PH3 and PEt3 ligands connected to the Ni sites () [Citation92]. The bare Ni9Te6 cluster has a high AIP value of 5.87 eV. However, it was observed that the attachment of eight PH3 and PEt3 ligands significantly decrease its AIP to 4.64 and 3.36 eV, respectively, both featuring the superalkali characteristics. The electronic energy spectrum () reveals that, upon the ligation, the entire spectrum of Ni9Te6 goes to higher energies, where the electrons in the HOMOs of these ligated clusters are easier to be ionized. Moreover, in the ligation process, it was demonstrated that, in addition to the covalent bonding, the ligands can be thought of creating a crystal field due to the excess negative charge. Also, the shift of the HOMO and peaks () indicates that the presence of ligands induces an internal electrostatic coulomb potential well by charging the Ni9Te6 that lifts its quantum states. Apart from the regulation of the electronic properties, the ligation process can also affect the magnetic properties of the cluster ().
Figure 4. The (a) optimized structures of Ni9Te6 and Ni9Te6(PEt3)8, (b) calculated one-electron energy levels in the Ni9Te6, Ni9Te6(PH3)8, and Ni9Te6(PEt3)8 clusters, (c) PDOS of the 3d Ni and p Te states of the bare Ni9Te6 and Ni9Te6 in the presence of six fictitious point charges together with the PDOS between the 3d-Ni, p-Te, and p-P states for Ni9Te6(PH3)8, and (d) energy landscape for the magnetization direction as a function of ϕ and θ. Adapted with permission from ref 92. Copyright 2002 American Chemical Society.
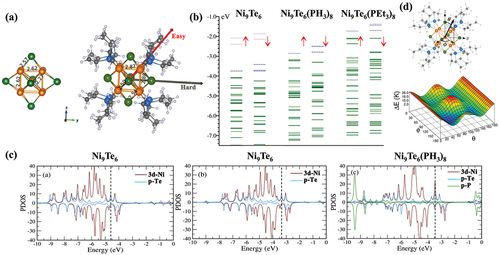
As mentioned above, modulation of the shell filling is a traditional strategy in designing superatoms. The species with one more or one less electron than the shell closure configuration usually behave as a superalkali or superhalogen. Interestingly, by employing the DFT calculations, Khanna et al. showed that the ionization energy of metal clusters featuring both filled and unfilled electronic shells can be significantly lowered by attaching suitable ligands [Citation93]. The N-ethyl-2-pyrrolidone (EP = C6H11NO) ligand was attached to several typical Al or doped Al clusters, which are 39 e (Al13 and BAl12), 40 e (Al13¯, CAl12, and SiAl12), and 41 e (PAl12) clusters (). It was observed that the ligation process can continuously decrease the adiabatic ionization energies (AIE) of these clusters with distinct valence electrons, leading to the formation of superalkalis (). More importantly, different from traditional methodologies, such a AIE reduction process does not alter the superatomic states of these clusters (). Additionally, the AIE lowering was attributed to the upward shift of the electronic spectrum of the ligand induced charge transfer complexes via crystal field like effect. Similar phenomena also exist in other ligated clusters, which demonstrate that such a ligand field is indeed a novel strategy in remarkably altering the electronic properties of metal clusters while maintaining their geometrical and electronic structures [Citation94].
Figure 5. Lowering the ionization energy of metallic clusters by organic ligands without changing shell filling [Citation93]. (a) The optimized geometries of Al130/-1 and MAl12 (M = B, C, Si, and P) clusters, (b) the adiabatic ionization energies of the bare Al13(EP)n and MAl12(EP)n (M = B, C, Si, and P; n = 0–3) clusters, and (c) molecular orbital iso-surfaces of CAl12(EP) cluster marked with the angular characters. Adapted with permission from ref 93. Copyright 2000 Nature Publishing Group.
![Figure 5. Lowering the ionization energy of metallic clusters by organic ligands without changing shell filling [Citation93]. (a) The optimized geometries of Al130/-1 and MAl12 (M = B, C, Si, and P) clusters, (b) the adiabatic ionization energies of the bare Al13(EP)n and MAl12(EP)n (M = B, C, Si, and P; n = 0–3) clusters, and (c) molecular orbital iso-surfaces of CAl12(EP) cluster marked with the angular characters. Adapted with permission from ref 93. Copyright 2000 Nature Publishing Group.](/cms/asset/f7935884-97b0-458c-913c-38000981043f/tapx_a_2244541_f0005_oc.jpg)
Different from the OEEF that only works well in constructing superhalogens, it has been demonstrated that the ligand field can regulate the electronic properties of metal clusters in producing both the superalkalis and superhalogens. In one of our recent reports [Citation95], ligands with different electron characteristics, i.e. the electron-donating PH3 and electron-withdrawing CO ligands, were connected to the cubic W4C4 cluster to form the W4C4Ln (L = CO, PH3; n = 1–6) complexes (). The AEAs of the clusters increase monotonically with the continuous addition of the CO ligand, while the PH3 ligation process reduces the AIP of W4C4 (). And obviously, upon the ligation of six CO ligands, W4C4(CO)6 turns to behave as a superhalogen since it has an AEA value of 3.23 eV (), which is higher than that of the I atom (3.06 eV). The superatomic properties of these ligated clusters were further verified by exploring the behavior of the salt generated via the superatoms. In this salt, a significant charge transfer (−0.95 |e|) takes place between W4C4(CO)6 and the Na atom, resulting in an obvious ionic bond pattern (). These findings clearly demonstrate that appropriate ligands can manipulate the electronic properties of clusters for the construction of the superhalogen and superalkali species. Meanwhile, the potential of assembling the cubic W4C4 cluster into a cluster solid nanomaterial was also examined (), which exhibits a good stability and the metallic property [Citation95].
