Abstract
Numerous studies have analyzed the distribution and concentration of microplastic particles (MPs) in various aquatic habitats, and estimated how they affect residual biota. Decidedly less research concerns how organisms (e.g. ichthyofauna) affect the quantitative and qualitative characteristics of MPs in the water. Therefore, the aim of our study was to test, if fish can increase the vertical flux of MPs to sediments. This would be the result of the combined effect of increased sedimentation rate and the active transport resulting from vertical migration of the fish in the simultaneous presence of a thermal gradient (and in turn water density gradient). We conducted eight 5-day-long experiments in thermally stratified twin vertical columns filled with a suspension of polystyrene beads of 25 and 250 µm in size, with initial density of 1000 and 200 × L−1, respectively. In each of the experiments, we placed sediment traps at three different depths in each of the columns, and four planktivorous fish in one of them. During the experiments, we monitored the distribution of fish. At the end of the experiment, we determined the number of MPs and the amount of detritus in both sediment traps and water. We found that the presence of planktivorous fish significantly increased the vertical flux of MPs to sediments and decreased the density of MPs in the water column. These effects seem to be more likely due to the increased sedimentation rate of MPs due to the fish activity, rather than to their active transport, as MPs in the experimental variant were covered with detritus to a greater extent, than in the control, and the fish remained in the subsurface layer in the water column. Our study seems to be the first one showcasing the effect of fish on the concentration and distribution of MPs in aquatic ecosystems.
Highlights
Fish foraging activity increased the sedimentation of PS MPs.
Fish foraging activity decreased the PS MP concentration in the water column.
The study seems to provide the first experimental evidence on the effect of fish on the distribution of MPs in aquatic environments.
1. Introduction
Plastic is a type of material consisting of a broad-spectrum of synthetic or natural organic compounds, usually polymers of high molecular mass (e.g. polyethylene, polypropylene, polyhydroxybutyrate). Within the last decade, great attention has been directed toward the proliferation and potential environmental impact of microplastics (MPs), which are usually defined as plastic particles smaller than 5 mm in the largest dimension (Thompson et al. Citation2009).
At least three reasons are responsible for the fact that MPs pollution has become an urgent scientific topic, particularly in aquatic habitats. First, MP particles have been identified as a more common fraction in terms of concentration and mass in relation to larger fractions (e.g. Eriksen et al. Citation2014). Second, they have a greater potential biological effect on marine and freshwater ecosystems, since smaller particles can be more easily ingested by organisms, such as zooplankton (Cole et al. Citation2013) and fish (Choy & Drazen Citation2013). Third, because plastics are suitable matrices for bacterial attachment and growth (Harrison et al. Citation2014), MPs can be a base for a larger numbers of microorganisms due to greater area-to-volume ratio of a single particle, compared to larger plastic fractions (Ahmed et al. Citation2021). Moreover, the larger surface area may result in greater adsorption of contaminants and a greater release of additives.
A great number of studies revealed that many organisms in natural environments ingest MPs (Blair et al. Citation2017), and that the harmfulness of ingested MPs is apparent in all levels of biological organization, from molecular/subcellular (e.g. Imhof et al. Citation2017; Jeong et al. Citation2017), cellular (e.g. von Moos et al. Citation2012; Ramsperger et al. Citation2022), physiological (e.g. Stienbarger et al. Citation2021; Mkuye et al. Citation2022) and organismal (e.g. Cole et al. Citation2013; Imhof et al. Citation2017) through population (e.g. Martins & Guilhermino Citation2018; Ma et al. Citation2019) and community (e.g. Li et al. Citation2022; Zebrowski et al. Citation2022) level, depends on particle type, size, density or even colour, and may stem from mechanical and chemical effects (Maszczyk et al. Citation2022). Mechanical harmfulness may consist of clogging the digestive tract (e.g. Jovanović Citation2017) or adhering to external surfaces, thereby hindering mobility (e.g. Frydkjær et al. Citation2017). Chemical harmfulness may result from both the presence of additives (e.g. plasticizers antioxidants, flame retardants and UV stabilizers) that have the potential to leach into the environment causing damage to organisms, and the ability to accumulate harmful hydrophobic substances from the surrounding water (Hong et al. Citation2017; Fackelmann & Sommer Citation2019). Much lower effort has been made to assess the inverse relationship, i.e. how organisms affect on the qualitative and quantitative characteristics of MPs.
For at least two reasons, determining the influence of organisms on the qualitative and quantitative characteristics of MPs seems to be an important issue. First, altering these characteristics may have implications for the biology of aquatic organisms (Phuong et al. Citation2016). For example, biochemical degradation can release dissolved and toxic organic substances, such as flame retardants (Song et al. Citation2021), plasticisers (Schrank et al. Citation2019), antioxidants (Zhao et al. Citation2020) and UV stabilisers (Li et al. Citation2019). In addition, organisms, by crushing MPs into smaller fractions – which directly affects the greater likelihood of ingestion by other organisms – can also change its microstructure. This change may in turn contribute to an increase in the number of available microhabitats for microorganisms, e.g. bacteria, and as a result, cause a significant change in the microbiota, both free-living and present in the digestive system of animals (Fackelmann & Sommer Citation2019). Secondly, the presence of organisms can affect the circulation of MPs in the environment, including its removal from the water column by deposition in sediments.
To date, interest in the effect of organisms on the qualitative and quantitative characteristics of MPs focused mainly on microorganisms, including fungi (e.g. Paço et al. Citation2017) and bacteria (e.g. Auta et al. Citation2018; Park & Kim Citation2019), as the biota that contribute to the biodegradation of MPs. Another example concerns the formation of biofilms on the MP surface (Oberbeckmann et al. Citation2015) affecting the physical properties of microplastics (Rummel et al. Citation2017). It has been shown, for example, that the sedimentation rate of MPs suspended in the water column may be higher when they are surrounded by phytoplankton aggregates (Chaetoceros neogracile and Rhodomonas salina), which increase their total density (Long et al. Citation2015). The general fouling of organic matter suspended in the water column (biofouling) also increases the rate of their sedimentation (Kaiser et al. Citation2017). Fewer studies focused on the effect of freshwater and marine invertebrates, including zooplankton (Cole et al. Citation2016; Katija et al. Citation2017; Dawson et al. Citation2018; Bonello et al. Citation2021), and benthic animals (Jang et al. Citation2018; Arossa et al. Citation2019; Bulleri et al. Citation2021; Coppock et al. Citation2021). For instance, it has been revealed that polychaetes (Marphysa sanguinea) can affect both the qualitative and quantitative characteristics of MPs by damaging expanded polystyrene marine debris (Jang et al. Citation2018) producing a huge number of new, smaller MP particles. Another example concerns Antarctic krill (Euphausia superba) which may affect qualitative characteristic of MPs by turning microplastic into nanoplastic trough digestive fragmentation (Dawson et al. Citation2018). Surprisingly, according to our knowledge, the literature does not provide any direct evidence on the effect of aquatic vertebrates, such as a pelagic fish, on the qualitative and quantitative characteristics of MPs, in spite of the fact that the literature provides numerous experimental and observational evidence that they play a key role in the circulation of matter in most freshwater and marine ecosystems (Holmlund & Hammer Citation1999; Villéger et al., Citation2017), and that they ingest MPs present in the environment (Collard et al. Citation2017), excrete them in faeces (Cole et al. Citation2016; Tanaka & Takada Citation2016; Lopes et al. Citation2020; Li et al. Citation2021) and that their faeces have greater density than MPs (Coppock et al. Citation2019).
