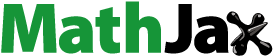
Abstract
This article presents a rapid, costless, and environment-friendly technique for the biosynthesis of ultra-small ZnO nanoparticles (NPs), which were synthesized using espresso spent coffee grounds (SCG). The synthesized ZnO NPs were determined by Field-emission scanning electron microscopy (FESEM), X-ray diffraction (XRD), and Fourier-transform infrared (FT-IR). The photocatalytic activity of the ZnO NPs was evaluated for photodegradation of tartrazine (Tz) dye under UV light. The response surface method (RSM) in a central composite rotatable design (CCRD) was used to design the experiments. The parameters affecting the efficiency of the degradation were evaluated and optimized. The ZnO NPs were assessed for antimicrobial and antioxidant activities. The results showed that XRD, FESEM, and FT-IR confirmed the formation of ZnO NPs. At optimized values, high removal efficiencies (92.5%) were found. Significant antioxidant activity of the NPs was demonstrated using a DPPH (2, 2-diphenyl-1-picrylhydrazyl) free radical assay with the IC50 value of 0.9586 mg/mL.
Introduction
Nowadays organic dyes are considered one of the resources of water pollution. Numerous industries like plastics, pharmaceutical, leather, textile, printing, cosmetic, rubber, paper, and food industries regularly use different dyes in several processes and frequently release excessive volumes of dye comprising waste into the environment.[Citation1] It has been reported that dyes in wastewater cause serious diseases in living beings such as kidney and liver damage, skin irritation, central nervous system poisoning, blood disorders, mutagenic, and carcinogenic effects. Moreover, the existence of a large number of dyes in wastewater both absorbs and reflects rays of the sun and makes photosynthesis difficult.[Citation2,Citation3]
One of the coloring agents generally used in food resources, cosmetics, shells of medicinal capsules, sirups, and vitamins are Tz. Tz (C16H9N4Na3O9S2) is an anionic dye that discharges anions in an aqueous environment. Although it is used in several kinds of food materials, its higher volume can possess carcinogenic and mutagenic repercussions. Thyroid, cancer, migraine, asthma, hypersensitivity, itching, blurred vision, and skin eczema are some of the diseases caused by breathing too much Tz. Therefore, due to the adverse effects and toxicity problems of dyes, an eco-friendly and cost-effective method for removing them from wastewater before discharging them into the environment should be taken into account.[Citation4]
A large number of methods have been applied for the removal of dyes from wastewater such as membrane filtration, biodegradation, adsorption, sedimentation, coagulation, catalytic degradation, and flocculation.[Citation5] One of the most efficient strategies for wastewater treatment is photocatalysis, particularly heterogeneous photocatalysis.[Citation6] This approach is considered one of the cutting-edge oxidation processes since the exploration of photoelectrochemical water splitting reaction based on the semiconductor. In recent decades, scientists have focused on photocatalysts due to their proven capability to destroy organic dyes.[Citation7] Various NPs are considered to remove synthetic dyes from aqueous solutions, such as titanium dioxide, iron oxide, zinc oxide, metal-organic framework, carbon nanotubes, chitosan, cellulose, and so forth.[Citation3, Citation8–12] ZnO NPs are one of the best semiconducting materials because of their nontoxicity, chemical stability, photo-catalytic ability, thermal stability, and higher decomposition rates for biological contaminants due to their higher optical properties in comparison with other semiconductors.[Citation13] Several approaches were described for the synthesis of ZnO NPs like microwave, precipitation, hydrothermal, microemulsion, supercritical water, mechanical techniques, and solvothermal.[Citation14] ZnO NPs are significantly concentrated on nanocatalysts. Semiconductors have turned into a fundamental ground for the photodegradation of wastewater treatment. Many researchers pay attention to the green synthesis method of ZnO NPs using diverse plant extracts.[Citation15] According to a recent review, ZnO NPs are important in medical applications particularly they are the best choice for drug delivery, wound healing, antioxidant, and antibacterial activities. All in all, using environmental-friendly chemicals in green synthesis is a fascinating field, which may offer solutions to industrial and therapeutic applications.[Citation16,Citation17]
One of the most crucial and consuming agricultural products in the world is coffee. The major solid by-products of the farming and preparing coffee are by-products of coffee fruit (coffee cherry), bean processing (coffee husks, peel, and pulp), and spent coffee grounds.[Citation18] SCG are the vital waste of coffee powder extracted by hot water and soluble coffee industry in restaurants, coffee shops, or at home. Around 0.91 g of the SCG are gained per 1 g of ground coffee, and around 2 kg of wet SCG are obtained for each kilogram of instant coffee. Disposal of these residues into the environment makes hazardous pollution because they include a great number of organic matters like polyphenols and caffeine.[Citation19] This problem has led to efforts to reuse and convert this residue into valuable products such as substrate to support the growth of carotenogenic yeasts strains and fungal species, animal feed, coextraction of oil, and diterpenes, adsorbent for the reduction of water-soluble dyes or heavy metals from wastewater, and raw-material for ethanol and biofuel production.[Citation20] Another attractive alternative method is microencapsulation of bioactive compounds from SCG which can potentially use in the pharmaceutical industries due to their safety compared with synthetic compounds. In addition to this, some studies showed that SCG consists of numerous domains of chemicals related to health such as melanoidins, methylxanthines, and phenolic compounds that show anti-allergic, antioxidant, anti-inflammatory, and anti-tumoral properties.[Citation21]
The major sections of the phenolic compounds are caffeic acids and chlorogenic which can use as natural antioxidants in cosmetic, pharmaceutical, feed-stock, and food industries. One of the fascinating properties of methylxanthines is the stimulation of the central nervous system. Their significance in cancer diseases has been related to the antagonism of adenosine receptors. Caffeine is the main methylxanthine retrieved from SCG which can be used in the production of energy drinks or dermatological preparation for cellulite, and chewing gum,[Citation22] whereas theobromine and theophylline can be applied by the pharmaceutical industry.[Citation23] SCG comprises great amounts of valuable organic materials such as cellulose, lignin, hemicellulose, fatty acids, proteins, and antioxidants that can be used as a basis for value-added products.[Citation24] Hence, the existence of polyphenols in SCG provides appropriate reduction properties for it, which makes it a tremendous reducing agent for the green method for synthesizing NPs. Moreover, SCG polyphenols are regarded as being nontoxic that are safe for the environment.[Citation25]
In previous studies, ZnO NPs were synthesized using potato and vegetable waste and their application to the removal of synthetic dyes from aqueous solutions.[Citation26,Citation27] To the best of the authors’ knowledge, however, the green method for synthesizing ZnO NPs using SCG has not been investigated. Hence, in this study, the outstanding catalytic performance of biosynthesized ZnO NPs was performed for the degradation of Tz. Managing and control of organic dye pollutants in wastewater is a thoughtful matter in most industries. It seems that a prominent solution to this problem is the facile green synthesis of ZnO NPs as a photocatalyst for wastewater treatment. The impact of factors such as photocatalyst dose, dye concentrations, and irradiation time on the degradation efficiency was considered. Furthermore, the antioxidant and antibacterial activities of ZnO NPs were also determined against two standard strains of Escherichia coli, and Staphylococcus aureus.