Figure 6. The ligand field strategy in the superatom design [Citation95]. The (a) optimized ground states of the neutral W4C4(CO)n and W4C4(PH3)n (n = 1–6) clusters, (b) theoretical AEAs and AIPs of the ligated W4C4Ln (L = CO, PH3; n = 1–6) clusters, (c) optimized ground-state structures of NaW4C4(CO)6, NaW4C4 and the cut-plane LOL of the NaW4C4(CO)6 cluster, and (d) the model of the W4C4 cluster solid. Adapted with permission from ref 95. Copyright 2001 the Royal Society of Chemistry.
![Figure 6. The ligand field strategy in the superatom design [Citation95]. The (a) optimized ground states of the neutral W4C4(CO)n and W4C4(PH3)n (n = 1–6) clusters, (b) theoretical AEAs and AIPs of the ligated W4C4Ln (L = CO, PH3; n = 1–6) clusters, (c) optimized ground-state structures of NaW4C4(CO)6, NaW4C4 and the cut-plane LOL of the NaW4C4(CO)6 cluster, and (d) the model of the W4C4 cluster solid. Adapted with permission from ref 95. Copyright 2001 the Royal Society of Chemistry.](/cms/asset/16e70ce3-aaba-4076-b3d0-31be2214dab2/tapx_a_2244541_f0006_oc.jpg)
In addition to the theoretical design, this kind of ligated cluster can also be produced in experiments [Citation96,Citation97]. By using Co6S8(PEt3)6 as a parent compound, Liu et al. synthesized the CO substituted Co6S8(PEt3)6-x(CO)x clusters, which were then brought into the gas phase to form the corresponding anions by employing an infrared desorption/photoelectron-emission/supersonic helium expansion source [Citation96]. Mass spectrometry () confirms the successful production of these clusters with x up to 3, which are Co6S8(PEt3)5(CO)¯, Co6S8(PEt3)4(CO)2¯, and Co6S8(PEt3)3(CO)3¯, and corresponding photoelectron spectra of these ligated clusters were also obtained (). According to the experimental spectra and optimized ligated clusters (), it was observed that the clusters’ EA and VDE increase with the number of the CO ligands (), which demonstrates the tunability of the electronic spectra of these ligated clusters.
Figure 7. Turning the electronic properties via ligand substitution [Citation96]. The (a) structure of Co6S8(PEt3)6-x and mass spectrum of Co6S8(PEt3)6-x(CO)x¯ produced utilizing IR/PE anion source, (b) negative ion photoelectron spectra of Co6S8(PEt3)6-x(CO)x¯ (x = 0–3), (c) theoretical lowest-energy structures of the Co6S8(PEt3)6-x(CO)x¯ (x = 0–3) clusters, and (d) incremental differences in the experimental AEA values, the theoretical AEA values, and the LUMO values of Co6S8(PEt3)6-x(CO)x, each with respect to Co6S8(PEt3)6. Adapted with permission from ref 96. Copyright 2001 the Royal Society of Chemistry.
![Figure 7. Turning the electronic properties via ligand substitution [Citation96]. The (a) structure of Co6S8(PEt3)6-x and mass spectrum of Co6S8(PEt3)6-x(CO)x¯ produced utilizing IR/PE anion source, (b) negative ion photoelectron spectra of Co6S8(PEt3)6-x(CO)x¯ (x = 0–3), (c) theoretical lowest-energy structures of the Co6S8(PEt3)6-x(CO)x¯ (x = 0–3) clusters, and (d) incremental differences in the experimental AEA values, the theoretical AEA values, and the LUMO values of Co6S8(PEt3)6-x(CO)x, each with respect to Co6S8(PEt3)6. Adapted with permission from ref 96. Copyright 2001 the Royal Society of Chemistry.](/cms/asset/de1ff414-b2fe-4c2d-8d9a-7c2c39e58cd5/tapx_a_2244541_f0007_oc.jpg)
Other examples of the ligand field induced superatom formation have also been discovered, demonstrating the feasibility and universality of the ligand field in designing novel superatoms [Citation38,Citation98–102].
2.2.3 The solvent field in the superhalogen construction
Apart from the aforementioned OEEF and ligand field, another external field that has recently garnered our interest is the solvent field [Citation103]. Typical pure and doped gold cluster anions (Aun¯ (n = 2, 4, and 8) and MAu8¯ (M = Ge, Sn)) featuring various geometries, including the linear, planar, and 3D structures, were taken as model systems (). Compared with the gas phase, it was observed that polar solvents can obviously increase the VDEs of these model clusters giving rise to the superhalogen anions, and the VDE values increase following the enhancement of the static dielectric constants of the solvents (). The one-electron energy level calculations also demonstrate that the downward shift of the electronic spectrum accounts for the changes from non-superhalogen to superhaogen in different solvents (). These findings provide solid evidence that the solvent field can be considered as an effective external field in the superhalogen modulation, in which it can significantly increase the ability of the electron-withdrawing of gold cluster anions while maintaining their geometrical and electronic structures ().
Figure 8. Geometrical and electronic structures of anions under solvent [Citation103]. The (a) theoretical lowest-energy geometries of the Aun¯ (n = 2, 4, and 8) and MAu8¯ (M = Ge, Sn) clusters, (b) calculated VDE values and (c) one-electron energy levels of the Au4¯ cluster as well as (d) the average Au-Au bond lengths (in Å) of Aun¯ (n = 2, 4, and 8) and MAu8¯ (M = Ge, Sn) in the gas phase and different solvents. Adapted with permission from ref 103. Copyright 1993 Elsevier.