Our aim was to test the hypothesis that the presence of fish affects on the distribution of MPs suspended in the water column by increasing the vertical flux of MPs to the sediment.
2. Methods
2.1. The approach
In order to test the hypothesis, we performed eight main experiments and a single additional experiment, each lasted five days and was performed in a system comprising a pair of vertical twin columns. The main experiments were performed with the presence of the smaller and larger fractions of MPs in both columns (as a first variable), and with the presence of fish in one of the columns (as a second variable). The experiments were done to assess the effect of fish on the sedimentation and distribution of MPs in the water column. An additional experiment was performed to determine the effect of fish on the sedimentation and distribution of organic matter. This experiment was performed in the same conditions as the main experiments; the only exception was that MPs were not added into the columns.
2.2. Experimental animals
All of the experiments we performed using four juvenile (0+) rudd (Scardinius erythrophthalmus; length 55 ± 5 mm), which are a pelagic freshwater herbivorous species, however in the juvenile stage they feed mainly on zooplankton. All the individuals used in the experiments were hatched at the Inland Fisheries Institute in Żabieniec, Poland. Two weeks before the start of the first experiment, 40 fish were transported to the fish breeding room at the Department of Hydrobiology, Faculty of Biology at the Biological and Chemical Research Centre, University of Warsaw. In both places, the fish were under the constant control of ichthyologists, who daily monitored the water parameters (temperature, oxygen concentration, pH) and the condition of fish (general activity, feeding behaviour, symptoms of fungal diseases). The fish were kept in a 16 L: 8 D summer photoperiod in aquariums filled with aerated tap water connected to a biological treatment system. During the holding of the fish in the laboratory of Department of Hydrobiology, their contact with plastics was minimized. In each of the experiments, we used a new group of fish consisting of four individuals.
2.3. Characteristics of microplastics used in the experiments
In the main experiments, we used a mixture of two MP fractions: (1) 23.3 µm in diameter (cat. no. FGP20K) and (2) 250 µm in diameter (cat. no. FGP250K) of green-fluorescent polystyrene (PS) beads of density similar to water (1.05 g × cm−3), purchased from Lab261®. The concentrations chosen for this study (1000 and 200 particles × L−1), respectively, can occasionally be found in aquatic environments (Stanton et al. Citation2020). The initial number of MPs was determined on subsamples, using a fluorescent microscope (Merazet MT6200). Polystyrene is widely used in various industries, e.g. for the production of packaging. It is one of the predominant types of plastic in the aquatic environment (Hwang et al. Citation2020).
2.4. Experimental system
The experimental system consisted of two identical, vertical, nontransparent PVC columns (depth = 1.8 m, diameter = 0.47 m) with a volume of 200 litres (filled up to 180 L; )). The inner walls of the columns were covered with a 2 mm thick layer of stainless steel. Inside the columns we reflected the conditions of a typical temperate dimictic lake, during summer stratification (i.e. brighter, warmer and aerated upper zone corresponding with epilimnion and darker, cooler and oxygen-free bottom zone corresponding with hypolimnion), albeit on a reduced scale (). To create a light gradient in the water column in each of the columns, we used an opaque steel lampshade with an LED bulb (EMOS 14 W, with light temperature 3000 K) installed tightly, adjacent to the column and separated from it by a light diffusing screen. The light was set to mimic a summer photoperiod (16 D: 8 N). The temperature gradient in each of the columns was obtained by heating the upper layers and cooling the lower layers using a system of steel pipes. Each of the steel pipes had a diameter of 1 cm forming 3 rings around the inner wall of each column at a height of 35, 55 and 170 cm, tightly connected pairs of silicone tubes outside the system (one being an inlet with the EHEIM 1000.220 water pump connected at its end and the other being a drain), connected with one of the 3 distilled water tanks. In one of the tanks there was a thermostat (ADA-REX ZEFIR) heating the water supplied through a pipe system to a depth of 35 cm, and in each of the other two tanks there was a cooler (AQUA MEDIC TITAN 500) to cool the water, which was supplied by a system of pipes to the depth of 55 and 170 cm. At three depths (25, 55 and 160 cm), in each of the columns, we installed four sets of sediment traps. A single set consisted on three glass tubes of 8 ml volume and 1 cm diameter. The analyzed sample was the content of a single set of traps. The sets of sediment traps were held at a given depth by a steel hanger made of rods and fixed at the top of each column, above the water surface. Horizontal arrangement of traps was set up in a way that they did not affect the number of particles sedimenting into the traps at lower depths. To assess the fish distribution in the water column during the experiments, two high-resolution miniature underwater cameras (P. P. H. Matar KT-370/540) were placed at the depths of 15 and 50 cm in the experimental column.
2.5. Experimental procedure
A day before each of the experiments, filtered tap water (through polypropylene fibre filter 1 µm FCPS1, AquaFilter, USA) was pumped to the columns up to 160 L. The water was aerated for 6 h using an aquarium oxygenator (Aqua Lifter AQ-20 Dosing Pump), until the water was completely saturated with oxygen, which was monitored using an oxygen probe (YSI ProODO). Then, sedimentation traps were installed. The circulating system was switched on and, over next 12 h, distilled water in each of the 3 rings was circulated to obtain a thermal gradient. Six hours before the start of the experiments, 4 fish (randomly selected) were introduced into one of the columns for acclimation. The control and experimental variants were alternately fixed in the columns (4 times in each of the columns). The main experiments started with the addition of MP suspensions (both MP fractions suspended in 100 ml of tap water) to the upper layer of the water column manually. The additional experiment was started by adding 100 ml tap water without MPs. Then, lampshades were put on each of the columns. During the experiments (both main and additional), the fish were fed 6 times per day light (every 2 h) with a small portion (0.12 g) of dried aquarium pellets (Tropical – SuperVit ®) added to the surface of the water columns. As the pellets are lighter than the water, they floated on the surface. The food in the column without fish crumbled into microscopic pieces and floated in the water. The amount and frequency of added food was determined in preliminary tests so that it was enough, on the one hand, to be eaten very quickly (up to 2 min), and on the other hand, to observe the hypothetical effect of fish defecation on the sedimentation of MPs. Every day, the temperature and the oxygen concentration were measured in the columns (at several depths, every 10 cm) by using an oxygen probe (YSI ProODO). Within 10 min before and 10 min after each of feeding procedures, vertical distribution of fish was monitored using two underwater cameras. A 10-min time interval was enough to count the fish at each depth ten times (in minute intervals). The behaviour of the fish during the 10-min pre-feeding period was representative to the behaviour of the fish for the duration of the experiment except for the first 10 min after adding the food, when the fish exhibited foraging behaviour and moved dynamically between different depths. The experiment was finished by switching off the light in columns. Then, the samples were taken using a night vision device. The duration of the experiments was set so that the amount of detritus in the water column at the end of experiments did not exceed the amount of detritus in the pelagic zone of a typical eutrophic lake (e.g. Gervais et al. Citation1999; Vargas-Sánchez et al. Citation2022).
