Abstract
G. mellonella is a promising species for use in the biodegradation of plastics. It is easy to breed and has high resistance to diverse climatic conditions, which is particularly valuable when considering its potential application in the decomposition of plastics. Thus, it demonstrated the capacity for biodegradation of the most common types of plastics, such as polyethylene (PE) and polypropylene (PP). However, reports on whether consumed plastics or their decomposition products will adversely affect the structure and functioning of the internal organs are rather poor. The studies aimed to determine whether the consumption of PP by a greater wax moth larvae caused any ultrastructural changes in the organs of the animal’s body, evaluate the survival rate of the animals, and describe their reproduction. Thus, this study provided an understanding of histological and ultrastructural changes caused, or not caused, by the PP diet. We investigated three organs – midgut, silk gland, and fat body – under PP consumption by G. mellonella caterpillars (7th instar larvae). The level of reactive oxygen species (ROS) in selected organs, as well as the ability of larvae to survive and undergo metamorphosis were also examined. The animals were divided into four groups: G0-C, G0-S, G0-24, and G0-48. The research used transmission electron microscopy (TEM), confocal microscopy, and flow cytometry. Our study showed that a diet containing PP did not affect internal organs at the ultrastructural level. Cells in the analyzed organs – midgut, silk gland, and fat body – showed no degenerative changes. An increase in the intensity of autophagy and cell vacuolization was noted, but they probably act as a survival pathway. These observations suggest that the final larval stage of the greater wax moth can potentially be applied in PP biodegradation.
1. Introduction
Numerous xenobiotics in the environment or the consumed food affect the functioning of organisms or cause degenerative changes in their various organs. These changes can often lead to the organism’s death (Wilczek et al. Citation2014; Zaffagnini & Martens Citation2016; Rost-Roszkowska et al. Citation2018, Citation2020a, Citation2020b, Citation2021, Citation2022; Augustyniak et al. Citation2020; Lipovšek et al. Citation2022; Ostróżka et al. Citation2022). Plastics consumed by animals will cause numerous lethal effects, so cells in their tissues and organs will be damaged (Anguissola et al. Citation2014; Rodriguez-Seijo et al. Citation2017; Chae et al. Citation2018; Pitt et al. Citation2018; Jâms Citation2020). Plastics mainly consist of synthetic organic polymers, i.e., polyethylene, polypropylene (PP), polyvinyl chloride (PVC), poly(ethylene terephthalate) (PET), or polystyrene (PS). Numerous additives, such as dyes, stabilizers, and fillers, must also be added to the finished product. Most plastics are non-biodegradable or decompose very slowly. Biodegradation occurs as a result of simultaneously operating factors related to the features of the natural environment, the presence of microorganisms, as well as properties of the polymers. Plastic can be degraded into microparticles (<5000 nm in diameter) and further into smaller fragments of nanoparticles (<100 nm in diameter) (Kik et al. Citation2020). They can accumulate in the soil, air, fresh waters, and marine environment, where they will affect organisms (Barnes et al. Citation2009; Thompson et al. Citation2009; Andrady Citation2011). Nanoplastics can even be transferred through the trophic chain (Andrady Citation2011; Mattsson et al. Citation2015; Sharma & Chatterjee Citation2017). Numerous in vivo and in vitro studies suggest that nanoparticles enter the body mainly via the alimentary and respiratory systems (Karpeta-Kaczmarek et al. Citation2016; Cox et al. Citation2019). Plastics, micro-, and nanoplastics cause significant mortality in animals, both invertebrates and vertebrates (Lee et al. Citation2013; Rodriguez-Seijo et al. Citation2017; Sharma & Chatterjee Citation2017). They damage numerous organs, tissues, cells, cell organelles, and cell membranes (Anguissola et al. Citation2014; Chae et al. Citation2018; Pitt et al. Citation2018) due to activating oxidative stress. Reactive oxygen species (ROS) are produced, causing a decrease in the viability of cells and damage to cell organelles (Azad & Iyer Citation2014; Schirinzi et al. Citation2017).