![Figure 8. Geometrical and electronic structures of anions under solvent [Citation103]. The (a) theoretical lowest-energy geometries of the Aun¯ (n = 2, 4, and 8) and MAu8¯ (M = Ge, Sn) clusters, (b) calculated VDE values and (c) one-electron energy levels of the Au4¯ cluster as well as (d) the average Au-Au bond lengths (in Å) of Aun¯ (n = 2, 4, and 8) and MAu8¯ (M = Ge, Sn) in the gas phase and different solvents. Adapted with permission from ref 103. Copyright 1993 Elsevier.](/cms/asset/b846b7c1-8acc-41dc-8d0d-d6fb026781af/tapx_a_2244541_f0008_oc.jpg)
In addition to model systems, a stable and experimentally identified gold nanocluster was also adopted to explore the regulation effect of the solvent. It was found that the cluster’s VDE increases from the original 2.36 eV to 3.22 eV by simply introducing the H2O solvent, reaching the superhalogen zone (). This shows that the solvent field is effective in the superhalogen formation for both the simplified model naked clusters and practical ligand-protected nanoclusters. Moreover, by utilizing the DFT calculations with the explicit solvation model and the QM/MM simulations, it has been demonstrated that the solvent field can indeed enhance the electron-binding ability of the nanocluster anion rather than the ability of the solvent, H2O here, itself ().
Figure 9. The (a) optimized structure of the Au18(SCH3)14¯ cluster in water and VDE values together with HOMO levels of Au18(SCH3)14¯ in the gas phase and water, (b) optimized geometries of Au18_nc@82H2O¯ and Au18_nc@43H2O@500H2O¯ used in the explicit solvent model and QM/MM MD simulation, and (c) schematic figure of the solvent field regulated superhalogen [Citation103]. Adapted with permission from ref 103. Copyright 1993 Elsevier.
![Figure 9. The (a) optimized structure of the Au18(SCH3)14¯ cluster in water and VDE values together with HOMO levels of Au18(SCH3)14¯ in the gas phase and water, (b) optimized geometries of Au18_nc@82H2O¯ and Au18_nc@43H2O@500H2O¯ used in the explicit solvent model and QM/MM MD simulation, and (c) schematic figure of the solvent field regulated superhalogen [Citation103]. Adapted with permission from ref 103. Copyright 1993 Elsevier.](/cms/asset/eff68ee1-a1c2-436e-8c6e-273a641b4c29/tapx_a_2244541_f0009_oc.jpg)
2.2.4 Dual external field (DEF) strategy in superatom design
Considering the fact that different external fields, e.g., the OEEF, the ligand field, and the solvent field, have the capability in regulating the electronic properties of metal clusters in constructing novel superatoms, it spurs our further interests in examining the modulation effect of the combination of multiple external fields. To this end, a dual external field (DEF) strategy was proposed by our group to combine the ligand field and OEEF to design hyperhalogen () [Citation104]. The design idea is to attach suitable ligands to metal clusters to obtain the superhalogen first, followed by the introduction of the OEEF to realize the formation of the hyperhalogen (). Different numbers of electron-withdrawing BO2 ligands were linked to the model Au8 cluster to form the ligated clusters (). Au8(BO2)2 was estimated to have the highest EA value (3.60 eV), which exhibits the superhalogen characteristic (). Strikingly, the EA value of the Au8(BO2)2 cluster dramatically soars to 6.73 eV upon the application of a 0.018 au OEEF, a second external field, giving rise to the hyperhalogen (). Therefore, it is obvious that the proposed DEF strategy, i.e., the ligation and the OEEF synergistic strategy, indeed can efficiently manipulate the electronic properties of clusters resulting in the formation of hyperhalogen, which represents a novel methodology in the hyperhalogen design. Furthermore, to further testify the applicability and universality of the proposed DEF strategy in more practical systems, an experimentally synthesized Ag17 nanocluster was adopted (), and it is apparent that the proposed DEF strategy also works in more practical systems. Thus, the proposed DEF strategy provides a potential methodology for constructing hyperhalogens in the chemical synthesis of atomically precise clusters. That is, applying suitable ligands to first synthesize stable ligand-protected clusters followed by imposing the OEEF via the mechanically controllable break junction (MCBJ) technique.
Figure 10. Dual external field-engineered hyperhalogen [Citation104]. The (a) schematic description of the concept of the proposed DEF regulation strategy for constructing the hyperhalogen, (b) optimized ground states of the neutral and anionic Au8(BO2)n (n = 1−4) clusters, (c) calculated EA values of the Au8(BO2)n (n = 1−4) clusters, (d) EA values of the Au8(BO2)2 and Au8 clusters under different OEEF intensities, and (e) geometry of the neutral Ag17_nc as well as the EA values of Ag17_nc under different OEEF intensities. Adapted with permission from ref 104. Copyright 2002 American Chemical Society.
![Figure 10. Dual external field-engineered hyperhalogen [Citation104]. The (a) schematic description of the concept of the proposed DEF regulation strategy for constructing the hyperhalogen, (b) optimized ground states of the neutral and anionic Au8(BO2)n (n = 1−4) clusters, (c) calculated EA values of the Au8(BO2)n (n = 1−4) clusters, (d) EA values of the Au8(BO2)2 and Au8 clusters under different OEEF intensities, and (e) geometry of the neutral Ag17_nc as well as the EA values of Ag17_nc under different OEEF intensities. Adapted with permission from ref 104. Copyright 2002 American Chemical Society.](/cms/asset/e03f8ec4-ba92-4490-988d-921db668c3ce/tapx_a_2244541_f0010_oc.jpg)
3. Conclusions and outlooks
In this concise review, we first present the basic concept of the superatoms, and discuss traditional electron-counting rules (ECR), including the Jellium shell closure rule, the Wade-Mingos rule, the octet shell closure rule, and the 18-electron rule, for the superatom design. The focus of the present review is the recently advanced external-field regulated strategies (EFRS) in the theoretical design of superatoms. Combining the research work conducted by our group and other peers, various external-field methodologies, including the OEEF, the ligand field, and the solvent field, are described and discussed. Admittedly, due to the limitations of space, we do not provide an exhaustive review of all outstanding work related to cluster science and superatoms.