Materials and methods
Materials and reagents
Espresso SCG were obtained as a by-product from a local coffee bar in Tehran (Iran). The SCG were dried at 50 °C for 48 h and then stored at 25 °C for further experiments.[Citation28] Tz (analytical standard) and all other reagents and chemicals were obtained from Sigma-Aldrich (St Louis, MO, USA) and they were of analytical grade.
SCG extract preparation
To prepare the SCG extract, 6 g of dried SCG was mixed in 150 mL of deionized water in a 500 mL flask, and the pH was adjusted to 12.0 with sodium hydroxide (1 M). The sample was mixed well on a magnetic stirrer at room temperature for 15 min. The mixture was subjected to extraction by using a microwave instrument (MicroSYNTH, Milestone, Italy) at 100 °C and 800 W of power for 5 min. The obtained sample was then centrifuged for 20 min at 4500 rpm and 4 °C to recover the bioactive compounds (mostly polysaccharides and polyphenols). The solid was discarded and the SCG extract was kept at −18 °C until further experiments.[Citation29]
Synthesis of ZnO NPs
The ZnO NPs were synthesized with some modifications as described earlier.[Citation30] For the synthesis of ZnO/Zn-NPs, a total of 12.5 mL of SCG extract was mixed with 37.5 mL of distilled water and adjusted to pH 12.0 by the addition of sodium hydroxide (1 M) solution. Then, this mixture was added dropwise to 10 mL of 0.37 M zinc chloride aqueous solution and was stirred for 20 min at room temperature. Afterward, the mixture was subjected to microwave irradiation (MicroSYNTH, Milestone, Italy) at 100 °C and 800 W of power for 5 min. Finally, the solid was separated by centrifugation and calcined at 550 °C for 2 h in a Muffle furnace (muffle furnace, Carbolite CWF, 1100) by maintaining a heating rate of 10 °C min−1 to get NPs.
Characterization of the ZnO NPs
The XRD patterns of the NPs were evaluated using a Seifert-3003 PTS (Germany) diffractometer with Cu (Kα) radiation (wavelength: 1.5406 Å) at 40 kV and 30 mA in the range of 2θ from 5 to 80°. The FT-IR spectra of samples were recorded at room temperature in the 4000–400 cm−1 range (Thermo Nicolet, Nexus 870, USA FTIR) with the KBr pellet method. The morphology of photocatalysts was investigated with FESEM (MIRA3 TESCAN-XMU, Czech Republic).[Citation31]
Photocatalytic degradation of Tz dye
The efficiency of the ZnO NPs for the photocatalytic degradation of Tz dye was measured in an aqueous medium. For this purpose, the photocatalysis experiment was evaluated by keeping reaction conditions as 0.02–0.05 g of photocatalyst dose, 100 mL of 30–90 mg/L Tz concentration, and 35–120 min of UV light (30 W) irradiation time. The pH of the dye solution was adjusted to 6.0. During the contact time, aliquots of 4.0 mL were withdrawn in known intervals and centrifuged at 4000 rpm for 10 min. Then, the supernatants were filtered through 0.45 μm Millipore® membranes and analyzed in the wavelength range of 200–800 nm using a UV–vis spectrophotometer (Cary 100, Varian, Australia). The percent degradation of the Tz dye was calculated using EquationEq. (1)(1)
(1) :
(1)
(1)
where “Co” and “C” are the initial and equilibrium concentration of Tz dye (mg/L), respectively.
RSM modeling
The CCRD and analysis of 3D surfaces via the RSM method were used to explore the interaction between studied variables and optimize the effect of the factors to enhance the photocatalytic activity of ZnO NPs. In this design, the independent variables were A, B, and C, corresponding to ZnO NPs dose (g), initial Tz concentration (mg/L), and contact time (min), respectively, whereas the response is Tz dye removal (%) (see EquationEq. (1)(1)
(1) ). The CCRD contained a full-factorial 23 (eight factorial points), six replicates, and six axial points with rotatable alpha, totaling 20 experiments. The independent variables were measured in five coded levels: −1.68; −1; 0; +1 and +1.68. The ranges and levels of independent variables studied are shown in . The CCRD and data processing were applied using Design Expert 13 software.
Table 1. Experimental ranges and levels of the selected variables for CCRD.
Antioxidant activity
The antioxidant activity of ZnO NPs was performed with some modifications as described earlier.[Citation32] DPPH as a radical source was used to evaluate the antioxidant activity of ZnO NPs. The various concentrations (0.039, 0.078, 0.156, 0.312, 0.625, 1.25, 2.5, and 5 mg/mL) of ZnO NPs were suspended in deionized water and sonicated for 15 min. Then, 1.0 ml of 100 µM DPPH and 1.0 ml of sample were vortexed and stored for 30 min at room temperature in the dark. Then, the mixture was centrifuged at 6,000 rpm for 10 min and the absorbance of the supernatant was measured at 517 nm by using a UV-spectrophotometer (Cary 100, Varian, Australia). The measurements were done in triplicate and the data were averaged. The percentage of scavenging was calculated using EquationEq. (2)(2)
(2) :
(2)
(2)
where A0 and A1 are the absorptions of the methanol solution of DPPH radical and DPPH with ZnO NPs, respectively. The ascorbic acid was used as the standard antioxidant and the IC50 value was measured.[Citation33]
Antibacterial activity
The antibacterial activity of ZnO NPs was performed by the agar well diffusion method against E. coli (ATCC 25922) (Gram-negative) and S. aureus (ATCC 25923) (Gram-positive) bacteria as follows.[Citation34,Citation35] Briefly, bacteria were cultured until the visible turbidity of 0.5 of the McFarland standard was achieved. Pathogenic bacteria strains were spread using sterile swap on the freshly prepared Nutrient Agar media plate, and the wells were prepared using a sterile 1-mL micropipette tip. About 100 µL of ZnO NPs with different concentrations (0.039, 0.078, 0.156, 0.312, 0.625, 1.25, 2.5, and 5 mg/mL) were added into each well and the plates incubated at 37 °C for 24 h. Simultaneously. Chloramphenicol (30 µg) (Padtan Teb Co, Tehran, Iran) was used as a positive control. After the incubation period, the zone of inhibition was recorded by mm.
Results and discussion
Morphological study
The size and morphology of ZnO NPs were analyzed by FESEM. As it is shown in , the NPs are mostly spherical and also have a uniform distribution of particles. The SEM image shows the size of the ZnO NPs ranging from 33.5 to 34.4 nm.
