Abstract
Introduction: Liposomes are nanospheres of lipid bilayer which can bind drug either in the phospholipid membrane or in the central aqueous droplet. Liposomes loaded with a drug can facilitate drug delivery, and empty liposomes can sequester certain drugs to reduce aqueous drug concentrations in experimental intoxication. Therapeutic dialysis in intoxication is limited by drug volumes of distribution and blood protein binding. In rats intoxicated with intravenous amitriptyline, we examined adding intravenous membrane-binding 1,2-dioleoyl-sn-glycero-3-phosphoglycerol (DOPG) liposomes to liposome supported peritoneal dialysis (LSPD) in which liposomes in dialysate were augmented with an acidic core. The hypothesis was that a “gradient of liposome affinity” would increase LSPD amitriptyline concentrations.
Methods: Seven control and five intravenous DOPG liposome pre-treated rats were injected with amitriptyline, followed 10 min later by a 10-min LSPD dwell.
Results: DOPG liposomes increased blood amitriptyline concentrations by 50%; control (median [IQR] 1250 [951–1356] nmol/L; intravenous DOPG 1702 [1602–1864] nmol/L, p = 0.032). However, there was no corresponding increase in LSPD dialysate amitriptyline concentrations at the end of the dwell; control (median [IQR] 430 [334–2180] nmol/l, intravenous DOPG 414 [387–483] nmol/L p = 0.75).
Conclusions: Pre-treatment with DOPG liposomes decreased amitriptyline volume of distribution but had no significant effect on elimination of amitriptyline by LSPD.
Introduction
Liposomes are nanosphere sacs of phospholipid bilayer enclosing an aqueous droplet, currently in clinical use as controlled release vectors for targeted drug delivery. Liposomes can bind drug either through electrostatic interaction in the membrane (where both the phospholipid amphiphile and the lipid tail play a role) and via entrapment of drug in the aqueous core. Entrapment in the aqueous core is driven by ion trapping – pH of the liposomal centre is altered such that weak acids or bases pass through the liposome membrane, become charged in the aqueous centre, and are entrapped. These pH gradient liposomes generally have greater binding avidity for drug than those which bind drug in the membrane alone [Citation1]. A growing body of experimental work demonstrates antidotal effect when the site of liposome drug loading is changed to in vivo [Citation1,Citation2]. Use of “empty” liposomes injected intravenously has ameliorated drug toxicity in animal models of dabigatran, calcium channel blocker and tricyclic antidepressant (TCA) toxicity [Citation3–6]. Factors such as complement activation limit the dose (and thus binding capacity) of “empty” intravenous liposomes and thereby limit the effectiveness of this approach [Citation2].
Limitations of both hemodialysis and peritoneal dialysis include large volumes of distribution (small fraction of drug in the blood), and significant blood protein binding of drugs commonly taken in overdose [Citation7]. Liposome supported peritoneal dialysis (LSPD) may overcome these limitations and, ameliorate intoxication in animal models [Citation8,Citation9]. In LSPD dialysate, capacity and avidity for drug is increased by the addition of acidic centre liposomes. Compared to peritoneal dialysis, LSPD increases amitriptyline concentrations in dialysate and appears to offset protein binding in blood [Citation8,Citation9]. One-third of amitriptyline in peritoneal bloodflow was estimated to be extracted into LSPD in a rat model [Citation9], compared with reported low single digit blood free percentages which appeared to equilibrate with unaugmented dialysate in previous models [Citation9,Citation10]. LSPD may overcome protein binding of drug in blood flowing through the peritoneal membrane. We proposed that intravenous nanoparticles with reversible drug-binding properties could increase blood drug carriage and could further increase drug concentrations in LSPD dialysate.
1,2-Dioleoyl-sn-glycero-3-phosphoglycerol (DOPG) liposomes (negatively charged liposomes) composed of DOPG/N-(carbonyl-methoxypolyethyleneglycol 2000)-1,2-dipalmitoyl-SN-glycero-3-phosphoethanolamine (DPPE mPEG2000) with a pharmacologically inert core may bind drug via electrostatic interaction within the membrane. Such DOPG liposomes have bound TCA in vitro in suspensions of blood cells and plasma proteins [Citation11], exhibited reversible TCA binding characteristics [Citation12], and have effected hemodynamic recovery from TCA toxicity in experimental rabbit models [Citation5].