As discussed above, conventional ECRs need to change the clusters’ intrinsic properties, e.g., the compositions and the number of valence electrons of parent clusters, which is relatively complicated and inconvenient to regulate in both the gas-phase and liquid-phase experiments. In comparison to existing ECRs, the EFRS offers distinct advantages as a novel and noninvasive methodology. It not only enables the regulation of electronic properties in metal clusters, forming superatoms, but also ensures the preservation of their geometrical and energetic stability. From the point of view of the atomically precise cluster synthesis experiments, ligands, which can stabilize the naked cluster core, and solvents are indispensable and conveniently available. Thus, the discovery of the regulation effect of the ligand field and solvent field in dramatically altering the electronic properties of clusters without destroying their geometrical and energetic stability is of great significance. However, a drawback of these two external field strategies is the challenge of precisely adjusting the electronic properties of clusters. Because the number of the ligands that we can attach to the naked cluster cores is integral and different polar solvents have fixed static dielectric constants, the electronic properties, e.g., the EA and IP values, that these external fields can modulate are discontinuous. For instance, the theoretical EA values of Co6Te8(PEt3)n (n = 1–6) are six fixed values because one can just attach integral ligands to the naked core in the cluster synthesis. Such a drawback could be overcome by the OEEF methodology, which can realize the precise and continuous regulation of the electronic properties of clusters because the OEEF intensity is continuously adjustable. The capability of the precise and continuous regulation of the OEEF represents a significant advantage over conventional ECR strategies. However, unlike the ligand field and solvent field that are easy to achieve in the liquid-phase synthesis, the manipulation of the OEEF on precisely controlling the conformation of clusters in the liquid-phase experiments is still a challenge. From this point of view, in experiments, one needs to fix the cluster materials between gold STM tips or on the substrates, and the directions of the OEEF could be adjusted based on the actual alignment of the clusters. Recently advanced scanning tunneling microscope break junction (STM-BJ) and mechanically controllable break junction (MCBJ) methods may help in this case [Citation84,Citation105,Citation106].
The EFRS opens a new avenue in the superatom design without disturbing the clusters’ intrinsic properties. However, as a new concept, further development is required to establish its theoretical framework. Some fundamental research topics, including understanding the inherent nature of the regulation effect of EFRS, exploring other valid external fields, and effectively coupling multiple external fields, are deserved to be comprehensively examined in the future.
Funding
This work is supported by the National Natural Science Foundation of China (NSFC) (Nos. 92161101, 21603119), the Taishan Scholars Project of Shandong Province (ts201712011), the Innovation Project of Jinan Science and Technology Bureau (2021GXRC032), the Instrument Improvement Funds of Shandong University Public Technology Platform (No. ts20220201), the Key Research & Developmental Program of Shandong Province (2021CXGC011202), the Natural Science Foundation of Shandong Province (ZR2020ZD35), the Young Scholars Program of Shandong University (YSPSDU, 2018WLJH48), the Qilu Youth Scholar Funding of Shandong University. The HPC Cloud Platform of Shandong University is also acknowledged for the support of theoretical calculations in some research references in this review.
Disclosure statement
No potential conflict of interest was reported by the author(s).
Additional information
Funding
References
- Claridge SA, Castleman AW Jr., Khanna SN, et al. Cluster-assembled materials. ACS Nano. 2009;3:244–255. doi: 10.1021/nn800820e.
- Guo BC, Kerns KP, Castleman AW Jr. Ti8C12+-Metallo-Carbohedrenes: A new class of molecular clusters? Science. 1992;255:1411–1413. doi: 10.1126/science.255.5050.1411.
- AW C Jr., Bowen KH. Clusters: Structure, energetics, and dynamics of intermediate states of matter. J Phys Chem. 1996;100:12911–12944. doi: 10.1021/jp961030k.
- Bergeron DE, Roach PJ, Castleman AW Jr., et al. Al cluster superatoms as halogens in polyhalides and as alkaline earths in iodide salts. Science. 2005;307:231–235. doi: 10.1126/science.1105820.
- Jena P, Castleman AW Jr. Clusters: A bridge across the disciplines of physics and chemistry. Proc Natl Acad Sci U S A. 2006;103:10560–10569. doi: 10.1073/pnas.0601782103.
- Roach PJ, Woodward WH, Castleman AW Jr., et al. Complementary active sites cause size-selective reactivity of aluminum cluster anions with water. Science. 2009;323:492–495. doi: 10.1126/science.1165884.
- Luo Z, AW C Jr. Special and general superatoms. Acc Chem Res. 2014;47:2931–2940. doi: 10.1021/ar5001583.
- Luo Z, AW C Jr., Khanna SN. Reactivity of metal clusters. Chem Rev. 2016;116(23):14456–14492. doi: 10.1021/acs.chemrev.6b00230.
- Cheng SB, Berkdemir C, Castleman AW Jr. Observation of d–p hybridized aromaticity in lanthanum-doped boron clusters. Phys Chem Chem Phys. 2014;16:533–539. doi: 10.1039/C3CP53245C.