PP is the second most common plastic material after polyethylene (PE). However, it differs from polyethylene in its ability to decompose (Jeon et al. Citation2021). Many studies indicate the possibility of using bacteria or fungi in the biodegradation of polyethylene (Yamada-Onodera et al. Citation2001; Bonhomme et al. Citation2003; Yang et al. Citation2014; Park & Kim Citation2019; Jeon et al. Citation2021), or polystyrene (Mor & Sivan Citation2008; Lou et al. Citation2020; Jeon et al. Citation2021). Meanwhile, little is known about the use of microorganisms in the biodegradation of PP (Auta et al. Citation2018; Skariyachan et al. Citation2018; Jeon et al. Citation2021). As it turns out, there are also insects whose digestive system contains a specific microbiome that facilitates the digestion of consumed plastics: the lesser grain borer Rhyzopertha dominica and tobacco beetle Lasioderma serricorne (Coleoptera) (Riudavets et al. Citation2007), rice mealworm (Corcyra cephalonica) (Lepidoptera), Indian meal moth (Plodia interpunctella) (Lepidoptera) (Bilal et al. Citation2021), the lesser wax moth (Achroia grisella) (Lepidoptera), superworm Zophobas atratus (Coleoptera) (Luo et al. Citation2021), yellow mealworm (Tenebrio molitor) (Coleoptera) (Brandon et al. Citation2018; Yang et al. Citation2018, Citation2021; Ghatge et al. Citation2020; Peng et al. Citation2020), confused flour beetle (T. confusum), red flour beetle (T. castaneum) (Bilal et al. Citation2021) and greater wax moth larvae Galleria mellonella (Lepidoptera) (Bombelli et al. Citation2017; Lou et al. Citation2020).
G. mellonella are insects characterized by fast growth, high fertility, and a relatively short life cycle. The structure and ultrastructure of different organs in this species were precisely described (Uwo et al. Citation2002; Büyükgüzel et al. Citation2013; Poyraz Tinartas et al. Citation2021). Thus, it is treated as a good model organism for histological, toxicological, biomedical, and microbiological studies before in vivo tests are available (Jorjão et al. Citation2018; Mikulak et al. Citation2018; Wojda et al. Citation2020). The caterpillars of the greater wax moth are well-known as a pest of honeycombs (Morse Citation1978). Due to the similarity of the chemical bonds present in the honeycomb and in polyethylene, the larvae of this species can consume products made of it (Bombelli et al. Citation2017; Wojda et al. Citation2020). In situ and in vitro studies revealed that G. mellonella enzymes and intestinal microbiota take part in polyethylene digestion (Bombelli et al. Citation2017; Khyade Citation2018; Kong et al. Citation2019; Lou et al. Citation2020; Bilal et al. Citation2021; Cassone et al. Citation2022). However, there are also reports that larvae of e.g. G. mellonella devoid of intestinal microflora successfully break down long-chain fatty acids found in waxes (Kong et al. Citation2019). It has been suggested that any metabolic mechanisms help caterpillars to obtain energy from polyethylene as a source of food (LeMoine et al. Citation2020). The participation of their salivary glands in polyethylene degradation was also reported (Peydaei et al. Citation2020). The microbial community of the digestive system of G. mellonella also participates in the degradation of PP (Peydaei et al. Citation2021). Some studies presented changes that appeared after the consumption of plastics by G. mellonella larvae in cells of their various organs, including the digestive system or the fat body (LeMoine et al. Citation2020; Cassone et al. Citation2022). However, it is still not known which organelles are targets of plastic degradation and if any survival mechanisms are activated to protect cells/tissues. Transmission electron microscopy enables to provide information if PP particles enter the cytoplasm of cells and are transferred to other organs as well as information regarding their electron density, size, or location (Dreaden et al. Citation2015; Behzadi et al. Citation2017). Thus, our research aimed to determine whether PP particles can be transferred into different organs from the midgut epithelium if the consumption of PP by greater wax moth (G. mellonella) larvae causes any changes at the ultrastructural level in the organs of the animal’s body and to evaluate the survival rate of the animals and describe their reproduction. Thus, this study provided an understanding of histological and ultrastructural changes caused, or not caused, by the PP diet.