XRD analysis
XRD was carried out to identify the crystalline structure and phase purity of the green synthesized ZnO. shows the diffraction peaks at 2θ = 31.720°, 34.400°, 36.212°, 47.494°, 62.603°, 56.519°, 66.203°, 67.066°, 68.992°, 72.516°, 76.860°, 81.315°, and 89.500° which correspond to (100), (002), (101), (102), (103), (110), (200), (112), (201), (004), (202), (104), and (203) reflection planes, respectively. All diffraction peaks confirm the formation of a crystalline hexagonal Wurtzite structure of the ZnO NPs (JCPDS no: 01-080-0075) and sharp diffraction peaks demonstrate the formation of a good crystalline structure.[Citation14] The X-ray diffraction pattern was similar to Janaki et al.[Citation36] and Pillai et al..[Citation37] The average size of ZnO NPs is assessed using the Debye–Scherrer formula (3):
(3)
(3)
where d is the crystal size, k is Scherrer’s constant (0.9), λ is X-Ray wavelength (0.15406 nm), ß is full width at half-maximum peak intensity (FWHM) and θ is the Bragg diffraction angle. So according to this equation, the crystallite size of ZnO was determined 9 nm.
FT-IR spectra
The formation of the green synthesized ZnO NPs is studied through FT-IR spectra which are shown in . The evident band at 434 cm−1 is attributed to Zn–O. This peak demonstrates the expected formation of NPs due to flavonoid (polyphenol) ingredients and is stabilized by protein structure. The absorption peaks at 1047 cm−1 can be also assigned to stretching vibrations of the C–OH functional group of phenolic compounds. The peak at 1650 cm−1 was assigned to the C = O bond of aromatic compounds.[Citation38] Bands observed at 2340 cm−1 are attributed to carbon dioxide molecules.[Citation39] The peak at 3443 cm−1 corresponds to the surface-adsorbed hydroxyl groups (O–H) of water.[Citation40] The obtained results were in agreement with Pillai et al.[Citation37] and Kaliraj et al..[Citation41]
Photocatalytic degradation of Tz dye
Out of numerous factors, which may affect the degradation of Tz dye, three specific parameters, that is, the effect of photocatalyst dose (g), the effect of Tz concentration (mg/L), and the effect of irradiation time (min) were chosen to conduct photocatalytic experiments. The impact of photocatalyst dose (g) on Tz degradation was assessed in the range of 35–120 min (). It was seen that increasing the amount of ZnO NPs additionally heightened the degradation efficiency from 65% (0.02 g) to 93% (0.05 g) which is consistent with the previous research reported in the literature.[Citation34, Citation42]
Figure 4. The effect of specific parameters (a) photocatalyst dose (g), (b) initial dye concentration (mg/mL), and (c) irradiation time on degradation efficiency of ZnO NPs.
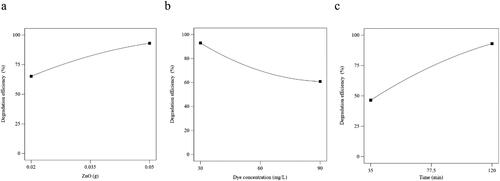
Similarly, the effect of Tz concentration (mg/L) was examined as another main factor for estimating the photocatalytic efficiency of ZnO NPs. As presented in , the degradation efficiency steadily declined from 93% to 65% with increasing an initial Tz concentration up to 90 mg/L. It has been reported that Tz degradation is a light-sensitive phenomenon.[Citation43] With a high concentration of dye, multilayer adsorption of dye molecules over active sites on the surface of the photocatalyst hinders the penetration ability of UV light to cause photo-oxidation.[Citation44] Therefore, the total of hydroxyl free radicals attacking Tz molecules would be lessened leading to the lowering of degradation efficiency. This result was well consistent with previous reports on the use of the dye with a high concentration.[Citation45,Citation46] Finally, the effect of UV contact time on degradation efficiency was measured up to 120 min (). The outcome data indicated a great upsurge in degradation (∼ 93%) up to 120 min.
Model fitting and statistical analysis
RSM was performed to realize the effect of three independent variables, that is, photocatalyst dose (g), initial dye concentration (mg/L), and irradiation time (min) on the degradation of Tz. One-to-one interactions between each process variable are clearly illustrated by response surface and contour plots, while the values were predicted and the values of the optimum response for degradation were measured as presented in . The final equation, which had effects on the degradation potential of ZnO NPs is described as follows (4):
(4)
(4)
Table 2. CCRD parameters were obtained for the independent variables and their corresponding response for degradation of Tz by ZnO NPs.
The accuracy of the experimental results was performed by the analysis of variance (ANOVA) as represented in . The ANOVA of the second-order quadratic polynomial model for dye degradation showed a high significance of the introduced model since Fisher values (F-value) of 21.11 were found with a p-value lower than 0.0001 at 95% confidence. The lack of fit of 1.50 has a good connection with the achieved R2 value (0.95) representing an acceptable compromise between expected and experimental responses of operational factors. Similarly, the responses of Adj-R2 (0.9095) and Pred-R2 (0.7187) were in the rational agreement where the variance was obtained to be under 0.2. Adequate precision measures (the signal-to-noise ratio), where a ratio greater than 4 indicates the overall model is desirable. In the present work, it was found to be 18.464, thus representing the model’s adequacy.
Table 3. Analysis of variance for the fit of the degradation efficiency from CCRD.
The ANOVA for the coefficients of the regression model for Tz degradation is illustrated in . Among three variables, contact time (C) has the most considerable effect on dye degradation (%) followed by initial Tz concentration (B), and photocatalyst dose (A) as exhibited by their F values of 3620.39, 1878.12, and 814.57, respectively. In this model A2, AB, AC, and BC did not show any great impact on Tz removal, except for B2 and C2. Initial Tz concentration has a low negative impact, whereas photocatalyst dose and contact time have an efficiently high positive impact on Tz degradation. Furthermore, the model fitting was proven by studying the residuals, that is, the difference between the observed and predicted values.
Response surface plots and optimal conditions
To recognize the impact of individual variables and their interaction on Tz degradation, 3D surfaces, and contour plots were designed by fixing one variable steady within the testable ranges (). As illustrated in , the interaction effect of photocatalyst dose and initial Tz concentration on dye degradation rate was plotted at a constant contact time of 120 min. The degradation efficiency (%) increased from 65.0% to 93% when the ZnO NPs dose increased from 0.02 to 0.05. The fact that the degradation efficiency is increased with increasing the amount of NPs is related to the active sites on the surfaces accessible for the photocatalysis reaction.[Citation27] Furthermore, the degradation efficiency decreases for a fixed ZnO NPs content at the high dye concentration because of the reduction in the number of active sites existing on the ZnO NPs surface.