DOPG liposomes in blood reversibly bind amitriptyline, and it is possible that amitriptyline bound in these liposomes could move into a higher affinity dialysate (). The hypothesis tested in this study is that increased blood amitriptyline concentrations caused by binding to intravascular DOPG liposomes will lead to increased amitriptyline concentrations in LSPD. If successful, this technique would offer a potential mitigant to current limitations of dialysis in poisoning.
Figure 1. (a) Amitriptyline in blood exists as protein bound and unbound. Unbound amitriptyline equlibrates between blood and peritoneal dialysate. (b) Addition of liposomes with acidic core in peritoneal dialysate increases the gradient from blood to dialysate. Protein-bound amitriptyline is in equilibrium with unbound amitriptyline and releases amitriptyline in the blood. (c) Amitriptyline-binding DOPG liposomes in the blood increase the blood amitriptyline concentration. Study question: Does addition of amitriptyline-binding DOPG liposomes in the blood promote extraction of amitriptyline into dialysate?
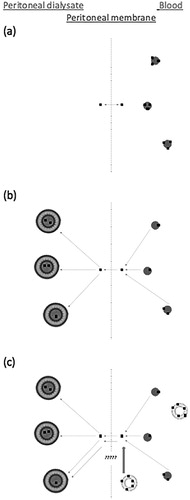
Materials and methods
Liposome/dialysate preparation
Liposomes were batch prepared in the School of Pharmacy laboratory, University of Auckland one week prior to use. Pure lipids (54% dipalmitoylphosphatidylcholine [DPPC], 45% cholesterol and 1% 1,2-distearoyl-sn-glycero-3-phosphoethanolamine-N-[methoxy(polyethylene glycol)-2000] (DSPEmPEG 2000); and 95% DOPG 5% DPPEmPEG, respectively as molar ratios) were dissolved in a 75:25 volume ratio of chloroform and methanol at a final total lipid concentration of 30 mg/ml. Total lipid weight was 1000 mg for DPPC and 400 mg for DOPG based liposomes. Lipids were dried in the vial under a stream of nitrogen in a water bath at 40 °C until formation of a dry thin layer of lipid on the vial walls. Citric acid solution was made by addition of citric acid to deionized water until the target pH of 2 was reached. The DPPC/cholesterol/DSPE2000 lipid film was rehydrated with a volume of citric acid solution (pH2) equal to that of the initial organic solvent to form liposomes. Bath sonication at 35 kHz of the coarse liposomes was undertaken for 60 min to reduce the size of liposomes and suspend any particulate matter. The liposome suspension was then extruded once through a 200 nm filter (10 ml LIPEX™ Extruder (Northern Lipids Inc., Burnaby, Canada) at 400–500 psi at room temperature. The DOPG/DSPEmPEG2000 lipid film was hydrated using phosphate buffered saline pH 7.4 to concentration of 20 mg lipid per ml. This was sonicated until all lipids were suspended, and then passed three times through a 100 nm filter. Particle size was measured using a Malvern Nano ZS Zetasizer (Malvern Instruments, UK).
Immediately prior to use, DPPC liposomes were pelleted by centrifugation at 20,000g for 30 min, and then resuspended in peritoneal dialysate solution with a final concentration of total lipids of 3 mg/ml. Peritoneal dialysate was prepared using commercially available compound sodium lactate (20 ml) with 60 mg lipid load of liposomes in suspension. The pH of dialysate was adjusted to a pH of 7.5 by dropwise addition of NaOH solution pH 12.8.
Location
The animal study was conducted at the Ruakura Animal Research facility, Hamilton, New Zealand. The Ruakura Animal Ethics committee reviewed and approved all study protocols.
Animals
Fourteen female Sprague–Dawley rats were studied, all born from January to March 2017 with an age range of 119–222 days and weight range 310–435 g. Animals were kept in single gender enclosures with no chance of pregnancy. Twelve-hour light-dark cycles (lights on/off at 07:00/19:00 h) and climate control were maintained. Access to feed and water was allowed ad libitum until the day of animal utilization.
