Abstract
Induced hypothermia may protect from ischemia reperfusion injury. The mechanism of protection is not fully understood and may include an effect on mitochondria. Here we describe the effect of hypothermia on circulating mitochondrial (mt) DNA in a substudy of a multicenter randomized trial (the Target Temperature Management trial). Circulating levels of mtDNA were elevated in patients with cardiac arrest at all-time points compared to healthy controls. After 24 h of temperature management, patients kept at 33 °C had significantly lower levels of COX3, NADH1 and NADH2 compared to baseline, in contrast to those kept at 36 °C. After regain of temperature, cytochrome – B was significantly reduced in patients kept at 33 °C with cardiac arrest. Cardiac arrest results in circulating mtDNA levels, which reduced during a temperature management protocol in patients with a target temperature of 33 °C.
Introduction
Induced hypothermia is thought to limit tissue injury following ischemia-reperfusion and is applied in operative procedures in which the aorta needs to be clamped (Polderman Citation2009). The mechanisms of action are not fully known, but may include an effect on mitochondria. When oxygen delivery to tissue is compromised, apoptotic pathways are activated. Mitochondrial (mt) DNA released from necrotic tissue was found to drive the systemic inflammatory response syndrome (SIRS) in patients with tissue damage due to traumatic injury (Zhang et al. Citation2010). In cardiac arrest, mitochondrial dysfunction was found to be present in a rat model (Han et al. Citation2008). Also, mtDNA is a possible predictor of adverse outcome of patients (Arnalich et al. Citation2012) and is thought to be a marker for hypoxic tissue damage.
Notably, in animal models of ischemia-reperfusion injury, hypothermia was recently found to reduce the production of reactive oxygen species, with a concomitant improvement in mitochondrial function in the heart (Tissier et al. Citation2012) and in the brain (Gong et al. Citation2012). These findings suggest a central role for mitochondria in the pathophysiology of organ damage after cardiac arrest and may point towards the mechanism of action of the beneficial effect of hypothermia in ischemia-reperfusion injury.
In the present study, we investigated the effect of induced hypothermia on circulating cell-free mtDNA in a post hoc single centre analysis of a subgroup of patients randomized to a trial controlling for body temperature (Nielsen et al. Citation2013).
Methods
Included patients were part of the Target Temperature Management (TTM) trial, which is an open-label multicentre trial in which patients were randomized to 33 °C or 36 °C following an out-of-hospital cardiac arrest (Gong et al. Citation2012). Analysis was planned prior to disclosure of results of the TTM trial. Eligible patients were >18 years of age, had suffered an out-of-hospital witnessed cardiac arrest with a presumed myocardial cause, had regained spontaneous circulation (ROSC), were admitted to the medical–surgical intensive care unit of a university hospital in Amsterdam, the Netherlands, with a Glasgow coma score ≤8 and for whom informed consent was obtained from the next of kin. Exclusion criteria were pregnancy, more than 4 h between ROSC and screening, cardiogenic shock and spontaneous hypothermia of <30 °C on admission. For sampling, patients were consecutively included between October 2011 and October 2012 and randomized using a web–based tool. Hypothermia was induced by intravenous infusion of ice cold Ringers lactate (4 °C, 100 ml/min) and with a cold mattress. Hypothermia was maintained during 24 h, after which the patients were actively rewarmed. Controls were kept at 36 °C. Temperature was measured using a bladder thermometer. All the patients received sedation with propofol for 24 h. Shivering was treated with neuromuscular blocking agents. Other treatment consisted of percutaneous coronary intervention on suspicion of myocardial ischemia and either thrombosis prophylaxis or anticoagulant medication as deemed appropriate. Norepinephrine was infused to maintain a minimum mean arterial pressure of 65 mmHg. All the patients received selective oropharyngeal decontamination. Blood from an arterial catheter was drawn at baseline, 24 h later and when body temperature had returned to 36 °C in the 33 °C group. In the 36 °C group, blood was drawn at baseline and after 24 h. Blood was also drawn from healthy volunteers (n = 5) of 30–34 years of age. The National Intensive Care Evaluation (NICE) minimal dataset prospectively collects data to calculate the Acute Physiology and Chronic Health Evaluation (APACHE II) score. Other data were collected from the electronic patient data monitoring system. Blood was centrifuged within 10 min of collection at 600 g, during 10 min at 4 °C and supernatant was stored at –80 for further analysis.
