ABSTRACT
Desiccation tolerance and longevity are two essential properties that enable seeds to ensure the plant life cycle. Desiccation tolerance is defined as the ability to tolerate a rapid drying that brings the seed moisture content in equilibrium with ambient air, whereas longevity is the capacity to remain alive in the dry state over long periods. Both properties are progressively acquired during seed development and are of considerable agronomic and ecological relevance. Here, we argue that desiccation tolerance and longevity should be considered and studied separately. Recent progress in elucidating the protective and regulatory mechanisms contributing to these two aspects of seed survival in the dry state is presented.
Desiccation tolerance and longevity: two components of seed persistence in the dry state that require further attention
As propagation material for the next progeny, seeds need to remain alive until conditions are favourable for germination and seedling establishment. After embryogenesis and seed filling, separation of the developing seeds from the mother plant at the end of maturation will inevitably result in the loss of water (Thompson Citation2018). One of the fascinating properties that nature developed to maintain seeds viable and tolerant to stress is desiccation tolerance. Desiccation tolerance is defined as the ability to survive a loss of water that rapidly brings the cellular moisture content in equilibration with ambient air (ie relative humidity). Generally, this moisture content corresponds to ca. 10% of the seed weight. The removal of water results in a dramatic increase in the viscosity of the cytoplasm, resulting in a decrease in the rate of detrimental reactions and leading to the arrest of metabolism, thereby minimizing production of reactive oxygen species (Buitink and Leprince Citation2004; ). In addition, in this immobilized state, dry seeds become tolerant to a wide range of stresses, including high temperature, freezing, anoxia or even very low atmospheric pressure.
Table 1. Molecular factors contributing to desiccation tolerance (ie water content threshold) and longevity (life span in the dry state). Putative factors that remain to be tested using direct evidence are highlighted in italics. ATM, ATAXIA TELANGIECTASIA MUTATED; HSP heat shock proteins; LEA; late embryogenesis abundant, LIG. DNA LIGASE; MSR: METHIONINE SULFOXIDE REDUCTASE; N.D., not detected; PMIT, PROTEIN l-ISOASPARTYL METHYLTRANSFERASE. For detailed information about these mechanisms, see Hoekstra, Golovina, and Buitink (Citation2001), Leprince et al. (Citation2017) and Sano et al. (Citation2016).
While desiccation-tolerant seeds are found in the vast majority of species, their lifespan in the dry state varies considerably between species, ranging from years to centuries during storage (Li and Pritchard Citation2009; Probert, Daws, and Hay Citation2009). This lifespan is referred to as longevity. Practically, longevity determines the storage potential of dry seeds that are held ex situ in dedicated seed banks to preserve genetic diversity. It is also used to characterize the performance at sowing of seed lots of field and vegetable crops (Finch-Savage and Bassel Citation2016). Direct measurements of seed longevity in seed bank conditions (eg 15% RH and −20°C) are impossible to obtain in real time. As an alternative, longevity is assessed by ageing seeds in warm and moist conditions to accelerate ageing. However, care has to be taken with extrapolating accelerated aging data to lower temperatures and drier atmosphere since it does not always predict longevities under these optimal conditions and more research is needed to understand the similarities and differences between both ageing conditions. Whereas the definition of desiccation tolerance implies a threshold value of moisture content during drying, longevity involves a time component that depends on the conditions of storage. This time component originates from the nature and speed of chemical and physical reactions that slowly deteriorate seeds during storage, despite extremely severe limitations in molecular diffusion and motion brought about by the glassy state (Ballesteros and Walters Citation2011; Buitink and Leprince Citation2004). Desiccation tolerance is first acquired during seed development (usually mid-way during seed filling), followed by the gradual increase in longevity until abscission and/or the dry state (Chatelain et al. Citation2012; Probert, Daws, and Hay Citation2009; Pereira Lima et al. Citation2017).