Figure 5. 3D response surfaces plots and 2D contour plots of the interaction effects between (a) photocatalyst dose and initial Tz concentration (at constant t = 120 min), (b) photocatalyst dose and irradiation time (at constant initial Tz concentration =30 mg/mL and (c) irradiation time and initial Tz concentration (at constant ∼ 0.05 g ZnO).
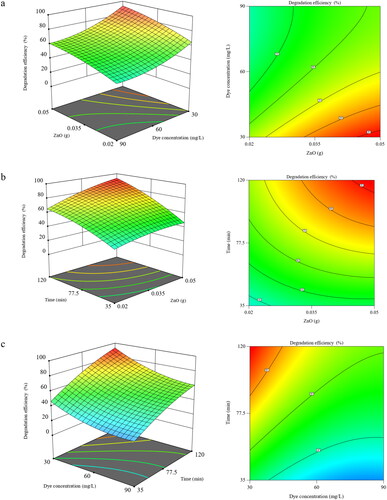
displays the interaction effects of photocatalyst dose and contact time on dye degradation. It is noted that the degradation efficiency increased progressively at higher contact time with increasing the amount of photocatalyst dose. Further increase in photocatalyst dose from 0.02 to 0.05 g led to a higher increase in photocatalytic activity up to 120 min.
shows the interaction impacts of initial Tz concentration and contact time on dye degradation. It is remarked that the degradation efficiency declined gradually at lower contact time with increasing initial Tz concentration. Higher exposure would result in the creation of a large amount of hydroxyl free radicals which are responsible for the oxidation of Tz molecules leading to more degradation.[Citation10]
Optimization procedure
The optimization was focused on considering three vital prerequisites, that is, treating the highest initial Tz concentration while minimizing contact time (faster) under a directly applicable photocatalyst dose. For the given experimental conditions, the optimum responses of photocatalyst dose, initial dye concentration, and irritation time are 0.048 g, 30.12 mg/L, and 119 min, respectively. The maximum degradation rate at this optimum condition is 92.5%. In the photocatalytic degradation mechanism of Tz with ZnO NPs under UV light irradiation, valence band (VB) electrons are excited and transferred into the conducting band (CB) and leaving behind electron holes (h+) in the VB. The molecular oxygen can be activated by electrons to produce superoxide radical’s anion Ο2●- and photogenerated holes (h+) not only react with water but also turn OH- to ΟH●. Both of them are energetic radicals, which can degrade Tz into inorganic by-products, CO2, and H2O.[Citation47] The overall mechanisms are illustrated below:
(5)
(5)
(6)
(6)
(7)
(7)
(8)
(8)
ZnO is prone to photo-corrosion which is one of the main reasons for its poor stability with organic pollutants in aqueous solutions. It is important in practical applications that NPs remain photostable during the photocatalytic reaction. To evaluate the stability of the biosynthesized ZnO NPs, Tz degradation was monitored for six consecutive cycles under optimum conditions (). NPs were separated after each cycle by centrifugation, then dried at 70 °C for 12 h for use in the following experiment. In the six consecutive runs, the degradation (%) of Tz gradually decreased from 93% to 59%. In this case, there were two prominent reasons for the decline: firstly, During the separation and photocorrosion processes, some photocatalysts were lost. Secondly, as a result of photocorrosion, some active sites are deactivated and the photocatalytic activity is gradually reduced. It was found that the biosynthesized ZnO NPs were highly reusable six times, suggesting they may be useful for practical wastewater treatment. The photocatalytic efficiency of ZnO NPs produced from SCG was herein comparable with that of ZnO synthesized by other plant extracts such as Thymus vulgaris leaf,[Citation42] Musa acuminata peel,[Citation10] soya chunk,[Citation48] Ulva lactuca seaweed,[Citation49] potato waste,[Citation27] jujube fruit,[Citation14] Justicia spicigera,[Citation50] and Hibiscus sabdariffa.[Citation51]
Antioxidant activity
Antioxidant molecules have a wide range of health and industrial applications. Antioxidants help the biological system function by scavenging free radicals. To assess the pharmacological role of nanoparticles, in vitro antioxidant activities are performed.[Citation33] DPPH is an electron transfer method and offers an easy and quick method of calculating antioxidant activity. After reduction, the DPPH color changes from deep violet to light yellow, which can be measured by its declined absorbance at 517 nm.[Citation41] The DPPH includes the π system and a nitrogen atom with an unpaired electron. The molar absorption of compounds on aromatic rings can be increased by an exchange, and this effect becomes more prominent if the exchange increases conjugation length. A benzene absorption band shifts from a shorter to a longer wavelength when the conjugation is extended. At 517 nm, the peak is responsible for n → π* the energy transition.[Citation52] The reaction mechanisms of the DPPH radical are described by EquationEq. (9)(9)
(9) :
(9)
(9)
The antioxidant activity of ZnO NPs on DPPH was illustrated () to upsurge as the concentration increased (0.039 mg/mL–40.82%; 0.078 mg/mL–40.94%; 0.156 mg/mL–43.09%; 0.312 mg/mL–43.18%; 0.625 mg/mL–48.96%; 1.25 mg/mL–55.65%; 2.5 mg/mL–66.98%; 5 mg/mL–78.07%). The ZnO NPs and ascorbic acid were confirmed to be effective in preventing the DPPH with the IC50 value of 0.9586, and 0.00012 mg/mL, respectively. The antioxidant activity of SCG has been demonstrated by scientific research.[Citation28] Furthermore, SCG contain higher levels of phenolic compounds.[Citation53] Hence polyphenols in SCG may play a significant role in the formation of ZnO NPs. Therefore, these phenolic compounds might act as reducing agents that increase the antioxidant properties of NPs.[Citation54] The free radical scavenging capacity of ZnO NPs may be a result of the change of electron density positioned at oxygen to the odd electron placed at the atom of the nitrogen in DPPH leading to the reduction in the intensity of n → π* transition at 517 nm.[Citation55] The scavenging capacity of standard ascorbic acid was significantly higher than ZnO NPs, but if further ZnO NPs used, the activity could surge. It is similarly observed from the literature that the synthesis of ZnO NPs using different plant extracts has antioxidant properties.[Citation54, Citation56–58]
Antibacterial activity
In this study, the antibacterial properties of ZnO NPs against gram-negative (E. coli) and gram-positive (S. aureus) bacteria were evaluated using the agar well diffusion method. In this method, the ZnO NPs indicated significant antibacterial activity on all two bacterial strains ( and ). It was demonstrated that increased concentration of ZnO NPs prevents microbial growth progressively. At a higher concentration (5 mg/mL), inhibition zone diameters values of 22 ± 0.25 and 28 ± 0.38 mm were observed for E. coli and S. aureus, respectively. However, at lower amounts of NPs (0.039, 0.078, and 0.156 mg/mL), both pathogenic bacteria exhibited resistance. Furthermore, the inhibition zone for S. aureus at a higher concentration of ZnO NPs (5 mg/mL) was almost 7% greater than that observed with the Chloramphenicol-impregnated disks. Based on the outcomes, it was determined that the ZnO NPs were more effective against Gram-positive bacteria in comparison with Gram-negative bacteria. This was a result of the different cell walls compositions of the bacteria, where Gram-negative bacteria have lipopolysaccharide molecules on the outer membrane layer which makes them resistant to ZnO NPs in comparison with Gram-positive bacteria. A comparable trend was found in the study by Alavi et al.[Citation35] and Hoseinzadeh et al.[Citation59] where the antibacterial activities of ZnO NPs were described to be more efficient on S. aureus than on E. coli. According to previous reports, the exact mechanism of antibacterial properties of ZnO NPs is currently not clear. However, the antibacterial activity of ZnO NPs can be explained by three mechanisms: (i) The production of reactive oxygen species (ROS) by electrostatic interaction between ZnO NPs and cell membrane that can react with DNA, membranes, proteins, and lipids, causing cell death,[Citation34, Citation60] (ii) The loss of cell membrane due to the release of zinc ions and their accumulation in the cell,[Citation61] and (iii) The direct contact between ZnO NPs and the cell wall of bacteria, destroying the bacterial cell.[Citation33] This action, however, is ultimately determined by the size of the NPs. Due to their smaller size and greater surface area, small NPs exhibit higher reactivity; thus, they are more effective at antibacterial activity compared to larger particles.[Citation62] Similarly, Abu Hanif et al. demonstrated that ZnO NPs have antibacterial properties dependent on their size.[Citation63] Therefore, in this research, we may assume that the antibacterial effect of NPs is related to their nanometric size and increased surface area, which allows for better adsorption onto the surface of bacterial cells. Other possible causes of cell damage include the production of ROS, the production of superoxide radicals and peroxide anions, and the internalization of nanoparticles.