2. Material and methods
2.1. Galleria mellonella breeding
Adult specimens of G. mellonella (Lepidoptera, Pyralidae), which were cultured in glass containers with a capacity of 1 liter at 30 ± 0.5°C, with a humidity of 75 ± 5% and constant darkness. Adult males and females do not eat food because they have their mouthparts backward. The glass containers contained linen material on which the females laid their eggs. After laying the eggs, adult individuals die. Eggs were placed in 1 L glass containers with a nutrient solution containing honey, powdered milk, wheat flour, bran, and glycerin (Sehnal Citation1966) because the larvae hatching from the eggs are small (about 1 mm long) and start to feed. Caterpillars of the 7th instar larvae, about 20 mm long (Fasasi & Malaka Citation2006), were selected for the study. The larvae were still feeding, and their size enabled us to collect them precisely for testing.
2.2. Analysis of survival
To test reproduction and survival, one hundred specimens of the last larval stage were collected and put into the 1 L containers (10 containers, 10 specimens per container). They were fed with PP bags (Sarantis Polska S.A.) ad libitum (without nutrient solution) for 4–5 days until pupation (generation G0-P). The number of adult specimens (generation G1-P) that emerged from pupae in each of 10 containers was counted (n = 10). As a control, 100 larvae were harvested and fed ad libitum with a standard nutrient solution (10 containers, 10 specimens per 1 container) (generation G0-C). Then, after pupation, the number of adults hatched from the pupa was counted (n = 10) (generation G1-C).
2.3. Experiment
For the evaluation of changes in the organs of the larvae fed with PP bags, individuals from the last larval stage (generation G0) were examined after 24 h and 48 h of the experiment. The last larval stage (L7) of G. mellonella lasts for about 7–8 days. However, before the pupation, the shrunk larva becomes inactive, entering the prepupa developmental stage (Fasasi & Malaka Citation2006; Desai et al. Citation2019; Wojda et al. Citation2020; Wrońska et al. Citation2022). It occurs during the last 1–2 days of the L7 stage. Larvae collected on the third day of the L7 stage were used for the experiment, then they were starved for 24 hours before the experiment to clean the digestive system of food debris and feces and to prevent any effect of previously eaten food. Thus, the experiment was scheduled for 24 (5th day of the L7 stage) and 48 h (6th day of the L7 stage). From the 7th day of the L7 stage, the control larvae gradually enter the prepupa stage. Time of the experiment cannot be longer (here, there was 24 h of fasting +24 h or 48 h of feeding with PP bags), as the changes appearing in the organs could be caused by the natural processes taking place in the development pattern, i.e., entering the pupal stage. The specimens were divided into the following experimental groups () according to experiments conducted on this species (Lou et al. Citation2020): G0-C – the control group, the animals reared in laboratory conditions in glass containers and fed ad libitum with the medium described by Sehnal (Citation1966) (see: 2.1. Material); G0-S – the animals starved for 48 h G0-24 – the animals cultured on Petri dishes with pieces of PP bags (3 cm × 3 cm) for 24 h; G0-48 – the animals cultured on Petri dishes with PP bags (3 cm × 3 cm) for 48 h. Fasting animals (group G0-S) allowed checking whether the observed changes were caused by the lack of standard food, which was not provided to the animals during the PP diet. The PP bags that were bought commercially (Sarantis Polska S.A.) were weighed before the experiment and also after 24 hours and 48 hours.
Before the section was performed, the animals were anesthetized with chloroform. The following organs were isolated from animals: the midgut, the fat body, and the silk gland, according to .
Table I. Numbers of specimens of approximately caterpillars of the 7th larval stage of G. mellonella prepared for histological and immunohistological methods.