In the main experiments, 15 ml of water samples were taken from 2 depths (0 and 60 cm) from each of the columns using syringes with a plastic tube loaded with a steel tip for further analysis of the number of bacteria. Each sample was fixed by adding a formaldehyde solution (final concentration 2%) and analysed within 14 days using epifluorescence microscopy. Then, another 1 litre of water samples were taken from each of the seven depths (0, 30, 60, 90, 120, 160 and 170 cm) for the further estimation of MP concentration. The samples were siphoned, starting from the highest depths and concentrated by pouring them through a 20 μm mesh strainer. Next, the sediment traps were removed and their content was poured into 250 ml containers for further enumeration of the MPs. Finally, the fish were removed from one of the columns with a 6 mm mesh net.
In the additional experiment, 1 litre of samples from each of four different depths (0, 60, 120 and 160 cm) was taken to assess the distribution of DW (of all particles > 0.45 µm) in the water column. Next, the sediment traps were removed and their content was poured into 250 ml containers for further assessment of the sedimentation rate of the DW. Finally, the fish were removed from the experimental column.
The experimental procedure was approved by the First Warsaw Local Ethics Committee for Animal Experimentation (Permit protocol No. 1350P1/2022).
2.6. Sample and data analysis
The number of both fractions of green fluorescent MPs from the water column samples and from the sediment traps was counted on Hydrobios® microscopic plankton counting chambers with fluorescent microscope (Merazet MT6200) using UV light. The sedimentation rate was calculated as the mean number of particles sedimenting at a given depth per one day of an experiment.
In order to determine the number of bacteria, we used standard DAPI staining (Porter & Feig Citation1980). For this purpose, we diluted 1 ml of each sample in 9 ml of miliQ water and added 200 µl of 50 µg × ml−1 DAPI (4′,6-diamidino-2-phenylindole). Next, we kept it in darkness for 15 min. Then, we filtered the stained sample through polycarbonate, a black membrane filter with 0.2 µm pores (Nucleopore®). The density of bacteria was calculated using the formula: Db = nb × nf −1 × Nf × V −1, where: nb is the summary number of bacteria in the fields of view, nf is the number of fields of view counted (14), Nf is the total number of fields of view on the filter (the ratio of the total filter area to the field of view area = 43 388), and V is the volume of the subsample (1 ml).
The vertical distribution of fish was determined manually on the recorded videos during 10 min before and 10 min after the food was introduced to the columns in three depth ranges (0–45, 45–60 and 60–160 cm).
To assess the DW in both the water column and sediment traps, the samples were first filtered using dried (40°C in a Thermocenter TC100 dryer, Salvislab®) and weighted (on Radwag XA 110/2X®) microfiber 0.45 µm filters (MF-Millipore® Membrane Filter). Next, the filters were dried in 40°C for 48 h and weighted. The DW in each of the samples was determined as the difference between the filter mass with and without the filtrate.
The statistical analysis was performed using R platform (v.4.2.0) by setting the level of significance at α = 0.05 for all of the statistics. Since the assumption of normality was not met for the response variable, we used non-parametric mixed effects models for the analysis of data.
To test the difference in the abiotic parameters (light intensity, temperature gradient and oxygen concentration), number of bacteria and dry weight (DW) distribution between columns and within each column, we performed mixed ANOVA for 10% trimmed means. We used the “bwtrim” function (“WRS2” package v. 1.1–4; Mair & Wilcox Citation2020) which allowed to fit the model despite non-normality and heteroscedasticity of initial data distribution. The experimental treatment (experimental – fish vs. control – no fish) was set as a between-subjects factor and the depth set a within-subjects factor. The post-hoc tests were conducted using the Yuen method for 10% trimmed means, and “yuen” or “yuend” functions for independent (between-subject) or dependent (within-subject) group comparisons, respectively.
To assess the effect of fish on the distribution of both fractions of MPs in the water column and in traps, we employed Generalized Linear Mixed Models with Poisson family and log link (GLMMs; Bolker et al. Citation2009), where the presence of the fish, the depth and the MP size were considered as fixed effects while the water column was considered as a random effect. The significance of the interaction between the factors was assessed using the analysis of deviance and Type II Wald Chi-square (χ2) difference test (car package v.3.0–12; Fox & Weisberg Citation2011). Contrasts for estimated marginal means (EMMs; the emmeans package) were used to test the significance of the effect of fish on the MP distribution between the experimental and control treatments separately for each depth and separately for each of the two fractions of MPs. The “holm” correction was used to adjust the p-value in the multiple comparisons.
3. Results
3.1. Experimental conditions
There was no significant difference for light intensity and temperature gradients in the water column between the control and experimental variant – the presence of the fish (Table S1, ). The oxygen concentration differed significantly between the experimental variant and control (Table S1, Supplementary material, ). Light intensity and temperature differed significantly with depth (Table S1, ), being greater in “epilimnion” (0–30 cm) than in “metalimnion” (40–60 cm) and “hypolimnion” (70–160 cm) and in “metalimnion” in comparison to “hypolimnion” (Table S2, ). Also, oxygen concentration differed significantly with depth (Table S1, ). The difference was non significantly between “epilimnion” and “metalimnion” and was significantly lower in the “metalimnion” than “hypolimnion” (Table S2, ).
3.2. The distribution and sedimentation rate of MPs
The distribution of MPs in the water column differed throughout the measured depths, while the main effect of the fish and MPs size was not significant (). The distribution of MPs differed significantly with the depth between control and experimental variant (significant interaction Tr × Dp, ), and the difference was affected by the size of MPs (significant interaction Tr × Dp × Size, ). The concentration of large MPs was significantly greater in the control than in experimental variant in two measured depths (at 0 and 30 cm) and the concentration of small MPs was significantly greater in the experimental variant in the bottom of the column (at 160 cm, Table S3, ).