- Cheng SB, Berkdemir C, Melko JJ, et al. S-P coupling induced unusual open-shell metal clusters. J Am Chem Soc. 2014;136:4821–4824. doi: 10.1021/ja412637j.
- Cheng SB, Berkdemir C, Castleman AW Jr. Mimicking the magnetic properties of rare earth elements using superatoms. Proc Natl Acad Sci U S A. 2015;112:4941–4945. doi: 10.1073/pnas.1504714112.
- Cheng SB, Castleman AW Jr. Direct experimental observation of weakly-bound character of the attached electron in europium anion. Sci Rep. 2015;5:12414. doi: 10.1038/srep12414.
- Cheng SB, Harmon CL, Yang H, et al. Electronic structure of the diatomic VO anion: A combined photoelectron-imaging spectroscopic and theoretical investigation. Phy Rev A. 2016;94:062506. doi: 10.1103/PhysRevA.94.062506.
- Lu S, Hu K, Zuo Z, et al. Beam generation and structural optimization of size-selected Au923 clusters. Nanoscale Adv. 2020;2:2720–2725. doi: 10.1039/D0NA00304B.
- Zhang K, Wang C, Zhang M, et al. A Gd@C82 single-molecule electret. Nat Nanotech. 2020;15:1019–1024. doi: 10.1038/s41565-020-00778-z.
- Wei X, Kang X, Zuo Z, et al. Hierarchical structural complexity in atomically precise nanocluster frameworks. Nat Sci Rev. 2021;8:nwaa077. doi: 10.1093/nsr/nwaa077.
- Hu KJ, Yan W, Zhang M, et al. Electrical devices designed based on inorganic clusters. Nanotechnology. 2022;33:502001. doi: 10.1088/1361-6528/ac8f4e.
- Sun Q, Wang Q, Jena P, et al. Clustering of Ti on a C60 surface and its effect on hydrogen storage. J Am Chem Soc. 2005;127:14582–14583. doi: 10.1021/ja0550125.
- Sun Q, Jena P, Wang Q, et al. First-principles study of hydrogen storage on Li12C60. J Am Chem Soc. 2006;128:9741–9745. doi: 10.1021/ja058330c.
- Jena P. Beyond the periodic table of elements: The role of superatoms. J Phys Chem Lett. 2013;4:1432–1442. doi: 10.1021/jz400156t.
- Zhang S, Zhou J, Wang Q, et al. Penta-graphene: A new carbon allotrope. Proc Natl Acad Sci U S A. 2015;112:2372–2377. doi: 10.1073/pnas.1416591112.
- Jena P, Sun Q. Super atomic clusters: Design rules and potential for building blocks of materials. Chem Rev. 2018;118:5755–5870. doi: 10.1021/acs.chemrev.7b00524.
- Yin B, Du Q, Geng L, et al. Anionic copper clusters reacting with NO: An open-shell superatom Cu18¯. J Phys Chem Lett. 2020;11:5807–5814. doi: 10.1021/acs.jpclett.0c01643.
- Yin B, Du Q, Geng L, et al. Superatomic signature and reactivity of silver clusters with oxygen: double magic Ag17– with geometric and electronic shell closure. CCS Chem. 2021;3:219–229. doi: 10.31635/ccschem.020.202000719.
- Du Q, Yin B, Zhou S, et al. The reactivity of O2 with copper cluster anions Cu− (n = 7−20): leveling effect of spin accommodation. Chin Chem Lett. 2022;33:995–1000. doi: 10.1016/j.cclet.2021.08.127.
- Yu X, Su Y, Xu WW, et al. Efficient photoexcited charge separation at the interface of a novel 0D/2D heterojunction: A time-dependent ultrafast dynamic study. J Phys Chem Lett. 2021;12:2312–2319. doi: 10.1021/acs.jpclett.1c00023.
- Yu X, Sun Y, Xu WW, et al. Tuning photoelectron dynamic behavior of thiolate-protected MAu24 nanoclusters via heteroatom substitution. Nanoscale Horiz. 2022;7:1192–1200. doi: 10.1039/D2NH00281G.
- Sun Y, Yu X, Liu P, et al. Isomerism effects in relaxation dynamics of Au24(SR)16 thiolate-protected gold nanoclusters. Nanotechnology. 2023;34:105701. doi: 10.1088/1361-6528/aca80d.
- Li XN, Jiang LX, Wang LN, et al. An eight-atom iridium-aluminum oxide cluster IrAlO6+ catalytically oxidizes six CO molecules. J Phys Chem Lett. 2019;10:7850–7855. doi: 10.1021/acs.jpclett.9b03056.
- Chen JJ, Li XN, Liu QY, et al. Water gas shift reaction catalyzed by rhodium-manganese oxide cluster anions. J Phys Chem Lett. 2021;12:8513–8520. doi: 10.1021/acs.jpclett.1c02267.
- Chen LS, Liu YZ, Li XN, et al. An IrVO4+ cluster catalytically oxidizes four CO molecules: Importance of Ir-V multiple bonding. J Phys Chem Lett. 2021;12:6519–6525. doi: 10.1021/acs.jpclett.1c01584.
- Liu Z, Wu X, Zhu Y, et al. Superatomic Rydberg state excitation. J Phys Chem Lett. 2021;12:11766–11771. doi: 10.1021/acs.jpclett.1c03520.
- Huang W, Zhang Q, Wang R, et al. Super-excimer: Anomalous bonding in a metastable excited-state dimer of superatomic dimers. J Phys Chem Lett. 2022;13:8455–8461. doi: 10.1021/acs.jpclett.2c02271.