Animal manipulations
On the day of study animals were sedated with ketamine at 50 mg/kg (Mayne Pharma Ltd., Auckland, New Zealand), and xylazine at 4 mg/kg (Bayer HealthCare, Leverkusen, Germany) via intraperitoneal injection. Animals were then placed on a warming board at 38.5 °C. An intravenous (IV) cannula was placed in the tail vein using a needle over catheter technique (24 G × 0.75 in Insyte, BD, Switzerland). Following dissection of the anterior neck, a tracheostomy tube (14 G × 1.77 in Insyte, BD, Switzerland) was placed under direct vision. Mechanical ventilation using a small animal ventilator (Inspira ASV, Harvard Apparatus, USA) was then undertaken with oxygen as the inspired gas admixed with 2% isoflurane (Merial, Auckland, New Zealand) via a vaporizer (Somnosuite Small Animal Anesthesia System, Kent Scientific Corporation, Torrington, USA) to maintain anesthesia. Electrocardiograph (ECG) electrodes (MLA1213 Needle Electrodes, AD Instruments, Bella Vista, Australia) were placed in three limbs of the subject for continuous ECG monitoring (Animal Bio Amp, AD Instruments, Bella Vista, Australia).
A carotid arterial line was placed under direct vision using a catheter over needle technique and tied off (24 G × 0.75 in Insyte, BD, Switzerland) allowing for arterial blood sampling and monitoring of arterial pressure (BP Amp, AD Instruments, Bella Vista, Australia). Anaesthesia, mechanical ventilation, and blood pressure and ECG monitoring were continued for the duration of the experiment. Hemodynamic metrics were recorded on a standardized template at predetermined time points in the experiment.
A 1 cm incision was made in the ventral midline 2 cm below the xyphisternum for placement of a peritoneal dialysis catheter. The abdominal muscles were grasped and elevated with subsequent dissection into the peritoneal space. A 10 cm fenestrated catheter was directed into the right lower quadrant and the incision in the abdominal wall and peritoneal space sealed by clamping.
Experimental protocol
Following completion of animal manipulations and collection of baseline metrics, subjects were randomly assigned to receive either IV DOPG liposomes 100 mg/kg lipid load or an equal volume of compound sodium lactate prior to administration of amitriptyline. Amitriptyline (Sigma-Aldrich, St. Louis, USA) dissolved in 0.9% NaCl (2.5 mg/ml) was injected into the tail vein over 2.5 min at a dose of 5 mg/kg. The end of amitriptyline injection was nominated time zero (T0).
At 10 min following drug administration (T10) blood was drawn for measurement of amitriptyline concentrations. Simultaneously 20 ml of dialysate/liposome suspension was injected into the peritoneal cavity over 30 s and manually agitated.
At 15 and 20 min (T15 and T20) a further blood sample was drawn for amitriptyline concentration measurement. Peritoneal dialysate was drawn at T20 for measurement of dialysate amitriptyline concentration.
At the end of the experimental period mechanical ventilation was ceased and a terminal bleed was taken from the carotid arterial line. Death was confirmed by absent respiratory efforts and examination of the ECG trace.
As in previous work by our group [Citation9] a post hoc estimation of the proportion of amitriptyline from peritoneal blood flow extracted into peritoneal dialysate was made for each group, termed estimated extraction ratio (ERest). Total amitriptyline in dialysate was calculated as (20 ml)×(concentration in dialysate at the end of dwell [nmol/mL]), dialysate volume being considered constant throughout the dwell period for the purposes of calculation. To estimate drug delivery to the peritoneal membrane, assumed values for cardiac output in anaesthetized rats and percentage of the cardiac output to peritoneum were sourced from the literature at 200 ml/min and 1.5% of cardiac output [Citation13,Citation14]. Over the dwell time of peritoneal dialysate peritoneal blood flow was thus estimated at 30 ml. ERest was calculated as estimated amount in peritoneum at end of dwell/estimated amitriptyline delivery = (20 × peritoneal amitriptyline concentration)/(30 × average blood concentration for peritoneal dwell).