Figure 2. Relative concentration (%) of large (a) and small (b) MPs in the water column in the control (filled box, mean + 1 SD) and experimental conditions (empty box, mean _ 1 SD) and the number of large (c) and small (d) MPs in sediment traps in the control (dotted line, mean _ 1 SD) and experimental conditions (solid line, mean + 1 SD).
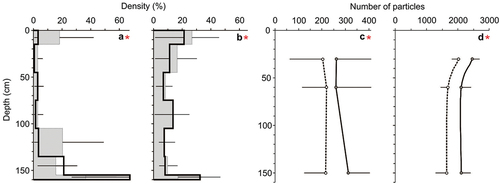
Table I. The results of the analysis of deviance (Type II Wald chi-square tests for model-fixed effects) of the generalised linear mixed effects model (GLME) to test the effect of experimental condition (Tr), depth (Dp), MP size (Size), the Tr × Dp, Tr × Size, Dp × Size and Tr × Dp × Size interactions on the distribution in water column and sedimentation rate of (MPs). The χ2 is Chi-squared statistic associated with a P-value (Pr(> χ2)). Statistically significant differences are marked in bold (df – degree of freedom).
The sedimentation rate differed significantly between the control and experimental variant, between depths and between two size classes of MPs (). The sedimentation rate of MPs differed significantly with the depth between control and experimental variant (significant interaction Tr × Dp, ), and the difference was affected by the size of MPs (significant interaction Tr × Dp × Size, ). The sedimentation rate was significantly greater in the experimental variant than in control only at 150 cm in the case of large MPs, and in all depths (30, 60 and 150 cm) in the case of small MPs (Table S3).
3.3. The distribution of bacteria
Overall, the abundance of bacteria was not affected by the fish presence, but their distribution varied between depths. Generally, the presence of the fish had the impact on the bacteria distribution across the depth (significant interaction Tr × Dp, ), and the difference was affected by the size of MPs (significant interaction Tr × Dp × Size, ). The density of bacteria was affected by the fish presence in each of the two depths, being greater at 60 and lower at 0 cm (Table S4).
Table II. The results of the two-way mixed ANOVA for trimmed means to test the effect of the presence of fish on the bacterial abundances with treatment (Tr) as between observations factor and depth (Dp) as within observations factors. Statistically significant differences are marked in bold (df – degree of freedom, Q – Q statistics, P corr. – p-value corrected).
3.4. The distribution and sedimentation rate of particulate matter
Overall, the presence of the fish affected the distribution of particulate matter suspended in the water and its sedimentation rate (). More specifically, the distribution of particulate matter was relatively greater in the control (than in the fish presence) at the middle depths (60 and 120 cm) and was relatively greater in the fish presence (than in the control) at the bottom water layer (160 cm; Table S5, )), while its sedimentation rate was greater in the fish presence (than in the control) for all measured depths (30, 60 and 120 cm; Table S5, )).
Table III. The results of the two-way mixed ANOVA for trimmed means to test the effect of the presence of fish on the DW distribution in the water column and the DW sedimentation rate with treatment (Tr) as between observation factor and depth (Dp) as within observation factors. Statistically significant differences are marked in bold (df – degree of freedom, Q – Q statistics, P corr. – p-value corrected).
4. Discussion
The results of our study confirmed the general hypothesis, as the presence of the fish resulted in an increase of the vertical flux of polystyrene MPs to the sediments and in a decrease of their concentration in the water column. In accordance with the state of our knowledge, this is the first experimental evidence on the effect of fish on the concentration and distribution of MPs in aquatic environments. In our study, the MPs were surrounded by detrital organic matter, including faecal pellets ()). Therefore, the results seem to be important in the context of the self-purification of lake water from MPs, since the fish are responsible for a significant part of the vertical transport of biologically generated particulate organic matter out of the marine and freshwater euphotic zone (e.g. Saba et al. Citation2021). This reasoning is in line with our observation that the presence of fish results in an increase of particulate matter concentration in the bottom layer of the water column. The results also seem to be important in the context of a putative significant role of fish in decreasing the availability of MPs for organisms living in the water column (e.g. copepods, jellyfish, decapod larvae, amphipods, rotifers and cladocerans) and in increasing it for benthic organisms (e.g. clams and larvae of various insects). Our results are in accordance with recent theoretical (e.g. Berezina et al. Citation2021) and field (e.g. Van Colen et al. Citation2021) studies concerning the effect of invertebrates and particular matter on the fate of MPs in the environment, which provided arguments for the functioning of a “biological pump” as one of the important drivers controlling the abundance and distribution of MPs in the water column. It should be pointed out that the general distribution of MPs in the water may also be affected by horizontal migrations of fish, which were not investigated in our study.
Figure 4. Fish faecal pellet with a single large particle and a few small MPs extracted from the sediment trap in the experimental treatment photographed under the UV microscope (Nikon Eclipse E450) with 4 × magnification (a) and a single particle of MPs extracted from the water in the experimental treatment (b) and in control (c) photographed by the UV microscope (Nikon Eclipse E450) with 8 × magnification.
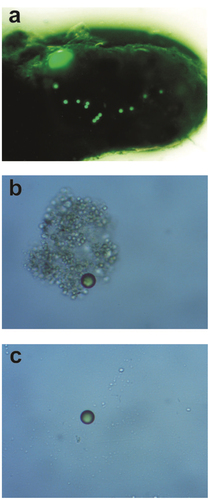
As at the end of our experiment, relatively more larger (250 µm) MPs in relation to the smaller ones (25 µm) were in the bottom layers of the water columns, it could be assumed that the sedimentation rate of the larger particles was greater than smaller ones. This is consistent with the results obtained in the earlier studies (Bergmann et al. Citation2017; Haave et. al. Citation2019) and may be due to their lower susceptibility to micro-turbulence in water (Zhang Citation2017) and a greater chance of their ingestion as a result of a mismatch between the MPs and the natural prey of fish (Peters et al. Citation2017; Ory et al. Citation2018). The effect of fish on the vertical flux of MPs may also depend on the relative contribution of active transport, consisting of the migration of fish into deeper layers of water, which deposit MPs bound in the faecal material in relation to the passive transport, consisting of the ingestion of MPs and the production of faecal material containing MPs with a total density greater than the density of the MPs themselves. It is very likely that in our study, the net effect of fish was mainly due to passive transport, as the fish continuously resided in the subsurface layers of the water throughout the experiments.