2.4. Methods
2.4.1. Light and transmission electron microscopy
Organs isolated from larvae of all experimental groups were fixed, washed, dehydrated, and embedded in epoxy resin (Epoxy Embedding Medium Kit; Sigma) according to the standard protocol described in our previous papers (Rost-Roszkowska et al. Citation2020b, Citation2022). After cutting the semi-thin sections (0.8 µm), they were stained with 1% methylene blue in 0.5% borax and examined using an Olympus B×60light microscope. Ultrathin sections (70 nm) after contrasting according to the standard method (Rost-Roszkowska et al. Citation2020b; Poprawa et al. Citation2022) were analyzed using the Hitachi H500 transmission electron microscope.
2.4.2. Qualitative analysis – confocal microscopy
2.4.2.1. Dihydroethidium (DHE) – ROS-positive cell staining
Confocal microscopy was used in the qualitative analysis of changes and localization of ROS-positive cells in organs (midgut, fat body, silk gland) isolated from G. mellonella larvae from groups G0-C, G0-S, G0-24, and G0-48. The isolated organs were subjected to the procedure without fixation (vital analysis). Preparation of the material for analysis was performed according to the procedures described in our previous articles (Rost-Roszkowska et al. Citation2020a, Citation2020b). Dihydroethidium (hydroethidine, 5-ethyl-5,6-dihydro-6-phenyl-3,8-diaminophenanthridine) is a dye commonly used to differentiate ROS+ and ROS- cells. It is oxidized by superoxide and is conversed to ethidium/2-hydroxyethidum. The material was incubated with 30 µM DHE (Invitrogen) and DAPI staining (1 µg/ml, 30 min, RT, in the dark) and eventually analyzed with an Olympus FluoView FV1000 confocal microscope diode and argon lasers: 405 nm laser (detection of DAPI) and 559 nm (detection of TMR) (Rost-Roszkowska et al. Citation2022).
2.4.2.2. LysoTracker Red (LTR) staining – labeling acidic organelles
A red fluorescent dye that selectively accumulates in acidic organelles such as lysosomes and autolysosomes was used in the qualitative analysis. Isolated without fixation, organs (midgut, silk gland, and fat body) were incubated with LysoTracker Red (1 µg/ml, 30 min, RT, in the dark) followed by DAPI staining (1 µg/ml, 30 min, RT, in the dark) according to the protocol described by Rost-Roszkowska et al. (Citation2020b) and examined using an Olympus FluoView FV1000 confocal microscope and argon lasers: 405 nm laser (detection of DAPI) and 559 nm (detection of TMR).
2.4.2.3. BODIPY lipid probes
BODIPY (4,4-difluoro-3a,4a-diaza-s-indacene) fluorophore incorporates natural lipids. Organs isolated from animals of all experimental groups without fixation were stained with 20 µl/ml BODIPY working solution (30 min, RT, in the dark) prepared from BODIPY stock according to producer protocol (Molecular probes). After washing with TBS, the material was stained with DAPI solution (1 µg/ml, 30 min, RT, in the dark). Organs were examined using an Olympus FluoView FV1000 confocal microscope and argon lasers: 405 nm laser (detection of DAPI) and 559 nm
2.4.3. Quantitative analysis – flow cytometry
The dissected organs (midgut, fat body, silk gland) isolated from each experimental group were mechanically fragmented and homogenized to obtain the cell suspension according to the procedure presented in our previous paper (Rost-Roszkowska et al. Citation2020b; Poprawa et al. Citation2022).
2.4.3.1. Muse oxidative stress kit
Muse Oxidative Stress Kit (Merck Millipore, № MCH100111) enables quantitative analysis of the differentiated population of ROS- and ROS+ cells. The procedure was conducted according to the manufacturer’s protocol, and the measurements were performed using the Muse Cell Analyzer (Millipore) (Włodarczyk et al. Citation2019).
2.4.3.2. Muse count & viability kit
Muse Count & Viability Kit (Merck Millipore, No MCH100102) stains viable (live cells) and non-viable cells (dead or dying cells). It enables the quantitative analysis of cell count and viability using the Muse Cell Analyzer. The procedure was conducted according to the manufacturer’s protocol, and the measurements and data were generated with the Muse Count & Viability Software Module.