Amitriptyline assay
Amitriptyline concentrations were measured using a liquid chromatography-mass spectrometry method (Sciex 3200 MSD Aglient 1200 series LS, Sciex [Redwood City, USA]) with isotope matched internal standards. The whole blood sample was prepared by a protein crash with acetonitrile, followed by centrifugation after which an aliquot was presented to the instrument for analysis. A four-point matrix-matched standard curve was used to calibrate the analyser for each run with two levels of quality control.
Statistical analysis
The experiment was powered on the expectation of a 100% increase in average blood amitriptyline concentration over the peritoneal dwell with IV liposomes. This increase was based on that seen with intravenous lipid emulsion after oral dosing of amitriptyline [Citation15]. The standard deviation of amitriptyline concentration in LSPD dialysate in previous work was 30% of the measured concentration [Citation8]. The hypothesis tested in this study is that increased blood amitriptyline concentrations caused by binding to intravascular DOPG liposomes will lead to increased amitriptyline concentrations in LSPD. We powered for a doubling of expected dialysate concentrations. The experiment was powered to 80% at an alpha of 0.05 to detect a doubling of peritoneal dialysate concentration with eight subjects in the control group and six in the IV liposome group [Citation16]. We used nonparametric statistical tests (Kruskal–Wallis and Mann–Whitney) as appropriate. We made no correction for multiple comparisons.
Results
Liposome characteristics
DPPC liposomes
Mean DPPC liposome size was 430 nm with a polydispersity index (PDI) (a measure of spread of liposome size) of 0.48 and a mean zeta potential (the surface charge of liposomes in an aqueous environment) of −14.5 mV. Mean DOPG liposome size was 119 nm with a PDI of 0.1 and a mean zeta potential of −33.0 mV.
Baseline and physiologic data
One animal inadvertantly received one-fifth the dose of intraperitoneal liposomes in the control group, and one animal died during experimental manipulation in the IV liposome group. These were excluded from analysis.
The dose of amitriptyline was sufficient to cause hypotension in both groups. A Kruskal–Wallis comparing within group mean arterial pressure (MAP) pre amitriptyline injection, immediately post amitriptyline injection, and at T10 was significant for both control and IV liposome groups (p = 0.032 and 0.007 for control and IV liposome groups, respectively). There were no statistically significant between group differences in baseline metrics nor MAP at any timepoint (Mann–Whitney) ().
Table 1. Baseline and physiologic data (shown as median [IQR]).
Effects of DOPG liposomes on blood and dialysate amitriptyline concentrations
Intravenous pre-treatment with DOPG liposomes sequestered amitriptyline in the blood. Total blood amitriptyline concentrations were higher at all timepoints measured (), though differences were not statistically significant (p = 0.07 at T10 and p = 0.051 at T15 and T20, Mann–Whitney). Average blood concentration of amitriptyline over the period of dialysate dwell (T10–T20) in the DOPG group was greater than for controls (median [IQR] 1250 [951–1356] nmol/L for control group; 1702 [1602–1864] nmol/L for DOPG group; p = 0.032, Mann–Whitney; ).
Figure 3. Average blood amitriptyline concentration over the period of the peritoneal dwell, and dialysate concentration of amitriptyline at the end of the peritoneal dwell.
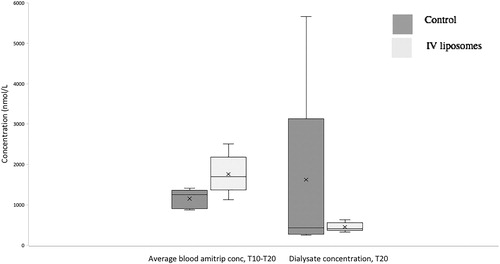
While IV DOPG liposomes prior to amitriptyline injection increased blood concentrations there was no corresponding increase in extraction of amitriptyline into dialysate. Dialysate concentrations being control (median [IQR]) 430 [334–2180] nmol/l, intravenous DOPG 414 [387–483] nmol/L; p = 0.75, Mann–Whitney, ).
The ERest was greater in controls than in DOPG-treated animals, but this difference did not reach statistical significance. Median ERest (IQR) was 0.30 (0.2–1.05) for control animals, and was 0.14 (0.14–0.24) for IV DOPG-treated animals (p = 0.07, Mann–Whitney, ).