In a recent review (Leprince et al. Citation2017), we argued that the distinction between desiccation tolerance and longevity needs to be taken into account to gain further knowledge about the mechanisms controlling survival in the dry state. Such distinction raises not only new fundamental questions but has also practical implications. One could imagine that longevity is a quantitative way of expressing desiccation tolerance, and that similar protective molecules that govern desiccation tolerance continue to increase and could explain longevity in a more quantitative manner (). However, as outlined below, this view does not help understanding the range of longevities within desiccation-tolerant species and does not explain the impact of the environment on seed longevity. In an ecological context, longevity is now considered to have a different significance than desiccation tolerance. Variation in seed longevity across and within species is viewed either as a complex long-term mechanism of a plant population/species to adapt to local climates (Probert, Daws, and Hay Citation2009; Zani and Müller Citation2017) or as a plastic trait wherein the developing seed incorporates the parental growth environment as a germination strategy (Mondoni et al. Citation2014; Finch-Savage and Bassel Citation2016). From a practical point of view, the question as to whether the same protective mechanisms and regulatory genes govern desiccation tolerance and longevity is needed for the development of markers useful for gene banking to identify seeds that are desiccation-sensitive, or those that are desiccation-tolerant but can be stored only for a limited amount of time. It is also needed for biotechnological applications leading to lyopreservation of mammalian cells.
Establishment of desiccation tolerance and acquisition of longevity necessitate different regulatory pathways
During seed development, desiccation tolerance and longevity exist probably as two inter-dependent maturation programs. In Medicago truncatula Gaertn., desiccation tolerance is acquired at the same developmental age regardless of the growth conditions of the mother plant, including drought or heat stress conditions (); Righetti et al. Citation2015). In Arabidopsis, percentage of desiccation tolerance was not altered in seeds obtained from 13 different environments and 12 different genotypes whereas gene x environment interactions were found for many other seed traits such as dry weight and dormancy (He et al. Citation2014). Considering that the acquisition of desiccation tolerance is a prerequisite for the species dispersal, the robustness of this trait is probably not surprising since the dry quiescent state offers a stable state that is tolerant to environmental extremes. In contrast to desiccation tolerance, the acquisition of longevity varies according to the environment ()). Indeed, longevity is under the influence of environmental cues perceived by both the mother plant and zygote, suggesting that this trait exhibits phenotypic plasticity. Environmental cues include temperature, water availability, levels of nutrients, light, flooding, altitude (Ellis Citation1993; He et al. Citation2014; Righetti et al. Citation2015; Nagel et al. Citation2015; Contreras et al. Citation2008; Mondoni et al. Citation2014). During maturation, environment can increase or retard the acquisition of longevity, thereby inducing large variations in the interval between the end of seed filling and maximum shelf life (reviewed in Leprince et al. Citation2017). Longevity can also be modulated by post-harvest treatments before the seeds are totally dry (Whitehouse, Hay, and Ellis Citation2017; Whitehouse, Hay, and Ellis Citation2015; Probert et al. Citation2007).
Figure 1. Acquisition of desiccation tolerance (A) and longevity (B) in developing seeds of Medicago truncatula under two different growth conditions from flowering onwards: high temperature (26/24°C) (red triangles) and standard (20/18°C, black triangles). Longevity is expressed as P50, the time for 50% of the seed lot to loose viability during storage at 35°C and 75% relative humidity. Data are taken from Righetti et al. (Citation2015).
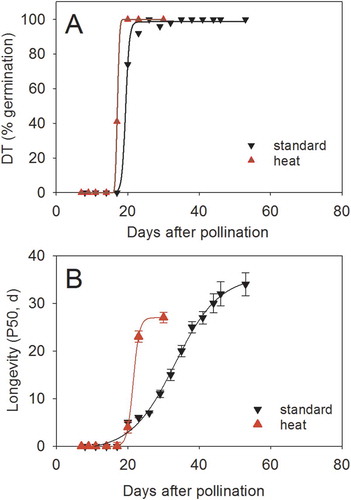
In recent years, underlying molecular pathways governing the desiccation tolerance and longevity programs during seed maturation were identified by the application of different – omics technologies and systems biology approaches. Progress was particularly made with Medicago truncatula because seeds of this legume species exhibit a long maturation phase, reaching full maturity three weeks after desiccation tolerance is acquired and during which longevity increases gradually over 30-fold (Chatelain et al. Citation2012; Verdier et al. Citation2013). This is in sharp contrast with other species such as Arabidopsis and soybean where longevity overlaps with seed filling (reviewed in Leprince et al. Citation2017; Pereira Lima et al. Citation2017). Detailed characterization of the accumulation of LEA proteins during seed maturation in M. truncatula identified distinct subgroups upon the acquisition of desiccation tolerance compared to the acquisition of longevity (Chatelain et al. Citation2012). Additional evidence of different programs for desiccation tolerance and longevity came from transcriptomic and metabolomic profiling together with conditional-dependent network models of global transcription interactions on M. truncatula (Verdier et al. Citation2013). The network revealed distinct co-expression modules related to the acquisition of desiccation tolerance and longevity (). The desiccation tolerance module was associated with abiotic stress responsive genes, whereas the longevity module was enriched in genes involved in RNA processing and translation. This approach was taken a step further by the incorporation of the environmental effects during seed maturation on the acquisition of both traits (Righetti et al. Citation2015) (). The gene module linked to desiccation tolerance was comparable to that previously found by Verdier et al. (Citation2013). About 85% of the genes present in this module were found to be deregulated in abi3 mutants, suggesting that this desiccation tolerance module is mainly under the regulation of ABI3 (Righetti et al. Citation2015; ). Incorporation of the plasticity of longevity in the gene network lead to the identification of a tightly regulated gene module correlating with longevity and highly conserved in Arabidopsis seeds. Interestingly, less than half of the genes present is this module were found to be downstream of ABI3, suggesting that these genes are regulated via alternative pathways that are not part of the ABI3 pathway (Righetti et al. Citation2015).