Statistical analysis
Statistical analysis of the data was performed with STATISTICA 13 (software package system, version 13.0, http://www.statsoft.com). The significance of differences in the levels of analyzed parameters was assessed using the LSD test. The correlation of parametric values was based on Pearson’s regression analysis using the multiple regression model. Results were considered significant at p < 0.05.
3. Results
3.1. PP bag measurements
After 24 and 48 hours of the experiment, the PP bags showed signs of consumption by the caterpillars ()). Additionally, PP bags were weighed before and after the experiment. The weight of PP bags after 48 h of consumption significantly decreased to 9 mg compared start point, while after 24 hours of consumption weight of PP bags was 6 mg lower than at the beginning of the experiment ().
Figure 2. (a) A fragment of the polypropylene bag before the experiment. (b) A fragment of the propylene bag after 48 h of the experiment. (c) Weight of polypropylene bags [mg] (mean ±SD) in analyzed experiment time points: 0 – start point, after 24 h and 48 h of polypropylene bags consumption. Different letters (a, b) indicate significant differences among groups within organs (Tukey test, p < 0.05; n = 5).
![Figure 2. (a) A fragment of the polypropylene bag before the experiment. (b) A fragment of the propylene bag after 48 h of the experiment. (c) Weight of polypropylene bags [mg] (mean ±SD) in analyzed experiment time points: 0 – start point, after 24 h and 48 h of polypropylene bags consumption. Different letters (a, b) indicate significant differences among groups within organs (Tukey test, p < 0.05; n = 5).](/cms/asset/f6c4462f-b39b-457b-871e-0f356ec46129/tizo_a_2308529_f0002_oc.jpg)
3.2. Analysis of survival of G. mellonella larvae fed with PP
Counting adult specimens (G1-C, G1-P experimental groups) that developed from larvae fed with PP bags (G0-P) and standard nutrients (G0-C) showed that the percentage of surviving individuals in both experimental groups was high and comparable ().
3.3. Analysis of ROS+/ROS- cells in organs of G. mellonella larvae fed with PP
Confocal microscopy showed a slight increase in the strength of signals emitted by positive ROS cells in the midgut after 24 and 48 hours of PP consumption ()). However, in the silk gland and fat body, the strength of the signals emitted by ROS+ cells slightly decreases with increasing consumption of PP ()). No changes were observed between G0-C and G0-S animals.
Figure 4. 3D representation of the DHE and DAPI staining of organs in G. mellonella larvae. (a-l) Confocal microscope. ROS-positive cells (red), nuclei (n, blue). (a-c) G0-C experimental group. (d-f) G0-S experimental group. (g-i) G0-24 experimental group. (j-l) G0-48 experimental group. (a, j) Scale bar = 11 μm. (b, e) Scale bar = 26 μm. (c, f, i, l) Scale bar = 16 μm. (d, g) Scale bar = 15 μm. (h, k) Scale bar = 28 μm. (m) Flow cytometry. Percentage of ROS positive cells (ROS+) (mean ±SD) in the midguts, silk glands, and fat bodies of. G. mellonella. Different letters (a, b) indicate significant differences among groups within organs (LSD, p < 0.05; n = 5).