DNA isolation and PCR
Total DNA was isolated in 200 μL plasma with Qiamp DNA kit (Qiagen, Venlo, Netherlands). DNA levels were measured by spectrophotometer and stored at –80. The expression of COX3 (forward: ATGACCCACCAATCACATGC, reverse: ATCAC-ATGGCTAGGCCGGAG), NADH1 (forward: ATACCCATGGCCAACCTCCT, reverse: GGGCCTTTGCGTAGTTGTAT), NADH2 (forward: CTCACATGACAAAAACTAGCCCCCA, reverse: TCCACCTCAACTGCCTGCTATGA) and cytochrome–B (forward: ATG-ACCCCAATACGCAAAAT, reverse: CGAAGTTTCATCATGCGGAG) were analyzed by reverse-transcription-polymerase chain reactions (RT-PCRs) using lightCycler® SYBR green I master mix (Roche, Mijdrecht, the Netherlands) and measured in a LightCycler® 480 (Roche) apparatus using the following conditions: 5 min 95° C hot-start, followed by 40 cycles of amplification (95 °C for 10 s, 60 °C for 5 s, 72 °C for 15 s). Standard curves were constructed on serial dilutions of a concentrated complementary DNA sample for quantifications and presented as arbitrary unit (AU). The intra-assay was zero, as all the samples from the groups were measured on the same 360 wells plate.
Statistical analysis
Data are expressed as mean with SD. Differences between healthy subjects and patients and 33 °C vs. 36 °C were analyzed using a Student’s T-test or Mann–Whitney U test according to data distribution. To determine changes occurring over time within a group, a paired T-test was used. A p value of < .05 was considered statistically significant (Graphpad Prism 5, San Diego, CA).
Results
In total 20 patients were included in this study, of whom 13 were enrolled in the 33 °C group while in the remaining 7 patients, body temperature was kept at 36 °C. There were no substantial differences in demographic data between these groups (), nor in APACHE score, cumulative norepinephrine infusion, CKMB levels, troponin – T levels and time to ROSC. Cumulative fluid balance tended to be higher in the 36 °C group. At ICU discharge, no difference in mortality was observed between the groups. None of the included patients received blood transfusions before and during the study.
Table 1. Characteristics of patients following cardiac arrest.
There were no baseline differences between the 33 °C and 36 °C patients (). Circulating levels of mtDNA were increased in patients after cardiac arrest compared to healthy controls (). After 24 h, treatment with 33 °C was associated with a relative reduction in levels of COX3 and NADH1, NADH2 compared to baseline (, p < .05), whereas levels in the 36 °C group did not change. At the end of the protocol, when 33 °C patients had regained normal body temperature and in the 36 °C patients, cytochrome B levels were lower compared to baseline in both groups, with significantly lower levels in the patients treated with 33 °C compared to patients in whom body temperature was kept at 36 °C.
Figure 1. Circulating levels of COX3, NADH1, NADH2 and cytochrome (cyto) B in patients following out of hospital cardiac arrest and in healthy controls. *p < .05 between patients and controls.
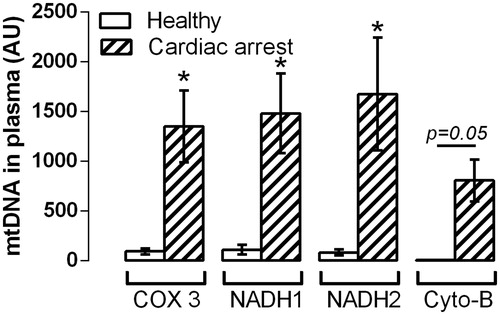
Figure 2. Change in circulating levels of COX3, NADH1, NADH2 and cytochrome (cyto) B relative to baseline (set at 100%) in cardiac arrest patients after 24 h of normo- or hypo-temperature (termed ‘24 h’) and when body temperature had returned to normal in patients with cardiac arrest cooled down to 32 °C (n = 10) and in normothermic controls (n = 6) (termed ‘regain of temp’). *p < .05.
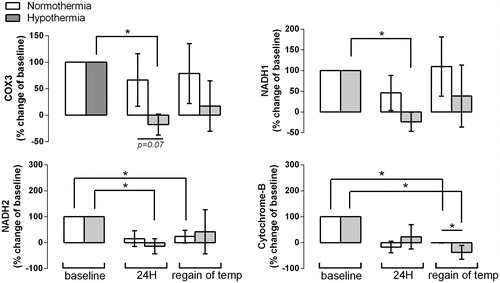
Discussion
Elevated circulating levels of mtDNA have been found before in cardiac arrest patients, which correlated with outcome (Arnalich et al. Citation2012). Here, we confirm that mtDNA levels are increased in cardiac arrest patients. We expand these findings by showing that levels of COX-3 and NADH-1 are reduced in patients kept at a target temperature of 33 °C compared to those kept at 36 °C.