- Li J, Wang R, Huang W, et al. Smallest endohedral metallofullerenes [Mg@C20]n (n = 4, 2, 0, −2, and −4): endo-ionic interaction in superatoms. J Phys Chem Lett. 2023;14:2862–2868. doi: 10.1021/acs.jpclett.3c00445.
- Khanna SN, Jena P. Assembling crystals from clusters. Phys Rev Lett. 1992;69:1664–1667. doi: 10.1103/PhysRevLett.69.1664.
- Bergeron DE, Castleman AW Jr., Morisato T, et al. Formation of Al13I−: evidence for the superhalogen character of Al13. Science. 2004;304:84–87. doi: 10.1126/science.1093902.
- Li X, Wu HB, Wang XB, et al. s-p hybridization and electron shell structures in aluminum clusters: A photoelectron spectroscopy study. Phys Rev Lett. 1998;81:1909–1912. doi: 10.1103/PhysRevLett.81.1909.
- Li J, Zhao Y, Bu YF, et al. On the theoretical construction of Nb2N2-based superatoms by external field strategies. Chem Phys Lett. 2020;754:137709. doi: 10.1016/j.cplett.2020.137709.
- Gutsev GL, Boldyrev AI. DVM-Xα calculations on the electronic structure of “superalkali” cations. Chem Phys Lett. 1982;92:262–266. doi: 10.1016/0009-2614(82)80272-8.
- Gutsev GL, Boldyrev AI. DVM-Xα calculations on the ionization potentials of MXk+1−complex anions and the electron affinities of MXk+1“superhalogens”. Chem Phys. 1981;56:277–283. doi: 10.1016/0301-0104(81)80150-4.
- Castleman AW Jr., Khanna SN, Clusters. Superatoms, and building blocks of new materials. J Phys Chem C. 2009;113:2664–2675. doi: 10.1021/jp806850h.
- Zhu MZ, Aikens CM, Hendrich MP, et al. Reversible switching of magnetism in thiolate-protected Au25 superatoms. J Am Chem Soc. 2009;131:2490–2492. doi: 10.1021/ja809157f.
- Reber AC, Khanna SN, AW C Jr. Superatom compounds, clusters, and assemblies: Ultra alkali motifs and architectures. J Am Chem Soc. 2007;129:10189–10194. doi: 10.1021/ja071647n.
- Chen S, Du W, Qin C, et al. Assembly of the thiolated Au1Ag22(S-Adm)123+ superatom complex into a framework material through direct linkage by SbF6¯ anions. Angew Chem Int Ed. 2020;59:7542–7547. doi: 10.1002/anie.202000073.
- Cheng L, Yuan Y, Zhang X, et al. Superatom networks in thiolate-protected gold nanoparticles. Angew Chem Int Ed. 2013;52:9035–9039. doi: 10.1002/anie.201302926.
- Dougherty DB, Feng M, Petek H, et al. Band formation in a molecular quantum well via 2D superatom orbital interactions. Phys Rev Lett. 2012;109:266802. doi: 10.1103/PhysRevLett.109.266802.
- Li Y, Wu D, Li ZR. Compounds of superatom clusters: Preferred structures and significant nonlinear optical properties of the BLi6-X (X = F, LiF2, BeF3, BF4) motifs. Inorg Chem. 2008;47:9773–9778. doi: 10.1021/ic800184z.
- Luo Z, Reber AC, Jia M, et al. What determines if a ligand activates or passivates a superatom cluster? Chem Sci. 2016;7:3067–3074. doi: 10.1039/C5SC04293C.
- Pei Y, Lin S, Su J, et al. Structure prediction of Au44(SR)28: a chiral superatom cluster. J Am Chem Soc. 2013;135:19060–19063. doi: 10.1021/ja409788k.
- Peppernick SJ, Gunaratne KDD, Castleman AW Jr. Superatom spectroscopy and the electronic state correlation between elements and isoelectronic molecular counterparts. Proc Natl Acad Sci U S A. 2010;107:975–980. doi: 10.1073/pnas.0911240107.
- Reimers JR, Wang Y, Cankurtaran BO, et al. Chemical analysis of the superatom model for sulfur-stabilized gold nanoparticles. J Am Chem Soc. 2010;132:8378–8384. doi: 10.1021/ja101083v.
- Stoll T, Sgro E, Jarrett JW, et al. Superatom state-resolved dynamics of the Au25(SC8H9)18¯ cluster from two-dimensional electronic spectroscopy. J Am Chem Soc. 2016;138:1788–1791. doi: 10.1021/jacs.5b12621.
- Takano S, Hirai H, Muramatsu S, et al. Hydride-doped gold superatom (Au9H)2+: Synthesis, structure, and transformation. J Am Chem Soc. 2018;140:8380–8383. doi: 10.1021/jacs.8b03880.
- Tofanelli MA, Ackerson CJ. Superatom electron configuration predicts thermal stability of Au25(SR)18 nanoclusters. J Am Chem Soc. 2012;134:16937–16940. doi: 10.1021/ja3072644.
- Wang Y, Su H, Xu C, et al. An intermetallic Au24Ag20 superatom nanocluster stabilized by labile ligands. J Am Chem Soc. 2015;137:4324–4327. doi: 10.1021/jacs.5b01232.
- Weber TM, Hoening M, Niederpruem T, et al. Mesoscopic Rydberg-blockaded ensembles in the superatom regime and beyond. Nat Phys. 2015;11:157–161. doi: 10.1038/nphys3214.
- Walter M, Akola J, Lopez-Acevedo O. A unified view of ligand-protected gold clusters as superatom complexes. Proc Natl Acad Sci U S A. 2008;105:9157–9162. doi: 10.1073/pnas.0801001105.