It could be assumed that in addition to the particle size and the relative contribution of active and passive transport of MPs by fish, several other factors not studied in our research, including the polymer type and its shape as well as the feeding mode of fish and water conditions (e.g. temperature and salinity) may contribute to the effect of fish on the vertical flux. The effect may depend on the polymer type due to their specific density in relation to the density of water. The polystyrene used in our study, which is ubiquitous in most freshwater (Li et al. Citation2020) and marine ecosystems (Do Sul & Costa Citation2014), has slightly greater density than water, therefore it can sediment itself, however rather slowly (de la Fuente et al. Citation2017). It seems that the effect of fish on the vertical flux of the other commonly found polymer types in the environment like polyethylene, polypropylene characterised by positive buoyancy in water, may be even stronger, because their sedimentation depends on attached heavier particles of particulate matter. Other properties of the MPs that we have not studied were their shape. Micro-beads seem to have the fastest sedimentation rate in comparison to other shapes of regular microparticles (Khatmullina & Isachenko Citation2017). Thus, the effect of fish presence would be more apparent in case of particles with different, less streamlined shapes, for instance rods or irregular fragments. Moreover, the effect may be different for fish with different feeding modes (Valente et al. Citation2022). For instance, it can be assumed that filter-feeder fish can ingest relatively greater amount of small particles over the larger ones compared to the planktivorous fish used in our study, whereby the sedimentation rate of the small particles may increase. Additionally, it can be expected that this effect can also be modified by water parameters such as temperature and salinity. Elevated temperature increases the rate of fish foraging (e.g. Persson Citation1986; Gliwicz & Maszczyk Citation2016) and this, in turn, may also lead to an increase of both passive and active transport of MPs. Higher water salinity and thus denser water slows down the sedimentation rate of particles including MPs (e.g. Uurasjärvi et al. Citation2021), which may increase the role of active transport by marine fish in the vertical flux of MPs, where MP buoyancy in salt water is higher than in freshwater and they need a greater load to sediment.
In our study, the presence of fish affected the distribution of the bacteria in each of the two depths, with greater number of bacteria at 60 and lower at subsurface water layers, which is consistent with other studies, where it was reported, that the presence of fish increases the number of free-living bacteria in ambient water (Maszczyk & Bartosiewicz Citation2012; Harrison et al. Citation2014). We cannot exclude the possibility, that the presence of fish also resulted in the increased number of bacteria attached to the MPs (). Even if the number of bacteria colonising MPs in our experiment was too small to change the relative density of the colonised particle and to change its sedimentation properties (Kaiser et al. Citation2017), the presence of bacteria on the surface of a particle may facilitate the binding of the organic matter produced by the fish (Arias-Andres et al. Citation2019). Moreover, the effect of fish on the bacterial density increased with depth, which coincides with the increase of the effect of fish on the particulate matter content in water. However, the interpretation of the results concerning the distribution of bacteria should be treated with caution, as we measured the density only at two depths.
Despite numerous factors putatively increasing the variability within the net effect of the fish on the sedimentation rate and, in turn, the concentration of MPs in the water, our study clearly demonstrated the importance of fish foraging in increasing the vertical flux of MPs to the sediments. Increased vertical flux of MPs due to fish presence and their foraging activity may combine with the effects of other processes previously described, which also may have a significant contribution in the observed effect, including microbial or phytoplankton attachment and colonization on the MPs (Zettler et al. Citation2013; Harrison et al. Citation2014; Long et al. Citation2015) and MP egestion in zooplankton faeces (Cole et al. Citation2016; Bonello et al. Citation2021). Our study, in addition to the aforementioned studies, may provide an explanation on the lower density of floating MPs and their greater concentrations in sediments than stems from conservative estimates (Cózar et al. Citation2014; Eriksen et al. Citation2014; Woodall et al. Citation2014). Our results provide new insights for water management and can be incorporated into several types of models, which would take fish into account as vectors in the freshwater transport pathways of MPs and their potential mechanical and biochemical effects on the concentration and qualitative features of MPs.
Author contributions
Conceptualization, E.B. and P.M.; Methodology, S.P., P.M.; Software, M.Z.; Validation, S.P., M.Z. and P.M.; Formal Analysis, S.P.; Investigation, S.P., K.D., M.Z.; Resources, E.B., P.M.; Data Curation, M.Z.; Writing – Original Draft Preparation, S.P.; Writing – Review and Editing, S.P. E.B. P.M, M.Z. Visualization, M.Z.; Supervision, P.M., E.B.; Project Administration, E.B., P.M.; Funding Acquisition, E.B., P.M.
Supplemental Material
Download MS Word (36.8 KB)Acknowledgements
We are grateful to Anna Wiewiorowska and Julia Wawrzenczak for assistance in the experimental work, Mark Kudriashov for technical support and Tomasz Brzezinski and Wojciech Wilczynski for comments on an earlier draft of this manuscript.
Disclosure statement
No potential conflict of interest was reported by the author(s).
Data availability statement
The data presented in this study are available on request from the corresponding author.
Supplementary material
Supplemental data for this article can be accessed online at https://doi.org/10.1080/24750263.2023.2217199
Additional information
Funding
References
- Ahmed MB, Rahman MS, Alom J, Hasan MS, Johir MAH, Mondal MIH, Lee DY, Park J, Zhou JL, Yoon MH. 2021. Microplastic particles in the aquatic environment: A systematic review. Science of the Total Environment 775:145793. DOI: 10.1016/j.scitotenv.2021.145793.
- Arias-Andres M, Rojas-Jimenez K, Grossart HP. 2019. Collateral effects of microplastic pollution on aquatic microorganisms: An ecological perspective. TrAC Trends in Analytical Chemistry 112:234–240. DOI: 10.1016/j.trac.2018.11.041.
- Arossa S, Martin C, Rossbach S, Duarte CM. 2019. Microplastic removal by Red Sea giant clam (Tridacna maxima). Environmental Pollution 252:1257–1266. DOI: 10.1016/j.envpol.2019.05.149.
- Auta HS, Emenike CU, Jayanthi B, Fauziah SH. 2018. Growth kinetics and biodeterioration of polypropylene microplastics by Bacillus sp. and Rhodococcus sp. isolated from mangrove sediment. Marine Pollution Bulletin 127:15–21. DOI: 10.1016/j.marpolbul.2017.11.036.
- Berezina A, Yakushev E, Savchuk O, Vogelsang C, Staalstrom A. 2021. Modelling the influence from biota and organic matter on the transport dynamics of microplastics in the water column and bottom sediments in the Oslo fjord. Water 13(19):2690. DOI:10.3390/w13192690.