Figure 2. Gene regulatory network analysis during seed development of M. truncatula reveals two modules associated respectively with desiccation tolerance (DT, green) and longevity (P50, blue). Within each module, large squares represent transcription factors and circles, other hub genes. The upper network represents genes that are confirmed targets of MtABI3 whereas the bottom network corresponds to genes that are not regulated by MtABI3. Data are from Righetti et al. (Citation2015). EMB, embryogenesis. WC, water content.
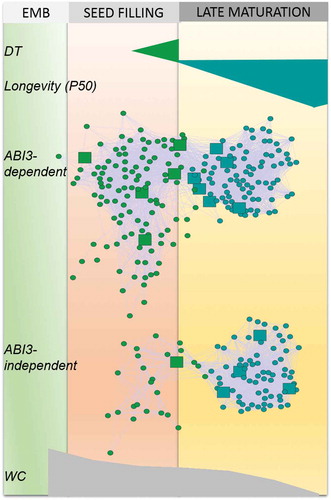
Longevity evolved by co-opting existing pathways regulating plant immunity
Intriguingly, the longevity module is highly enriched with GO categories related to biotic stress defence (Righetti et al. Citation2015). Mutant seeds defective in the two biotic stress-related transcription factors WRKY3 and NFXL1 (nuclear factor-X1-like1) present in this module exhibited reduced longevity. Furthermore, in the wrky3 seeds, WRK33, a regulator of jasmonate-related defence pathway was also downregulated (Righetti et al. Citation2015). Seeds of these two mutants displayed weakened physical barrier reflected by increased seed coat permeability. Other molecular players in plant immunity have been shown to be implicated in longevity. For example, the seed–specific regulatory subunit SNF4b of the SnRK1 complex plays a role in plant defence in dormant seeds of M. truncatula and was also shown to be involved in longevity (Bolingue et al. Citation2010). Mechanisms involved in passive defence also include physical barriers related to thickened cell walls, or substances such as cutin, suberin, or pectin. Interestingly, seeds of Arabidopsis mutants with defects in cutin deposition exhibited reduced longevity (De Giorgi et al. Citation2015). Defects in the testa structure and composition such as flavonoid pigmentation are known to affect seed storability (reviewed in Sano et al. Citation2016) and these components have also an important role in defence against pathogens. All these observations point to a link between developmental programs regulating (late) seed maturation, longevity and defence. Such a link was also illustrated by the observation that developing M. truncatula seeds responded to the presence of xanthomonads, a seed-borne pathogen, leading to a temporary delay in seed maturation (Terrasson et al. Citation2015). Thus the expression of defence-related components during seed maturation could be a programmed mechanism to install passive barriers as a pre-emptive strategy to protect the embryos from pathogen attack as in the dry quiescent state the seed will not be able to activate defence responses. In turn, these barriers will also influence the longevity by restricting water and oxygen diffusion to the embryo, two factors contributing to accelerated ageing during storage (, Sano et al. Citation2016).
Further work on the protective and regulatory mechanisms contributing to desiccation tolerance and longevity is necessary, keeping in mind the robustness of the former and the plasticity of the latter. Further analysis of these two maturation programs will be difficult in species where synthesis of storage reserves overlap with the acquisition of longevity, such as Arabidopsis. While ABA plays a key role in inducing both DT and longevity programs, the question as to whether other hormones and signalling pathways intervene as well remains to be investigated. Finally, whether seed longevity evolved by co-opting existing pathways regulating the activation of defence against pathogens to ensure proper protection during storage in the soil warrants investigations.