Table 4. Zone of inhibition of antibacterial activities of ZnO NPs. The data represent the mean ± SD of three experiments.
Conclusions
ZnO NPs were successfully synthesized by using espresso SCG. The NPs were characterized through FESEM, XRD, and FT-IR which clearly illustrates their generation. FESEM image confirmed the morphology of NPs with an average size of 33.5–34.4 nm. The hexagonal wurtzite structure was found for NPs with a crystalline size of 9 nm. Tz dye was successfully degraded under UV light by adding NPs. RSM approach was effectively conducted to optimize photodegradation of Tz dye using ZnO NPs. Twenty sets of well-designed experiments, following CCRD, were performed to determine the individual and interaction effects of photocatalyst dose, initial dye concentration, and contact time on the photocatalytic degradation of Tz. The maximum percent of Tz dye removal was measured at 92.5% when the photocatalyst dose and the dye concentration were 0.048 g and 30.12 mg/L, respectively, at 119 min. The biosynthesized ZnO NPs exhibited significant antioxidant activities. When ZnO NPs were present in higher concentrations, their free radical scavenging activity increased. According to antibacterial results, the ZnO NPs were determined to be more effective against Gram-positive bacteria than Gram-negative bacteria. Hence, ZnO NPs using SCG have a great potential to eliminate pathogenic bacteria and dye pollutants from wastewater.
Disclosure statement
The author declares that he has no conflict of interest.
References
- Pavithra, K. G.; S. K, P.; J, V.; S. R, P. Removal of Colorants from Wastewater: A Review on Sources and Treatment Strategies. J. Ind. Eng. Chem. 2019, 75, 1–19. DOI: 10.1016/j.jiec.2019.02.011.
- Gupta, S.; Tejavath, K. K. Phytosynthesized Nanoparticle-Directed Catalytic Reduction of Synthetic Dyes: Beast to Beauty. Nanotechnol. Environ. Eng. 2021, 6, 6. DOI: 10.1007/s41204-021-00101-8.
- Mahmoodi, N. M.; Abdi, J. Nanoporous Metal-Organic Framework (MOF-199): Synthesis, Characterization and Photocatalytic Degradation of Basic Blue 41. Microchem. J. 2019, 144, 436–442. DOI: 10.1016/j.microc.2018.09.033.
- Srivastava, V.; Maydannik, P.; Sharma, Y. C.; Sillanpää, M. Synthesis and Application of Polypyrrole Coated Tenorite Nanoparticles (PPy@TN) for the Removal of the Anionic Food Dye ‘Tartrazine’ and Divalent Metallic Ions viz. Pb(ii), Cd(ii), Zn(ii), Co(ii), Mn(ii) from Synthetic Wastewater. RSC Adv. 2015, 5, 80829–80843. DOI: 10.1039/C5RA14108G.
- Gomathi, R.; Suhana, H. Green Synthesis, Characterization and Antimicrobial Activity of Zinc Oxide Nanoparticles Using Artemisia Pallens Plant Extract. Inorg. Nano-Metal Chem. 2020, 51, 1–10. DOI: 10.1080/24701556.2020.1852256.
- Aissani, T.; Yahiaoui, I.; Boudrahem, F.; Yahia Cherif, L.; Fourcad, F.; Amrane, A.; Aissani-Benissad, F. Sulfamethazine Degradation by Heterogeneous Photocatalysis with ZnO Immobilized on a Glass Plate Using the Heat Attachment Method and Its Impact on the Biodegradability. Reac. Kinet. Mech. Cat. 2020, 131, 471–487. DOI: 10.1007/s11144-020-01842-4.
- Oluwole, A. O.; Omotola, E. O.; Olatunji, O. S. Pharmaceuticals and Personal Care Products in Water and Wastewater: A Review of Treatment Processes and Use of Photocatalyst Immobilized on Functionalized Carbon in AOP Degradation. BMC Chem. 2020, 14, 62. DOI: 10.1186/s13065-020-00714-1.
- Mahmoodi, N. M.; Arami, M.; Zhang, J. Preparation and Photocatalytic Activity of Immobilized Composite Photocatalyst (Titania Nanoparticle/Activated Carbon). J. Alloys Compd. 2011, 509, 4754–4764. DOI: 10.1016/j.jallcom.2011.01.146.
- Truskewycz, A.; Shukla, R.; Ball, A. S. Iron Nanoparticles Synthesized Using Green Tea Extracts for the Fenton-like Degradation of Concentrated Dye Mixtures at Elevated Temperatures. J. Environ. Chem. Eng. 2016, 4, 4409–4417. DOI: 10.1016/j.jece.2016.10.008.