- Chou MY, Cleland A, Cohen ML. Total energies, abundances, and electronic shell structure of lithium, sodium, and potassium clusters. Solid State Commun. 1984;52:645–648. doi: 10.1016/0038-1098(84)90725-7.
- Leuchtner RE, Harms AC, Castleman AW Jr. Thermal metal cluster anion reactions: Behavior of aluminum clusters with oxygen. J Chem Phys. 1989;91:2753–2754. doi: 10.1063/1.456988.
- Li J, Li X, Zhai HJ, et al. Au20: a tetrahedral cluster. Science. 2003;299:864–867. doi: 10.1126/science.1079879.
- Clayborne PA, Lopez-Acevedo O, Whetten RL, et al. The Al50Cp*12 Cluster: A 138-electron closed shell (L = 6) superatom. Eur J Inorg Chem. 2011;17:2649–2652. doi: 10.1002/ejic.201100374.
- Kumar V, Kawazoe Y. Metal-encapsulated fullerenelike and cubic caged clusters of silicon. Phys Rev Lett. 2001;87:045503. doi: 10.1103/PhysRevLett.87.045503.
- Kumar V, Kawazoe Y. Metal-encapsulated caged clusters of germanium with large gaps and different growth behavior than silicon. Phys Rev Lett. 2002;88:235504. doi: 10.1103/PhysRevLett.88.235504.
- Wade K. The structural significance of the number of skeletal bonding electron-pairs in carboranes, the higher boranes and borane anions, and various transition-metal carbonyl cluster compounds. J Chem Soc D. 1971;:792–793. doi: 10.1039/c29710000792.
- Mingos DMP. A general theory for cluster and ring compounds of the main group and transition elements. Nat Phys Sci. 1972;236:99–102. doi: 10.1038/physci236099a0.
- Mingos DMP. Polyhedral skeletal electron pair approach. Acc Chem Res. 1984;17:311–319. doi: 10.1021/ar00105a003.
- Pathak B, Samanta D, Ahuja R, et al. Borane derivatives: A new class of super- and hyperhalogens. Chemphyschem. 2011;12:2423–2428. doi: 10.1002/cphc.201100320.
- Li X, Grubisic A, Stokes ST, et al. Unexpected stability of Al4H6: a borane analog? Science. 2007;315:356–358. doi: 10.1126/science.1133767.
- Cui LF, Huang X, Wang LM, et al. Sn122-: Stannaspherene. J Am Chem Soc. 2006;128:8390–8391. doi: 10.1021/ja062052f.
- Cui LF, Huang X, Wang LM, etal. Pb122-: Plumbaspherene. J Phys Chem A. 2006;110:10169–10172. doi: 10.1021/jp063617x.
- Lewis GN. The atom and the molecule. J Am Chem Soc. 1916;38:762–785. doi: 10.1021/ja02261a002.
- Langmuir I. The arrangement of electrons in atoms and molecules. J Am Chem Soc. 1919;41:868–934. doi: 10.1021/ja02227a002.
- Gutsev GL, Bartlett RJ, Boldyrev AI, et al. Adiabatic electron affinities of small superhalogens: LiF2, LiCl2, NaF2, and NaCl2. J Chem Phys. 1997;107:3867–3875. doi: 10.1063/1.474764.
- Koirala P, Willis M, Kiran B, et al. Superhalogen properties of fluorinated coinage metal clusters. J Phys Chem C. 2010;114:16018–16024. doi: 10.1021/jp101807s.
- Paduani C, Wu MM, Willis M, et al. Theoretical study of the stability and electronic structure of Al(BH4)n=1→4 and Al(BF4) n=1→4 and their hyperhalogen behavior. J Phys Chem A. 2011;115:10237–10243. doi: 10.1021/jp206330d.
- Bradforth SE, Kim EH, Arnold DW, et al. Photoelectron spectroscopy of CN−, NCO−, and NCS−. J Chem Phys. 1993;98:800–810. doi: 10.1063/1.464244.
- Lievens P, Thoen P, Bouckaert S, et al. Ionization potentials of LinO (2⩽n⩽70) clusters: Experiment and theory. J Chem Phys. 1999;110:10316–10329. doi: 10.1063/1.478965.
- Willis M, Gotz M, Kandalam AK, et al. Hyperhalogens: Discovery of a new class of highly electronegative species. Angew Chem Int Ed. 2010;49:8966–8970. doi: 10.1002/anie.201002212.
- Langmuir I. Types of valence. Science. 1921;54:59–67. doi: 10.1126/science.54.1386.59.
- Pyykko P, Runeberg N. Icosahedral WAu12: a predicted closed-shell species, stabilized by aurophilic attraction and relativity and in accord with the 18-electron rule. Angew Chem Int Ed. 2002;41:2174–2176. doi: 10.1002/1521-3773(20020617)41:12<2174:AID-ANIE2174>3.0.CO;2-8.
- Stuyver T, Danovich D, Joy J, et al. External electric field effects on chemical structure and reactivity. WIREs Comput Mol Sci. 2020;10:e1438. doi: 10.1002/wcms.1438.
- Shaik S, Ramanan R, Danovich D, et al. Structure and reactivity/selectivity control by oriented-external electric fields. Chem Soc Rev. 2018;47:5125–5145. doi: 10.1039/C8CS00354H.
- Shaik S, Mandal D, Ramanan R. Oriented electric fields as future smart reagents in chemistry. Nat Chem. 2016;8:1091–1098. doi: 10.1038/nchem.2651.
- Aragonès AC, Haworth NL, Darwish N, et al. Electrostatic catalysis of a Diels–Alder reaction. Nature. 2016;531:88–91. doi: 10.1038/nature16989.