Disclosure statement
No potential conflict of interest was reported by the authors.
Additional information
Funding
Notes on contributors
Julia Buitink
Julia Buitink is Research Director at INRA in France. She is specialized in seed biology, with a special interest in system biology approaches to understand seed survival in the dry state.
Contribution: She wrote the article and contributed to the figures.
Olivier Leprince
Olivier Leprince is Professor of Plant Physiology at Agrocampus Ouest in France. His research interest is related to seed stability in the dry state, the impact of abiotic stress on seed quality and longevity in crops and wild species.
Contribution: He contributed to the writing of the manuscript and design of the figures.
References
- Ballesteros, D. , and C. Walters . 2011. “Detailed Characterization of Mechanical Properties and Molecular Mobility within Dry Seed Glasses: Relevance to the Physiology of Dry Biological Systems.” The Plant Journal 68 (4): 607–619. doi:10.1111/j.1365-313X.2011.04711.x.
- Bolingue, W. , C. Rosnoblet , O. Leprince , B. L. Vu , C. Aubry , and J. Buitink . 2010. “The MtSNF4b Subunit of the Sucrose Non-Fermenting-Related Kinase Complex Connects After-Ripening and Constitutive Defense Responses in Seeds of Medicago truncatula .” Plant Journal 61 (5): 792–803. doi:10.1111/j.1365-313X.2009.04106.x.
- Buitink, J. , and O. Leprince . 2004. “Glass Formation in Plant Anhydrobiotes: Survival in the Dry State.” Cryobiology 48 (3): 215–228. doi:10.1016/j.cryobiol.2004.02.011.
- Chatelain, E. , M. Hundertmark , O. Leprince , S. Le Gall , P. Satour , S. Deligny-Penninck , H. Rogniaux , and J. Buitink . 2012. “Temporal Profiling of the Heat-Stable Proteome during Late Maturation of Medicago truncatula Seeds Identifies a Restricted Subset of Late Embryogenesis Abundant Proteins Associated with Longevity.” Plant, Cell & Environment 35 (8): 1440–1455. doi:10.1111/j.1365-3040.2012.02501.x.
- Contreras, S. , M. A. Bennett , J. D. Metzger , and D. Tay . 2008. “Maternal Light Environment during Seed Development Affects Lettuce Seed Weight, Germinability, and Storability.” HortScience 43 (3): 845–852.
- De Giorgi, J. , U. Piskurewicz , S. Loubery , A. Utz-Pugin , C. Bailly , L. Mène-Saffrané , and L. Lopez-Molina . 2015. “An Endosperm-Associated Cuticle Is Required for Arabidopsis Seed Viability, Dormancy and Early Control of Germination.” PLOS Genetics 11 (12): e1005708. doi:10.1371/journal.pgen.1005708.
- Ellis, R. 1993. “Seed Production Environment, Time of Harvest, and the Potential Longevity of Seeds of Three Cultivars of Rice (Oryza sativa L.).” Annals of Botany 72 (6): 583–590. doi:10.1006/anbo.1993.1148.
- Finch-Savage, W. E. , and G. W. Bassel . 2016. “Seed Vigour and Crop Establishment: Extending Performance beyond Adaptation.” Journal of Experimental Botany 67 (3): 567–591. doi:10.1093/jxb/erv490.
- He, H. , D. De Souza Vidigal , L. B. Snoek , S. Schnabel , H. Nijveen , H. Hilhorst , and L. Bentsink . 2014. “Interaction between Parental Environment and Genotype Affects Plant and Seed Performance in Arabidopsis .” Journal of Experimental Botany 65 (22): 6603–6615. doi:10.1093/jxb/eru378.
- Hoekstra, F. A. , E. A. Golovina , and J. Buitink . 2001. “Mechanisms of Plant Desiccation Tolerance.” Trends iHn Plant Science 6 (9): 431–438. doi:10.1016/S1360-1385(01)02052-0.
- Leprince, O. , A. Pellizzaro , S. Berriri , and J. Buitink . 2017. “Late Seed Maturation: Drying without Dying.” Journal of Experimental Botany 68 (4): 827–841.
- Li, D. Z. , and H. W. Pritchard . 2009. “The Science and Economics of Ex Situ Plant Conservation.” Trends in Plant Science 14 (11): 614–621. doi:10.1016/j.tplants.2009.09.005.