- Abdullah, F. H.; Abu Bakar, N. H. H.; Abu Bakar, M. Comparative Study of Chemically Synthesized and Low Temperature Bio-Inspired Musa acuminata Peel Extract Mediated Zinc Oxide Nanoparticles for Enhanced Visible-Photocatalytic Degradation of Organic Contaminants in Wastewater Treatment. J. Hazard Mater. 2021, 406, 124779. DOI: 10.1016/j.jhazmat.2020.124779.
- Mahmoodi, N. M. Photodegradation of Dyes Using Multiwalled Carbon Nanotube and Ferrous Ion. J. Environ. Eng. 2013, 139, 1368–1374. DOI: 10.1061/(ASCE)EE.1943-7870.0000762.
- Jawad, A. H.; Mohammed, I. A.; Abdulhameed, A. S. Tuning of Fly Ash Loading into Chitosan-Ethylene Glycol Diglycidyl Ether Composite for Enhanced Removal of Reactive Red 120 Dye: Optimization Using the Box–Behnken Design. J. Polym. Environ. 2020, 28, 2720–2733. DOI: 10.1007/s10924-020-01804-w.
- Park, J. K.; Rupa, E. J.; Arif, M. H.; Li, J. F.; Anandapadmanaban, G.; Kang, J. P.; Kim, M.; Ahn, J. C.; Akter, R.; Yang, D. C.; et al. Synthesis of Zinc Oxide Nanoparticles from Gynostemma Pentaphyllum Extracts and Assessment of Photocatalytic Properties through Malachite Green Dye Decolorization under UV Illumination-A Green Approach. Optik (Stuttg) 2021, 239, 166249. DOI: 10.1016/j.ijleo.2020.166249.
- Golmohammadi, M.; Honarmand, M.; Ghanbari, S. A Green Approach to Synthesis of ZnO Nanoparticles Using Jujube Fruit Extract and Their Application in Photocatalytic Degradation of Organic Dyes. Spectrochim. Acta A Mol. Biomol. Spectrosc. 2020, 229, 117961. DOI: 10.1016/j.saa.2019.117961.
- Akintelu, S. A.; Folorunso, A. S. A Review on Green Synthesis of Zinc Oxide Nanoparticles Using Plant Extracts and Its Biomedical Applications. BioNanoSci 2020, 10, 848–863. DOI: 10.1007/s12668-020-00774-6.
- Huang, Y.; Haw, C. Y.; Zheng, Z.; Kang, J.; Zheng, J.-C.; Wang, H.-Q. Biosynthesis of Zinc Oxide Nanomaterials from Plant Extracts and Future Green Prospects: A Topical Review. Adv. Sustain. Syst. 2021, 5, 2000266. DOI: 10.1002/adsu.202000266.
- Alavi, M.; Kennedy, J. F. Recent Advances of Fabricated and Modified Ag, Cu, CuO and ZnO Nanoparticles by Herbal Secondary Metabolites, Cellulose and Pectin Polymers for Antimicrobial Applications. Cellulose 2021, 28, 3297–3310. DOI: 10.1007/s10570-021-03746-5.
- Klingel, T.; Kremer, J. I.; Gottstein, V.; Rajcic de Rezende, T.; Schwarz, S.; Lachenmeier, D. W. A Review of Coffee by-Products Including Leaf, Flower, Cherry, Husk, Silver Skin, and Spent Grounds as Novel Foods within the European Union. Foods 2020, 9, 665. DOI: 10.3390/foods9050665.
- Stylianou, M.; Agapiou, A.; Omirou, M.; Vyrides, I.; Ioannides, I. M.; Maratheftis, G.; Fasoula, D. Converting Environmental Risks to Benefits by Using Spent Coffee Grounds (SCG) as a Valuable Resource. Environ. Sci. Pollut. Res. Int. 2018, 25, 35776–35790. DOI: 10.1007/s11356-018-2359-6.
- McNutt, J.; He, Q. (. Spent Coffee Grounds: A Review on Current Utilization. J. Ind. Eng. Chem. 2019, 71, 78–88. DOI: 10.1016/j.jiec.2018.11.054.
- Kovalcik, A.; Obruca, S.; Marova, I. Valorization of Spent Coffee Grounds: A Review. Food Bioprod. Process 2018, 110, 104–119. DOI: 10.1016/j.fbp.2018.05.002.
- Mohammadi, N.; Ehsani, M. R.; Bakhoda, H. Design and Evaluation of the Release Characteristics of Caffeine-Loaded Microcapsules in a Medicated Chewing Gum Formulation. Food Biophys. 2018, 13, 240–249. DOI: 10.1007/s11483-018-9530-y.
- Magalhães, L. M.; Machado, S.; Segundo, M. A.; Lopes, J. A.; Páscoa, R. N. M. J. Rapid Assessment of Bioactive Phenolics and Methylxanthines in Spent Coffee Grounds by FT-NIR Spectroscopy. Talanta 2016, 147, 460–467. DOI: 10.1016/j.talanta.2015.10.022.
- Leow, Y.; Yew, P. Y. M.; Chee, P. L.; Loh, X. J.; Kai, D. Recycling of Spent Coffee Grounds for Useful Extracts and Green Composites. RSC Adv. 2021, 11, 2682–2692. DOI: 10.1039/D0RA09379C.
- Shang, Y.-F.; Xu, J.-L.; Lee, W.-J.; Um, B.-H. Antioxidative Polyphenolics Obtained from Spent Coffee Grounds by Pressurized Liquid Extraction. South African J. Bot. 2017, 109, 75–80. DOI: 10.1016/j.sajb.2016.12.011.
- Ullah, H.; Mushtaq, L.; Ullah, Z.; Fazal, A.; Khan, A. M. Effect of Vegetable Waste Extract on Microstructure, Morphology, and Photocatalytic Efficiency of ZnO–CuO Nanocomposites. Inorg. Nano-Metal Chem. 2021, 51, 963–975. DOI: 10.1080/24701556.2020.1813766.
- Alharthi, F. A.; Al-Zaqri, N.; El Marghany, A.; Alghamdi, A. A.; Alorabi, A. Q.; Baghdadi, N.; Al-Shehri, H. S.; Wahab, R.; Ahmad, N. Synthesis of Nanocauliflower ZnO Photocatalyst by Potato Waste and Its Photocatalytic Efficiency against Dye. J. Mater. Sci. Mater. Electron. 2020, 31, 11538–11547. DOI: 10.1007/s10854-020-03701-3.
- Ranic, M.; Nikolic, M.; Pavlovic, M.; Buntic, A.; Siler-Marinkovic, S.; Dimitrijevic-Brankovic, S. Optimization of Microwave-Assisted Extraction of Natural Antioxidants from Spent Espresso Coffee Grounds by Response Surface Methodology. J. Clean. Prod. 2014, 80, 69–79. DOI: 10.1016/j.jclepro.2014.05.060.