- Bergmann M, Wirzberger V, Krumpen T, Lorenz C, Primpke S, Tekman MB, Gerdts G. 2017. High quantities of microplastic in Arctic deep-sea sediments from the HAUSGARTEN observatory. Environmental Science and Technology 51(19):11000–11010. DOI:10.1021/acs.est.7b03331.
- Blair RM, Waldron S, Phoenix V, Gauchotte-Lindsay C. 2017. Micro-and nanoplastic pollution of freshwater and wastewater treatment systems. Springer Science Reviews 5(1):19–30. DOI:10.1007/s40362-017-0044-7.
- Bolker BM, Brooks ME, Clark CJ, Geange SW, Poulsen JR, Stevens MHH, White JSS. 2009. Generalized linear mixed models: A practical guide for ecology and evolution. Trends in Ecology and Evolution 24(3):127–135. DOI:10.1016/j.tree.2008.10.008.
- Bonello G, Zanetti L, Carpi L, Mucerino L, Pane L. 2021. The role of Crassostrea gigas (Thunberg, 1793) in the vertical microplastic transfer: A plankton-benthos linkage laboratory protocol. Food Webs 27:e00189. DOI: 10.1016/j.fooweb.2021.e00189.
- Bulleri F, Ravaglioli C, Anselmi S, Renzi M. 2021. The sea cucumber Holothuria tubulosa does not reduce the size of microplastics but enhances their resuspension in the water column. Science of the Total Environment 781:146650. DOI: 10.1016/j.scitotenv.2021.146650.
- Choy CA, Drazen JC. 2013. Plastic for dinner? Observations of frequent debris ingestion by pelagic predatory fishes from the central North Pacific. Marine Ecology Progress Series 485:155–163. DOI: 10.3354/meps10342.
- Cole M, Lindeque PK, Fileman E, Clark J, Lewis C, Halsband C, Galloway TS. 2016. Microplastics alter the properties and sinking rates of zooplankton faecal pellets. Environmental Science and Technology 50(6):3239–3246. DOI:10.1021/acs.est.5b05905.
- Cole M, Lindeque P, Fileman E, Halsband C, Goodhead R, Moger J, Galloway TS. 2013. Microplastic ingestion by zooplankton. Environmental Science and Technology 47(12):6646–6655. DOI:10.1021/es400663f.
- Collard F, Gilbert B, Compère P, Eppe G, Das K, Jauniaux T, Parmentier E. 2017. Microplastics in livers of European anchovies (Engraulis encrasicolus, L.). Environmental Pollution 229:1000–1005. DOI: 10.1016/j.envpol.2017.07.089.
- Coppock RL, Galloway TS, Cole M, Fileman ES, Queirós AM, Lindeque PK. 2019. Microplastics alter feeding selectivity and faecal density in the copepod, Calanus helgolandicus. Science of the Total Environment 687:780–789. DOI: 10.1016/j.scitotenv.2019.06.009.
- Coppock RL, Lindeque PK, Cole M, Galloway TS, Näkki P, Birgani H, Richards S, Queirós AM. 2021. Benthic fauna contribute to microplastic sequestration in coastal sediments. Journal of Hazardous Materials 415:125583. DOI: 10.1016/j.jhazmat.2021.125583.
- Cózar A, Echevarría F, González-Gordillo JI, Irigoien X, Úbeda B, Hernández-León S, Palma ÁT, Navarro S, García-de-Lomas J, Ruiz A, Fernández-de-Puelles ML, Duarte CM. 2014. Plastic debris in the open ocean. Proceedings of the National Academy of Sciences 111(28):10239–10244. DOI:10.1073/pnas.1314705111.
- Dawson AL, Kawaguchi S, King CK, Townsend KA, King R, Huston WM, Nash SMB. 2018. Turning microplastics into nanoplastics through digestive fragmentation by Antarctic krill. Nature Communications 9(1):1–8. DOI:10.1038/s41467-018-03465-9.
- R, Drótos G, Hernández-García E, López C Van Sebille E 2021. Sinking microplastics in the water column: Simulations in the Mediterranean Sea. Ocean Science 17(2): 431–453.
- Do Sul JAI, Costa MF. 2014. The present and future of microplastic pollution in the marine environment. Environmental Pollution 185:352–364. DOI: 10.1016/j.envpol.2013.10.036.
- Eriksen M, Lebreton LC, Carson HS, Thiel M, Moore CJ, Borerro JC, Galgani F, Ryan PG, Reisser J. 2014. Plastic pollution in the world’s oceans: More than 5 trillion plastic pieces weighing over 250,000 tons afloat at sea. PloS One 9(12):e111913. DOI:10.1371/journal.pone.0111913.
- Fackelmann G, Sommer S. 2019. Microplastics and the gut microbiome: How chronically exposed species may suffer from gut dysbiosis. Marine Pollution Bulletin 143:193–203. DOI: 10.1016/j.marpolbul.2019.04.030.
- Fox J, Weisberg S. 2011. Multivariate linear models in R. In: An R companion to applied regression. Los Angeles: Thousand Oaks.
- Frydkjær CK, Iversen N, Roslev P. 2017. Ingestion and egestion of microplastics by the cladoceran Daphnia magna: Effects of regular and irregular shaped plastic and sorbed phenanthrene. Bulletin of Environmental Contamination and Toxicology 99(6):655–661. DOI:10.1007/s00128-017-2186-3.
- Gervais F, Berger S, Schönfelder I, Rusche R. 1999. Basic limnological characteristics of the shallow eutrophic lake Grimnitzsee (Brandenburg, Germany). Limnologica 29(2):105–119. DOI:10.1016/S0075-9511(99)80058-9.
- Gliwicz ZM, Maszczyk P. 2016. Heterogeneity in prey distribution allows for higher food intake in planktivorous fish, particularly when hot. Oecologia 180(2):383–399. DOI:10.1007/s00442-015-3485-1.
- Haave M, Lorenz C, Primpke S, Gerdts G. 2019. Different stories told by small and large microplastics in sediment-first report of microplastic concentrations in an urban recipient in Norway. Marine Pollution Bulletin 141:501–513. DOI: 10.1016/j.marpolbul.2019.02.015.
- Harrison JP, Schratzberger M, Sapp M, Osborn AM. 2014. Rapid bacterial colonization of low-density polyethylene microplastics in coastal sediment microcosms. BMC Microbiology 14(1):1–15. DOI:10.1186/s12866-014-0232-4.
- Holmlund CM, Hammer M. 1999. Ecosystem services generated by fish populations. Ecological Economics 29(2):253–268. DOI:10.1016/S0921-8009(99)00015-4.
- Hong SH, Shim WJ, Hong L. 2017. Methods of analysing chemicals associated with microplastics: A review. Analytical Methods 9(9):1361–1368. DOI:10.1039/C6AY02971J.