- Sun WM, Li CY, Kang J, et al. Superatom compounds under oriented external electric fields: Simultaneously enhanced bond energies and nonlinear optical responses. J Phys Chem C. 2018;122:7867–7876. doi: 10.1021/acs.jpcc.8b01896.
- He HM, Li Y, Yang H, et al. Efficient external electric field manipulated nonlinear optical switches of all-metal electride molecules with infrared transparency: Nonbonding electron transfer forms an excess electron lone pair. J Phys Chem C. 2017;121:958–968. doi: 10.1021/acs.jpcc.6b11919.
- Zhao Y, Wang J, Huang HC, et al. Tuning the electronic properties and performance of low-temperature CO oxidation of the gold cluster by oriented external electronic field. J Phys Chem Lett. 2020;11:1093–1099. doi: 10.1021/acs.jpclett.9b03794.
- Zhao Y, Chen J, Yang H, et al. A density functional theory calculation on the geometrical structures and electronic properties of Ag19 under the oriented external electric field. Chem Phys Lett. 2020;754:137703. doi: 10.1016/j.cplett.2020.137703.
- Duan YJ, Zhao Y, Cheng SB, et al. On the precise and continuous regulation of the superatomic and spectroscopic behaviors of the quasi-cubic W4C4 cluster by the oriented external electric field. J Phys Chem A. 2022;126:29–35. doi: 10.1021/acs.jpca.1c08452.
- Chen J, Yang H, Wang J, et al. Revealing the effect of the oriented external electronic field on the superatom-polymeric Zr3O3 cluster: Superhalogen modulation and spectroscopic characteristics. Spectrochim Acta A. 2020;237:118400. doi: 10.1016/j.saa.2020.118400.
- Chen J, Wei Q, Yang H, et al. On the structures, electronic properties, and superhalogen regulation of the MnB6¯ cluster: A density functional theory investigation. Chem Phys Lett. 2020;754:137723. doi: 10.1016/j.cplett.2020.137723.
- Chauhan V, Sahoo S, Khanna SN. Ni9Te6(PEt3)8C60 is a superatomic superalkali superparamagnetic cluster assembled material (S3-CAM). J Am Chem Soc. 2016;138:1916–1921. doi: 10.1021/jacs.5b10986.
- Chauhan V, Reber AC, Khanna SN. Strong lowering of ionization energy of metallic clusters by organic ligands without changing shell filling. Nat Commun. 2018;9:2357. doi: 10.1038/s41467-018-04799-0.
- Li J, Cui MW, Yang H, et al. Ligand-field regulated superalkali behavior of the aluminum-based clusters with distinct shell occupancy. Chin Chem Lett. 2022;33:5147–5151. doi: 10.1016/j.cclet.2022.02.039.
- Li J, Huang HC, Wang J, et al. Polymeric tungsten carbide nanoclusters: structural evolution, ligand modulation, and assembled nanomaterials. Nanoscale. 2019;11:19903–19911. doi: 10.1039/C9NR05613K.
- Liu GX, Pinkard A, Ciborowski SM, et al. Tuning the electronic properties of hexanuclear cobalt sulfide superatoms via ligand substitution. Chem Sci. 2019;10:1760–1766. doi: 10.1039/C8SC03862G.
- Liu GX, Chauhan V, Aydt AP, et al. Ligand effect on the electronic structure of cobalt sulfide clusters: A combined experimental and theoretical study. J Phys Chem C. 2019;123:25121–25127. doi: 10.1021/acs.jpcc.9b04153.
- Li J, Huang HC, Chen J, et al. Organic ligand mediated evolution from aluminum-based superalkalis to superatomic molecules and one-dimensional nanowires. Nano Res. 2022;15:1162–1170. doi: 10.1007/s12274-021-3619-1.
- Wang J, Zhao Y, Li J, et al. Unveiling the electronic structures and ligation effect of the superatom-polymeric zirconium oxide clusters: a computational study. Phys Chem Chem Phys. 2019;21:14865–14872. doi: 10.1039/C9CP01870K.
- Chauhan V, Reber AC, Khanna SN. Metal chalcogenide clusters with closed electronic shells and the electronic properties of alkalis and halogens. J Am Chem Soc. 2017;139:1871–1877. doi: 10.1021/jacs.6b09416.
- Chauhan V, Khanna SN. Strong effect of organic ligands on the electronic structure of metal-chalcogenide clusters. J Phys Chem A. 2018;122:6014–6020. doi: 10.1021/acs.jpca.8b03355.
- Reber AC, Khanna SN. The effect of chalcogen and metal on the electronic properties and stability of metal–chalcogenides clusters, TM6Xn(PH3)6 (TM = Mo, Cr, Re, Co, Ni; X = Se, Te; n = 8,5). Eur Phys J D. 2018;72:199. doi: 10.1140/epjd/e2018-90223-7.
- Wang H, Li J, Chen J, et al. Solvent field regulated superhalogen in pure and doped gold cluster anions. Chin Chem Lett. 2023;108222:108222. doi: 10.1016/j.cclet.2023.108222.
- Dong XX, Zhao Y, Li J, et al. Dual external field-engineered hyperhalogen. J Phys Chem Lett. 2022;13:3942–3948. doi: 10.1021/acs.jpclett.2c00916.
- Huang XY, Tang C, Li JQ, et al. Electric field–induced selective catalysis of single-molecule reaction. Sci Adv. 2019;5:eaaw3072. doi: 10.1126/sciadv.aaw3072.
- Chen HL, Jiang F, Hu C, et al. Electron-catalyzed dehydrogenation in a single-molecule junction. J Am Chem Soc. 2021;143:8476–8487. doi: 10.1021/jacs.1c03141.