- Mellinas, A. C.; Jiménez, A.; Garrigós, M. C. Optimization of Microwave-Assisted Extraction of Cocoa Bean Shell Waste and Evaluation of Its Antioxidant, Physicochemical and Functional Properties. LWT 2020, 127, 109361. DOI: 10.1016/j.lwt.2020.109361.
- Mellinas, A. C.; Jiménez, A.; Garrigós, M. C. Pectin-Based Films with Cocoa Bean Shell Waste Extract and ZnO/Zn-NPs with Enhanced Oxygen Barrier, Ultraviolet Screen and Photocatalytic Properties. Foods 2020, 9, 1572. DOI: 10.3390/foods9111572.
- Lari, K.; Ostovar, n The Effect of Magnetic Nanoparticles along with Magnetic Experimental Modeling for the Desalination of the Caspian Sea Water. J. Phys. Theor. Chem. 2018, 14, 297–306.
- Ravichandran, V.; Sumitha, S.; Ning, C. Y.; Xian, O. Y.; Kiew Yu, U.; Paliwal, N.; Shah, S. A. A.; Tripathy, M. Durian Waste Mediated Green Synthesis of Zinc Oxide Nanoparticles and Evaluation of Their Antibacterial, Antioxidant, Cytotoxicity and Photocatalytic Activity. Green Chem. Lett. Rev. 2020, 13, 102–116. DOI: 10.1080/17518253.2020.1738562.
- Zare, M.; Namratha, K.; Byrappa, K.; Surendra, D. M.; Yallappa, S.; Hungund, B. Surfactant Assisted Solvothermal Synthesis of ZnO Nanoparticles and Study of Their Antimicrobial and Antioxidant Properties. J. Mater. Sci. Technol. 2018, 34, 1035–1043. DOI: 10.1016/j.jmst.2017.09.014.
- Kahsay, M. H. Synthesis and Characterization of ZnO Nanoparticles Using Aqueous Extract of Becium Grandiflorum for Antimicrobial Activity and Adsorption of Methylene Blue. Appl. Water Sci. 2021, 11, 45. DOI: 10.1007/s13201-021-01373-w.
- Alavi, M.; Dehestaniathar, S.; Mohammadi, S.; Maleki, A.; Karimi, N. Antibacterial Activities of Phytofabricated ZnO and CuO NPs by Mentha pulegium Leaf/Flower Mixture Extract against Antibiotic Resistant Bacteria. Adv. Pharm. Bull. 2021, 11, 497–504. DOI: 10.34172/apb.2021.057.
- Janaki, A. C.; Sailatha, E.; Gunasekaran, S. Synthesis, Characteristics and Antimicrobial Activity of ZnO Nanoparticles. Spectrochim. Acta A Mol. Biomol. Spectrosc. 2015, 144, 17–22. DOI: 10.1016/j.saa.2015.02.041.
- Pillai, A. M.; Sivasankarapillai, V. S.; Rahdar, A.; Joseph, J.; Sadeghfar, F.; Anuf A, R.; Rajesh, K.; Kyzas, G. Z. Green Synthesis and Characterization of Zinc Oxide Nanoparticles with Antibacterial and Antifungal Activity. J. Mol. Struct. 2020, 1211, 128107. DOI: 10.1016/j.molstruc.2020.128107.
- Khan, M.; Ware, P.; Shimpi, N. Synthesis of ZnO Nanoparticles Using Peels of Passiflora foetida and Study of Its Activity as an Efficient Catalyst for the Degradation of Hazardous Organic Dye. SN Appl. Sci. 2021, 3, 528. DOI: 10.1007/s42452-021-04436-4.
- Babaheydari, A. K.; Salavati-Niasari, M.; Khansari, A. Solvent-Less Synthesis of Zinc Oxide Nanostructures from Zn(Salen) as Precursor and Their Optical Properties. Particuology 2012, 10, 759–764. DOI: 10.1016/j.partic.2012.03.006.
- Mohammadi, N.; Ehsani, M. R.; Bakhoda, H. Development of Caffeine-Encapsulated Alginate-Based Matrix Combined with Different Natural Biopolymers, and Evaluation of Release in Simulated Mouth Conditions. Flavour Fragr. J. 2018, 33, 357–366. DOI: 10.1002/ffj.3452.
- Kaliraj, L.; Ahn, J. C.; Rupa, E. J.; Abid, S.; Lu, J.; Yang, D. C. Synthesis of Panos Extract Mediated ZnO Nano-Flowers as Photocatalyst for Industrial Dye Degradation by UV Illumination. J. Photochem. Photobiol. B 2019, 199, 111588. DOI: 10.1016/j.jphotobiol.2019.111588.
- Zare, M.; Namratha, K.; Thakur, M. S.; Byrappa, K. Biocompatibility Assessment and Photocatalytic Activity of Bio-Hydrothermal Synthesis of ZnO Nanoparticles by Thymus Vulgaris Leaf Extract. Mater. Res. Bull. 2019, 109, 49–59. DOI: 10.1016/j.materresbull.2018.09.025.
- Chen, X.; Wu, Z.; Liu, D.; Gao, Z. Preparation of ZnO Photocatalyst for the Efficient and Rapid Photocatalytic Degradation of Azo Dyes. Nanoscale Res. Lett. 2017, 12, 143. DOI: 10.1186/s11671-017-1904-4.
- Asfaram, A.; Ghaedi, M.; Dashtian, K.; Ghezelbash, G. R. Preparation and Characterization of Mn0.4Zn0.6Fe2O4 Nanoparticles Supported on Dead Cells of Yarrowia Lipolytica as a Novel and Efficient Adsorbent/Biosorbent Composite for the Removal of Azo Food Dyes: Central Composite Design Optimization Study. ACS Sustain. Chem. Eng. 2018, 6, 4549–4563. DOI: 10.1021/acssuschemeng.7b03205.
- Tayeb, A. M.; Tony, M. A.; Ismaeel, E. K. Engineered Nanostructured ZnO for Water Remediation: Operational Parameters Effect, Box–Behnken Design Optimization and Kinetic Determinations. Appl. Water Sci. 2019, 9, 43. DOI: 10.1007/s13201-019-0921-0.
- Begum, S.; Ahmaruzzaman, M. Green Synthesis of SnO 2 Quantum Dots Using Parkia speciosa Hassk Pods Extract for the Evaluation of anti-Oxidant and Photocatalytic Properties. J. Photochem. Photobiol. B 2018, 184, 44–53. DOI: 10.1016/j.jphotobiol.2018.04.041.
- Bouarroudj, T.; Aoudjit, L.; Djahida, L.; Zaidi, B.; Ouraghi, M.; Zioui, D.; Mahidine, S.; Shekhar, C.; Bachari, K. Photodegradation of Tartrazine Dye Favored by Natural Sunlight on Pure and (Ce, Ag) Co-Doped ZnO Catalysts. Water Sci. Technol. 2021, 83, 2118–2134. DOI: 10.2166/wst.2021.106.