- Hwang J, Choi D, Han S, Jung SY, Choi J, Hong J. 2020. Potential toxicity of polystyrene microplastic particles. Scientific Reports 10(1):1–12. DOI:10.1038/s41598-020-64464-9.
- Imhof HK, Rusek J, Thiel M, Wolinska J, Laforsch C. 2017. Do microplastic particles affect Daphnia magna at the morphological, life history and molecular level? PLoS One 12(11):0187590. DOI:10.1371/journal.pone.0187590.
- Jang M, Shim WJ, Han GM, Song YK, Hong SH. 2018. Formation of microplastics by polychaetes (Marphysa sanguinea) inhabiting expanded polystyrene marine debris. Marine Pollution Bulletin 131:365–369. DOI: 10.1016/j.marpolbul.2018.04.017.
- Jeong CB, Kang HM, Lee MC, Kim DH, Han J, Hwang DS, Souissi S, Shin KK, Heum GP, Lee JS. 2017. Adverse effects of microplastics and oxidative stress induced MAPK/Nrf2 pathway-mediated defense mechanisms in the marine copepod Paracyclopina nana. Scientific Reports 7(1):1–11. DOI:10.1038/srep41323.
- Jovanović B. 2017. Ingestion of microplastics by fish and its potential consequences from a physical perspective. Integrated Environmental Assessment and Management 13(3):510–515. DOI:10.1002/ieam.1913.
- Kaiser D, Kowalski N, Waniek JJ. 2017. Effects of biofouling on the sinking behavior of microplastics. Environmental Research Letters 12(12):124003. DOI:10.1088/1748-9326/aa8e8b.
- Katija K, Choy CA, Sherlock RE, Sherman AD, Robison BH. 2017. From the surface to the seafloor: How giant larvaceans transport microplastics into the deep sea. Science Advances 3(8):e1700715. DOI:10.1126/sciadv.1700715.
- Khatmullina L, Isachenko I. 2017. Settling velocity of microplastic particles of regular shapes. Marine Pollution Bulletin 114(2):871–880. DOI:10.1016/j.marpolbul.2016.11.024.
- Li C, Busquets R, Campos LC. 2020. Assessment of microplastics in freshwater systems: A review. Science of the Total Environment 707:135578. DOI: 10.1016/j.scitotenv.2019.135578.
- Li W, Chen X, Li M, Cai Z, Gong H, Yan M. 2022. Microplastics as an aquatic pollutant affect gut microbiota within aquatic animals. Journal of Hazardous Materials 423:127094. DOI: 10.1016/j.jhazmat.2021.127094.
- Li Z, Li W, Zha J, Chen H, Martyniuk CJ, Liang X. 2019. Transcriptome analysis reveals benzotriazole ultraviolet stabilizers regulate networks related to inflammation in juvenile zebrafish (Danio rerio) brain. Environmental Toxicology 34(2):112–122. DOI:10.1002/tox.22663.
- Li J, Ouyang Z, Liu P, Zhao X, Wu R, Zhang C, Chong L, Yiyong L, Guo X. 2021. Distribution and characteristics of microplastics in the basin of Chishui River in Renhuai, China. Science of the Total Environment 773:145591. DOI: 10.1016/j.scitotenv.2021.145591.
- Long M, Moriceau B, Gallinari M, Lambert C, Huvet A, Raffray J, Soudant P. 2015. Interactions between microplastics and phytoplankton aggregates: Impact on their respective fates. Marine Chemistry 175:39–46. DOI: 10.1016/j.marchem.2015.04.003.
- Lopes C, Raimundo J, Caetano M, Garrido S. 2020. Microplastic ingestion and diet composition of planktivorous fish. Limnology and Oceanography Letters 5(1):103–112. DOI:10.1002/lol2.10144.
- Mair P, Wilcox R. 2020. Robust statistical methods in R using the WRS2 package. Behavior Research Methods 52(2):464–488. DOI:10.3758/s13428-019-01246-w.
- Martins A, Guilhermino L. 2018. Transgenerational effects and recovery of microplastics exposure in model populations of the freshwater cladoceran Daphnia magna Straus. Science of the Total Environment 631:421–428. DOI: 10.1016/j.scitotenv.2018.03.054.
- Maszczyk P, Bartosiewicz M. 2012. Threat or treat: The role of fish exudates in the growth and life history of Daphnia. Ecosphere 3(10):1–19. DOI:10.1890/ES12-00146.1.
- Maszczyk P, Pijanowska J, Mrówka P, Babkiewicz E. 2022. Effects of nanoplastics on aquatic organisms. In: Environmental nanopollutants: Sources, occurrence, analysis and fate. CRC Press. pp. 156–210. DOI:10.1039/9781839166570-00156.
- Ma P, Wei Wang M, Liu H, Feng Chen Y, Xia J. 2019. Research on ecotoxicology of microplastics on freshwater aquatic organisms. Environmental Pollutants and Bioavailability 31(1):131–137. DOI:10.1080/26395940.2019.1580151.
- Mkuye R, Gong S, Zhao L, Masanja F, Ndandala C, Bubelwa E, Yang C, Deng Y. 2022. Effects of microplastics on physiological performance of marine bivalves, potential impacts, and enlightening the future based on a comparative study. Science of the Total Environment 838:155933. DOI: 10.1016/j.scitotenv.2022.155933.
- Oberbeckmann S, Löder MG, Labrenz M. 2015. Marine microplastic-associated biofilms–a review. Environmental Chemistry 12(5):551–562. DOI:10.1071/EN15069.
- Ory NC, Gallardo C, Lenz M, Thiel M. 2018. Capture, swallowing, and egestion of microplastics by a planktivorous juvenile fish. Environmental Pollution 240:566–573. DOI: 10.1016/j.envpol.2018.04.093.
- Paço A, Duarte K, da Costa JP, Santos PS, Pereira R, Pereira ME, Freitas AC, Duarte AC, Rocha-Santos TA. 2017. Biodegradation of polyethylene microplastics by the marine fungus Zalerion maritimum. Science of the Total Environment 586:10–15. DOI: 10.1016/j.scitotenv.2017.02.017.
- Park SY, Kim CG. 2019. Biodegradation of micro-polyethylene particles by bacterial colonization of a mixed microbial consortium isolated from a landfill site. Chemosphere 222:527–533. DOI: 10.1016/j.chemosphere.2019.01.159.
- Persson L. 1986. Temperature-induced shift in foraging ability in two fish species, roach (Rutilus rutilus) and perch (Perca fluviatilis): Implications for coexistence between poikilotherms. The Journal of Animal Ecology 829–839. DOI: 10.2307/4419.