Discussion
Liposomal augmentation of peritoneal dialysate has been shown to increase dialysate drug concentration in previous models [Citation8,Citation9]. We investigated whether the addition of less avidly binding liposomes to blood would further increase dialysate drug concentrations. Our results showed an increase in blood amitriptyline concentrations and decreased the volume of distribution for amitriptyline, but no difference in end dialysate concentrations for the DOPG pre-treated group. Taken together, these results suggest that pre-treatment with DOPG liposomes increases blood amitriptyline carriage, but amitriptyline in intravascular liposomes did not move into dialysate meaning there was no net effect on dialysate amitriptyline concentration.
Experimental conditions herein differ from those of clinical intoxication in which the route of intoxication is predominantly oral and intravenous drug binders would be given after, rather than before exposure. While these results are not directly applicable to other dosing scenarios, they do suggest that extra amitriptyline in blood carried in DOPG liposomes was minimally available for exchange into peritoneal dialysate. Pharmacologic parameters which merit consideration for hemodialysis in intoxication (a volume of distribution less than 2 l/kg and extractable fraction of >20%) seem unlikely to be achievable with this combination of blood/dialysate nanoparticles for amitriptyline [Citation11,Citation17].
Future work may uncover a blood/dialysate nanoparticle combination holding more promise for eventual clinical utility. Lower affinity blood lipid based particles such as lipid emulsion (e.g. Intralipid®), higher pH acidic centre liposomes, or intravenous albumin multimers may improve drug extraction into LSPD.
Given a pKa of 9.7, greater than 99% of amitriptyline is ionized at physiologic pH [Citation18], with significant potential for binding to negative amphiphile liposomes. DOPG liposomes bind amitriptyline in the presence plasma of proteins and red blood cells in vitro and augment recovery from TCA toxicity in vivo [Citation5,Citation11]. Average concentration results are consistent with TCA sequestration by DOPG liposomes. While we observed no physiologic effects, our study was not powered to these endpoints. Further investigation of intravenous negative amphiphile liposomes as drug binding antidotes is warranted with some caveats. Intravascular drug binders present during the absorptive phase of an enteric intoxication may diminish first pass metabolism, paradoxically increasing toxicity [Citation15,Citation19]. Additionally, the binding mechanism of negative amphiphile liposomes is only moderately selective. Endogenous molecules with both polar and hydrophilic domains (e.g. serotonin and other monoamines) could theoretically be bound.
This experiment has other limitations. The small sample size comes with the risk of type II error. There was, however, no signal for effect for intravenous DOPG liposomes to augment LSPD. We regarded average blood amitriptyline concentrations as the a priori measure of interest for effect of DOPG liposomes. A caveat in interpreting this result is differences did not reach statistical significance at any discreet timepoint and we did not correct for multiple comparisons. We used low doses of small intraperitoneal liposomes relative to other experiments [Citation8], which may have biased against effect. However, median ERest was the same as previous work for LSPD [Citation9] and was similarly greater than ERest for unaugmented dialysate from that study. This suggests that dialysate augmentation was effective in the present work. Peritoneal dialysis has limited clinical use in treating drug intoxication partly due to lower bloodflow relative to hemodialysis.
Future work exploring the use of nanoparticle drug carriers in ameliorating intoxication could follow a number of avenues. The use of intraperitoneal enzyme containing liposomes to effect detoxification through metabolism rather than sequestration is a promising experimental strategy [Citation20]. Albumin augmented hemodialysis may increase extraction of protein-bound drugs [Citation21]. Similar hemodialysis experiments could use the combination of high affinity dialysates/high flux membranes (HAHF).
Conclusions
Pre-treatment with intravenous DOPG liposomes increased the time-averaged blood concentrations of amitriptyline but did not increase LSPD dialysate concentrations.
Data availability
Granular data from this experiment are available on reasonable request
Acknowledgements
The authors would like to acknowledge Mr. Ric Broadhurst and Mr. Robert Smith of Agresearch Ruakura for assistance with the experiment. We also wish to acknowledge Miss Emma Kang for assistance with liposome manufacture.