- Safardoust-Hojaghan, H.; Salavati-Niasari, M.; Amiri, O.; Rashki, S.; Ashrafi, M. Green Synthesis, Characterization and Antimicrobial Activity of Carbon Quantum Dots-Decorated ZnO Nanoparticles. Ceram. Int. 2021, 47, 5187–5197. DOI: 10.1016/j.ceramint.2020.10.097.
- Ishwarya, R.; Vaseeharan, B.; Kalyani, S.; Banumathi, B.; Govindarajan, M.; Alharbi, N. S.; Kadaikunnan, S.; Al-Anbr, M. N.; Khaled, J. M.; Benelli, G. Facile Green Synthesis of Zinc Oxide Nanoparticles Using Ulva lactuca Seaweed Extract and Evaluation of Their Photocatalytic, Antibiofilm and Insecticidal Activity. J. Photochem. Photobiol. B 2018, 178, 249–258. DOI: 10.1016/j.jphotobiol.2017.11.006.
- Soto-Robles, C. A.; Nava, O.; Cornejo, L.; Lugo-Medina, E.; Vilchis-Nestor, A. R.; Castro-Beltrán, A.; Luque, P. A. Biosynthesis, Characterization and Photocatalytic Activity of ZnO Nanoparticles Using Extracts of Justicia spicigera for the Degradation of Methylene Blue. J. Mol. Struct. 2021, 1225, 129101. DOI: 10.1016/j.molstruc.2020.129101.
- Soto-Robles, C. A.; Luque, P. A.; Gómez-Gutiérrez, C. M.; Nava, O.; Vilchis-Nestor, A. R.; Lugo-Medina, E.; Ranjithkumar, R.; Castro-Beltrán, A. Study on the Effect of the Concentration of Hibiscus sabdariffa Extract on the Green Synthesis of ZnO Nanoparticles. Results Phys. 2019, 15, 102807. DOI: 10.1016/j.rinp.2019.102807.
- Apak, R.; Özyürek, M.; Güçlü, K.; Çapanoğlu, E. Antioxidant Activity/Capacity Measurement. 1. Classification, Physicochemical Principles, Mechanisms, and Electron Transfer (ET)-Based Assays. J. Agric. Food Chem. 2016, 64, 997–1027. DOI: 10.1021/acs.jafc.5b04739.
- Balzano, M.; Loizzo, M. R.; Tundis, R.; Lucci, P.; Nunez, O.; Fiorini, D.; Giardinieri, A.; Frega, N. G.; Pacetti, D. Spent Espresso Coffee Grounds as a Source of anti-Proliferative and Antioxidant Compounds. Innov. Food Sci. Emerg. Technol. 2020, 59, 102254. DOI: 10.1016/j.ifset.2019.102254.
- Suresh, D.; Nethravathi, P. C.; Rajanaika, H.; Nagabhushana, H.; Sharma, S. C.; Udayabhanu. Green Synthesis of Multifunctional Zinc Oxide (ZnO) Nanoparticles Using Cassia Fistula Plant Extract and Their Photodegradative, Antioxidant and Antibacterial Activities. Mater. Sci. Semicond. Process 2015, 31, 446–454. DOI: 10.1016/j.mssp.2014.12.023.
- Das, D.; Nath, B. C.; Phukon, P.; Kalita, A.; Dolui, S. K. Synthesis of ZnO Nanoparticles and Evaluation of Antioxidant and Cytotoxic Activity. Colloids . B Biointerfaces 2013, 111, 556–560. DOI: 10.1016/j.colsurfb.2013.06.041.
- Brindhadevi, K.; Samuel, M. S.; Verma, T. N.; Vasantharaj, S.; Sathiyavimal, S.; Saravanan, M.; Pugazhendhi, A.; Duc, P. A. Zinc Oxide Nanoparticles (ZnONPs) -Induced Antioxidants and Photocatalytic Degradation Activity from Hybrid Grape Pulp Extract (HGPE). Biocatal. Agric. Biotechnol. 2020, 28, 101730. DOI: 10.1016/j.bcab.2020.101730.
- Nagajyothi, P. C.; Cha, S. J.; Yang, I. J.; Sreekanth, T. V. M.; Kim, K. J.; Shin, H. M. Antioxidant and anti-Inflammatory Activities of Zinc Oxide Nanoparticles Synthesized Using Polygala tenuifolia Root Extract. J. Photochem. Photobiol. B 2015, 146, 10–17. DOI: 10.1016/j.jphotobiol.2015.02.008.
- Rajeshkumar, S.; Kumar, S. V.; Ramaiah, A.; Agarwal, H.; Lakshmi, T.; Roopan, S. M. Biosynthesis of Zinc Oxide Nanoparticles Using Mangifera indica Leaves and Evaluation of Their Antioxidant and Cytotoxic Properties in Lung Cancer (A549) Cells. Enzyme Microb. Technol. 2018, 117, 91–95. DOI: 10.1016/j.enzmictec.2018.06.009.
- Hoseinzadeh, E.; Alikhani, M.-Y.; Samarghandi, M.-R.; Shirzad-Siboni, M. Antimicrobial Potential of Synthesized Zinc Oxide Nanoparticles against Gram Positive and Gram Negative Bacteria. Desalin. Water Treat. 2014, 52, 4969–4976. DOI: 10.1080/19443994.2013.810356.
- Alavi, M.; Rai, M. Antisense RNA, the Modified CRISPR-Cas9, and Metal/Metal Oxide Nanoparticles to Inactivate Pathogenic Bacteria. Cell. Mol. Biomed. Rep. 2021, 1, 52–59. DOI: 10.55705/cmbr.2021.142436.1014.
- Zare, E.; Pourseyedi, S.; Khatami, M.; Darezereshki, E. Simple Biosynthesis of Zinc Oxide Nanoparticles Using Nature’s Source, and It’s in Vitro Bio-Activity. J. Mol. Struct. 2017, 1146, 96–103. DOI: 10.1016/j.molstruc.2017.05.118.
- Mohd Yusof, H.; Abdul Rahman, N.; Mohamad, R.; Zaidan, U. H.; Samsudin, A. A. Biosynthesis of Zinc Oxide Nanoparticles by Cell-Biomass and Supernatant of Lactobacillus plantarum TA4 and Its Antibacterial and Biocompatibility Properties. Sci. Rep. 2020, 10, 19996. DOI: 10.1038/s41598-020-76402-w.
- Hanif, M.; Lee, I.; Akter, J.; Islam, M.; Zahid, A.; Sapkota, K.; Hahn, J. Enhanced Photocatalytic and Antibacterial Performance of ZnO Nanoparticles Prepared by an Efficient Thermolysis Method. Catalysts 2019, 9, 608. DOI: 10.3390/catal9070608.