- Peters CA, Thomas PA, Rieper KB, Bratton SP. 2017. Foraging preferences influence microplastic ingestion by six marine fish species from the Texas Gulf Coast. Marine Pollution Bulletin 124(1):82–88. DOI:10.1016/j.marpolbul.2017.06.080.
- Phuong NN, Zalouk-Vergnoux A, Poirier L, Kamari A, Châtel A, Mouneyrac C, Lagarde F. 2016. Is there any consistency between the microplastics found in the field and those used in laboratory experiments? Environmental Pollution 211:111–123. DOI: 10.1016/j.envpol.2015.12.035.
- Porter KG, Feig YS. 1980. The use of DAPI for identifying and counting aquatic microflora 1. Limnology and Oceanography 25(5):943–948. DOI:10.4319/lo.1980.25.5.0943.
- Ramsperger AFRM, Jasinski J, V¨olkl M, Witzmann T, Meinhart M, J´erˆome V, Kretschmer WP, Freitag R, Senker J, Fery A, Kress H, Scheibel T, Laforsch C. 2022. Supposedly identical microplastic particles substantially differ in their material properties influencing particle–cell interactions and cellular responses. Journal of Hazardous Material 425:127961. DOI: 10.1016/j.jhazmat.2021.127961.
- Rummel CD, Jahnke A, Gorokhova E, Kühnel D, Schmitt-Jansen M. 2017. Impacts of biofilm formation on the fate and potential effects of microplastic in the aquatic environment. Environmental Science and Technology Letters 4(7):258–267. DOI:10.1021/acs.estlett.7b00164.
- Saba GK, Burd AB, Dunne JP, Hernández‐León S, Martin AH, Rose KA, Salisbury J, Steinberg DK, Trueman CN, Wilson RW, Wilson SE, Wilson SE. 2021. Toward a better understanding of fish‐based contribution to ocean carbon flux. Limnology and Oceanography 66(5):1639–1664. DOI:10.1002/lno.11709.
- Schrank I, Trotter B, Dummert J, Scholz-Böttcher BM, Löder MG, Laforsch C. 2019. Effects of microplastic particles and leaching additive on the life history and morphology of Daphnia magna. Environmental Pollution 255:113233. DOI: 10.1016/j.envpol.2019.113233.
- Song J, Na J, An D, Jung J. 2021. Role of benzophenone-3 additive in chronic toxicity of polyethylene microplastic fragments to Daphnia magna. Science of the Total Environment 800:149638. DOI: 10.1016/j.scitotenv.2021.149638.
- Stanton T, Johnson M, Nathanail P, MacNaughtan W, Gomes RL. 2020. Freshwater microplastic concentrations vary through both space and time. Environmental Pollution 263:114481. DOI: 10.1016/j.envpol.2020.114481.
- Stienbarger CD, Joseph J, Athey SN, Monteleone B, Andrady AL, Watanabe WO, Seaton P, Taylor AR, Brander SM. 2021. Direct ingestion, trophic transfer, and physiological effects of microplastics in the early life stages of Centropristis striata, a commercially and recreationally valuable fishery species. Environmental Pollution 285:117653. DOI: 10.1016/j.envpol.2021.117653.
- Tanaka K, Takada H. 2016. Microplastic fragments and microbeads in digestive tracts of planktivorous fish from urban coastal waters. Scientific Reports 6(1):1–8. DOI:10.1038/srep34351.
- Thompson RC, Moore CJ, Vom Saal FS, Swan SH. 2009. Plastics, the environment and human health: Current consensus and future trends. Philosophical Transactions of the Royal Society B: Biological Sciences 364(1526):2153–2166. DOI:10.1098/rstb.2009.0053.
- Uurasjärvi E, Pääkkönen M, Setälä O, Koistinen A, Lehtiniemi M. 2021. Microplastics accumulate to thin layers in the stratified Baltic Sea. Environmental Pollution 268:115700. DOI: 10.1016/j.envpol.2020.115700.
- Valente T, Pelamatti T, Avio CG, Camedda A, Costantini ML, de Lucia GA, Jacomini C, Piermarini R, Regoli F, Sbrana A, Ventura D, Silvestri C, Matiddi M. 2022. One is not enough: Monitoring microplastic ingestion by fish needs a multispecies approach. Marine Pollution Bulletin 184:114133. DOI: 10.1016/j.marpolbul.2022.114133.
- Van Colen C, Moereels L, Vanhove B, Vrielinck H, Moens T. 2021. The biological plastic pump: Evidence from a local case study using blue mussel and infaunal benthic communities. Environmental Pollution 274:115825. DOI: 10.1016/j.envpol.2020.115825.
- Vargas-Sánchez M, Alcocer J, Oseguera LA. 2022. Seston and eutrophication on a tropical karst lake district: Lagunas de Montebello, Chiapas, Mexico. Limnetica 41(2):269–279. DOI:10.23818/limn.41.16.
- Villéger S, Brosse S, Mouchet M, Mouillot D, Vanni MJ. 2017. Functional ecology of fish: Current approaches and future challenges. Aquatic Sciences 79(4):783–801. DOI:10.1007/s00027-017-0546-z.
- von Moos N, Burkhardt-Holm P, Kohler A. 2012. Uptake and effects of microplastics on cells and tissue of the blue mussel Mytilus edulis L. after an experimental exposure. Environmental Science and Technology 46:11327–11335. DOI: 10.1021/es302332w.
- Woodall LC, Sanchez-Vidal A, Canals M, Paterson GL, Coppock R, Sleight V, Calafat A, Rogers AD, Narayanaswamy BE, Thompson RC. 2014. The deep sea is a major sink for microplastic debris. Royal Society Open Science 1(4):140317. DOI:10.1098/rsos.140317.
- Zebrowski ML, Babkiewicz E, Błażejewska A, Pukos S, Wawrzeńczak J, Wilczynski W, Zebrowski J, Ślusarczyk M, Maszczyk P. 2022. The effect of microplastics on the interspecific competition of Daphnia. Environmental Pollution 313:120121. DOI: 10.1016/j.envpol.2022.120121.
- Zettler ER, Mincer TJ, Amaral-Zettler LA. 2013. Life in the “plastisphere”: Microbial communities on plastic marine debris. Environmental Science and Technology 47(13):7137–7146. DOI:10.1021/es401288x.
- Zhang H. 2017. Transport of microplastics in coastal seas. Estuarine, Coastal and Shelf Science 199:74–86. DOI: 10.1016/j.ecss.2017.09.032.
- Zhao HJ, Xu JK, Yan ZH, Ren HQ, Zhang Y. 2020. Microplastics enhance the developmental toxicity of synthetic phenolic antioxidants by disturbing the thyroid function and metabolism in developing zebrafish. Environment International 140:105750. DOI: 10.1016/j.envint.2020.105750.