- Mondoni, A. , S. Orsenigo , M. Donà , A. Balestrazzi , R. J. Probert , F. R. Hay , A. Petraglia , and T. Abeli . 2014. “Environmentally Induced Transgenerational Changes in Seed Longevity: Maternal and Genetic Influence.” Annals of Botany 113 (7): 1257–1263. doi:10.1093/aob/mcu046.
- Nagel, M. , I. Kranner , K. Neumann , H. Rolletschek , C. E. Seal , L. Colville , B. Fernández-Marín , and A. Börner . 2015. “Genome-Wide Association Mapping and Biochemical Markers Reveal that Seed Ageing and Longevity are Intricately Affected by Genetic Background and Developmental and Environmental Conditions in Barley.” Plant, Cell & Environment 38 (6): 1011–1022. doi:10.1111/pce.12474.
- Pereira Lima, J. J. , J. Buitink , D. Lalanne , R. F. Rossi , S. Pelletier , E. A. Da Silva , and O. Leprince . 2017. “Molecular Characterization of the Acquisition of Longevity during Seed Maturation in Soybean.” Plos One 12: 7. doi:10.1371/journal.pone.0180282.
- Probert, R. , J. Adams , J. Coneybeer , A. Crawford , and F. Hay . 2007. “Seed Quality for Conservation Is Critically Affected by Pre-Storage Factors.” Australian Journal of Botany 55 (3): 326–335. doi:10.1071/BT06046.
- Probert, R. J. , M. I. Daws , and F. R. Hay . 2009. “Ecological Correlates of ex situ Seed Longevity: A Comparative Study on 195 Species.” Annals of Botany 104 (1): 57–69. doi:10.1093/aob/mcp082.
- Righetti, K. , J. L. Vu , S. Pelletier , B. L. Vu , E. Glaab , D. Lalanne , A. Pasha , and J. Buitink . 2015. “Inference of Longevity-Related Genes from a Robust Coexpression Network of Seed Maturation Identifies Regulators Linking Seed Storability to Biotic Defense-Related Pathways.” Plant Cell 27 (10): 2692–2708.
- Sano, N. , L. Rajjou , H. M. North , I. Debeaujon , A. Marion-Poll , and M. Seo . 2016. “Staying Alive: Molecular Aspects of Seed Longevity.” Plant and Cell Physiology 57 (4): 660–674. doi:10.1093/pcp/pcv186.
- Terrasson, E. , A. Darrasse , K. Righetti , J. Buitink , D. Lalanne , B. Ly Vu, S. Pelletier, W. Bolingue, M. A. Jacques, and O. Leprince. 2015. “Identification of a Molecular Dialogue Between Developing Seeds of Medicago truncatula and Seedborne Xanthomonads.” Journal of Experimental Botany 66: 3737 –3752.
- Thompson, R. 2018. “Letters to the Twenty-First Century Botanist. Second Series: “What Is a Seed? – 1.” Botany Letters 165 (1): 3–5. doi:10.1080/23818107.2017.1413998.
- Verdier, J. , D. Lalanne , S. Pelletier , I. Torres-Jerez , K. Righetti , K. Bandyopadhyay , O. Leprince , and J. Buitink . 2013. “A Regulatory Network-Based Approach Dissects Late Maturation Processes Related to the Acquisition of Desiccation Tolerance and Longevity of Medicago truncatula Seeds.” Plant Physiology 163 (2): 757–774. doi:10.1104/pp.113.222380.
- Whitehouse, K. J. , F. R. Hay , and R. H. Ellis . 2015. “Increases in the Longevity of Desiccation-Phase Developing Rice Seeds: Response to High-Temperature Drying Depends on Harvest Moisture Content.” Annals of Botany 116 (2): 247–259. doi:10.1093/aob/mcv091.
- Whitehouse, K. J. , F. R. Hay , and R. H. Ellis . 2017. “High-Temperature Stress during Drying Improves Subsequent Rice (Oryza sativa L.) Seed Longevity.” Seed Science Research 27 (4): 281–291. doi:10.1017/S0960258517000277.
- Zani, D. , and J. V. Müller . 2017. “Climatic Control of Seed Longevity of Silene during the Post-Zygotic Phase: Do Seeds from Warm, Dry Climates Possess Higher Maturity and Desiccation Tolerance than Seeds from Cold, Wet Climates?” Ecological Research 32 (6): 983–994. doi:10.1007/s11284-017-1508-6.