In patients with elevated levels of mtDNA, SIRS with organ failure can develop in up to 30%, which can be clinically indistinguishable from bacterial sepsis (Lenz et al. Citation2007). Mitochondria are thought to be descendants of bacteria, engulfed and locked up by host cells. Thereby, when outside the cell, parts of the mitochondrial molecules which resemble bacterial molecular patterns, including mtDNA, may trigger an immune response by binding to toll like receptors (Zhang et al. Citation2010). These units are part of the mitochondrial respiratory chain complexes, and are involved in generating ATP. Of note, these markers are encoded in the mitochondrial genome and therefore can be recognized as ‘foreign’ molecules (Zhang et al. Citation2010) and thereby may induce SIRS. NADH2 did not differ between temperature groups.
Compared to baseline, levels of all markers of mtDNA following cardiac arrest were lower after 24 h, in particular, of NADH2 and cytochrome B. However, the differences between groups at 24 h suggest that induced hypothermia exerts effects which are independent of changes in time. We propose that reduction of mtDNA levels may be a mechanism of the observed beneficial effects of induced hypothermia in inhibiting the inflammatory response in animal models (2;6). Results also demonstrate a role for mitochondria in the pathogenesis of hypoxic tissue injury.
The clinical implication of our findings is uncertain, as the TTM trial did not show a survival benefit in cardiac arrest patients with body temperature kept at 33 °C compared to those kept at 36 °C (Nielsen et al. Citation2013). However, the therapeutic potential of hypothermia in these patients remains subject of research in preclinical setting. For instance, hypothermia exerts its neuroprotective role by inhibiting mitochondrial apoptotic pathway in cardiac arrest model (Wu et al. Citation2016).
The small number has obvious limitations to our findings. We cannot exclude the role of chance in our findings. Also, healthy controls were younger than the patients.
Conclusions
Circulating levels of mtDNA were reduced in patients with an out-of-hospital cardiac arrest with a target temperature of 33 °C but not in patients with a target temperature of 36 °C. Prevention of mitochondrial damage and release of mtDNA may play a role in the beneficial effect of hypothermia during ischemia-reperfusion injury as observed in preclinical models.
Disclosure statement
The authors report no conflicts of interest. The authors alone are responsible for the content and writing of this article.
References
- Arnalich F, Codoceo R, Lopez-Collazo E, Montiel C. 2012. Circulating cell-free mitochondrial DNA: a better early prognostic marker in patients with out-of-hospital cardiac arrest. Resuscitation. 83:e162–e163.
- Gong P, Li CS, Hua R, Zhao H, Tang ZR, Mei X, Zhang MY, Cui J. 2012. Mild hypothermia attenuates mitochondrial oxidative stress by protecting respiratory enzymes and upregulating MnSOD in a pig model of cardiac arrest. PLoS One. 7:e35313.
- Han F, Da T, Riobo NA, Becker LB. 2008. Early mitochondrial dysfunction in electron transfer activity and reactive oxygen species generation after cardiac arrest. Crit Care Med. 36:S447–S453.
- Lenz A, Franklin GA, Cheadle WG. 2007. Systemic inflammation after trauma. Injury. 38:1336–1345.
- Nielsen N, Wetterslev J, Cronberg T, Erlinge D, Gasche Y, Hassager C, Horn J, Hovdenes J, Kjaergaard J, Kuiper M, et al. 2013. Targeted temperature management at 33 degrees C versus 36 degrees C after cardiac arrest. N Engl J Med. 369:2197–2206.
- Polderman KH. 2009. Mechanisms of action, physiological effects, and complications of hypothermia. Crit Care Med. 37:S186–S202.
- Tissier R, Chenoune M, Pons S, Zini R, Darbera L, Lidouren F, Ghaleh B, Berdeaux A, Morin D. 2012. Mild hypothermia reduces per-ischemic reactive oxygen species production and preserves mitochondrial respiratory complexes. Resuscitation. 84:249–255.
- Wu L, Sun HL, Gao Y, Hui KL, Xu MM, Zhong H, Duan ML. 2016. Therapeutic hypothermia enhances cold-inducible RNA-binding protein expression and inhibits mitochondrial apoptosis in a rat model of cardiac arrest. Mol Neurobiol. [cited 2016 Mar 19]. doi:10.1007/s12035-016-9813-6
- Zhang Q, Raoof M, Chen Y, Sumi Y, Sursal T, Junger W, Brohi K, Itagaki K, Hauser CJ. 2010. Circulating mitochondrial DAMPs cause inflammatory responses to injury. Nature. 464:104–107.