Disclosure statement
The authors declare no conflicts of interest.
Funding
This study was self-funded by the authors.
References
- Cave G, Wu Z, Harvey M. Drug scavenging lipid-based nanoparticles as detoxifying agents. In: Salam A, Makhlouf M, Barhoum A, editors. Emerging applications of nanoparticles and architectural nanostructures. Vol. 1. Amsterdam, Netherlands: Elsevier; 2018.
- Damitz R, Chauhan A. Parenteral emulsions and liposomes to treat drug overdose. Adv Drug Deliv Rev. 2015;90:12–23.
- Cave G, Wu Z, Hunter N. Reversal of lipophilic weak bases using pH gradient acidic centre liposomes: demonstration of effect in dabigatran-induced anticoagulation. Clin Toxicol. 2016;54:428–433.
- Bertrand N, Bouvet C, Moreau P, et al. Transmembrane pH gradient liposomes to treat cardiovascular drug intoxication. ACS Nano. 2010;12:7552–7558.
- Cave G, Harvey M, Shaw T. Comparison of intravenous lipid emulsion, bicarbonate and tailored liposomes in rabbit clomipramine toxicity. Acad Emerg Med. 2013;20:1076–1079.
- Forster V, Paola L, LeRoux JC. Treatment of calcium channel blocker-induced cardiovascular toxicity with drug scavenging liposomes. Biomaterials. 2012;33:3578–3585.
- The Extracorporeal Treatments In Poisoning workgroup. EXTRIP workgroup recommendations; [cited 2018 Apr 7]. Available from: http://www.extrip-workgroup.org/recommendations
- Forster V, Signorell R, Roveri M, et al. Liposome supported peritoneal dialysis for detoxification of drugs and endogenous metabolites. Sci Transl Med. 2014;6:258ra141
- Chapman R, Harvey M, Davies P, et al. Liposome supported peritoneal dialysis in rat amitriptyline exposure with and without intravenous lipid emulsion. J Liposome Res. 2017;1.
- Oshima N, Kotaki H, Sawada Y, Iga T. Tissue distribution and metabolism after repeated amitriptyline administration in rats. Drug Metab Distribut. 1994;22:21–25.
- Cave G, Raghavan M, Burrows J, et al. Liposomal binding of imipramine in human red cell/albumin solution with simulated plasmapheresis. J Pharm Technol Drug Res. . 2013;2.
- Howell B, Chauhan A. Uptake of amitriptyline and nortriptyline with liposomes, proteins, and serum: Implications for drug detoxification. J Colloidal Interface Sci. 2008;319:81–93.
- Sweet CS, Emmert SE, Seymour AA, et al. Measurement of cardiac output in anesthetized rats by dye dilution using a fibreoptic catheter. J Pharmacol Methods. 1987;17:189–203.
- Grzegorzewska AE, Moore HL, Nolph KD, et al. Ultrafiltration and effective peritoneal blood flow during peritoneal dialysis in the rat. Kidney Int.. 1991;39:608–617.
- Perichon D, Turfus S, Gerostamoulos D, et al. An assessment of the in vivo effects of intravenous lipid emulsion on blood drug concentration and haemodynamics following oro-gastric amitriptyline overdose. Clin Toxicol. 2013;51:208–215.
- Lenth R. (2006–2009). Java Applets for Power and Sample Size [Computer software]. Available from: http://www.stat.uiowa.edu/rlenth/Power
- Ghannoum M, et al. A stepwise approach for the management of poisoning using extracorporeal treatments. Semin Dial.. 2014;27:362–370.
- Chemicalize.com. Calculation amitriptyline. Available from https://chemicalize.com/#/calculation
- Buckley N, Dawson A . The intralipid genie is out of the bottle-spin and wishful thinking. Anaesth Intensive Care . 2013;41:154–156.
- Pratsinis A, Zuercher S, Fischer V, et al. Liposome-supported enzymatic peritoneal dialysis. Biomaterials 2017;145:128.
- Churchwell MD, Pasko DA, Smoyer WE, et al. Enhanced clearance of highly protein bound drugs by albumin supplemented dialysate during modelled continuous haemodialysis. Nephrol Dialy Transplant. 2008;24:231–238.