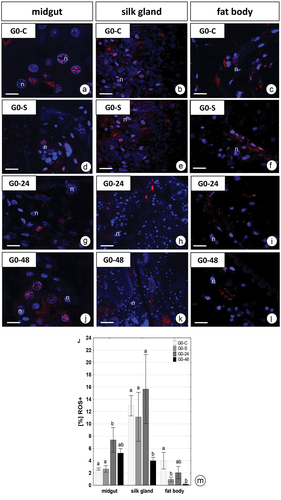
The quantitative analysis using dihydroethidium (DHE) for G. mellonella larvae revealed a diverse distribution of ROS in all experimental groups depending on the period of exposure to PP and type of organ. The quantitative analysis showed 2.6% ± 0.19, 13.0% ± 1.65, and 4.0% ± 1.35 ROS positive cells (ROS+) in the midgut, silk glands, and fat body of control individuals, respectively (). After 24 h fed of PP, the percentage of ROS+ cells in the midgut strongly increased, nearly 3-fold compared to the control group (p = 0.03), but after 48 h fed on this xenobiotic, the percentage of ROS+ cells in this organ was only 2-fold higher than in the control (p = 0.61) (). The opposite relationships were observed for the other organs. After 48 h of PP feeding the number of ROS+ cells in the silk gland was 4-fold lower (p = 0.001), while in the fat body nearly 20-fold lower (p = 0.01) than in the complementary control group. Generally, the signals from the fat body cells were the weakest, compared with signals from the silk gland and midgut cells after PP exposure. The starvation (G0-S) did not cause a significant change in the number of viable cells in the midguts and silk glands compared with the control group while the percentages of viable cells in the fat body of these specimens were significantly lower than in control animals (p = 0.03) but similar to G0-24 and G0-48 groups ().
3.4. Ultrastructure of cells in organs of G. mellonella larvae fed with PP
3.4.1. Midgut epithelium
In the last instar of G. mellonella, the pseudostratified midgut epithelium is formed by digestive cells, goblet cells, regenerative cells (), and endocrine cells. In individuals of the G0-C control group, the apical cytoplasm had numerous mitochondria, cisterns of rough endoplasmic reticulum, and single autophagic structures ()). Two types of secretion were observed: merocrine (), and apocrine (). The perinuclear cytoplasm was rich in mitochondria, cisterns of rough endoplasmic reticulum (RER) and glycogen granules, while only some cisterns of the smooth endoplasmic reticulum (SER) were present (). The basal cytoplasm was represented by a well-developed membranous labyrinth, numerous mitochondria, cisterns of RER, and glycogen granules (). The main feature of goblet cells was the electron-lucent cavity with distinct cytoplasmic projections possessing mitochondria. The oval nucleus was surrounded by numerous mitochondria, cisterns of RER, and sporadic autophagic structures ()). The cytoplasm of regenerative cells that form regenerative nidi was poor in organelles ()). Endocrine cells were recognized by numerous droplets with storage material (not shown). In fasted individuals (G0-S group), midgut epithelial cells showed the same ultrastructure as in the control specimens (G0-C group) ()).
Figure 5. The midgut epithelium of G. mellonella 7th instar larva. (a-g) Control animals (G0-C). TEM. (a) Fragment of the midgut epithelium with digestive cells (dc), goblet cell (gc), and regenerative cell (rc). Mitochondria (m), nuclei (n), goblet cell cavity (star), cytoplasmic projections of the goblet cell (arrows), basal lamina (bl), visceral muscles (vm), autophagic structures (au). Scale bar = 2.9 μm. (b-c) the apical cytoplasm of digestive cells. Microvilli (mv), mitochondria (m), cisterns of RER (RER), nucleus (n), midgut lumen (l), autophagic structures (au), apocrine secretion (star), exocrine secretion (arrows). (b) Scale bar = 1.7 μm. (c) Scale bar = 1.8 μm. (d) Perinuclear cytoplasm in digestive cells. Mitochondria (m), cisterns of RER (RER) and SER (SER), nuclei (n), and glycogen granules (g). Scale bar = 1.4 μm. (e) Basal cytoplasm in the digestive cell with the distinct membranous labyrinth (arrows) surrounded by mitochondria (m), glycogen granules (g), and cisterns of RER (RER). Basal lamina (bl). Scale bar = 0.6 μm. (f) the goblet cell (gc) with the cavity (star) and cytoplasmic projections (arrows) filled with mitochondria (m). Cisterns of RER (RER), autophagic structures (au), nucleus (n), digestive cells (dc). Scale bar = 0.9 μm. (g) a fragment of the regenerative nidi with regenerative cells (rc). Nucleus (n), mitochondria (m), digestive cells (dc). Scale bar = 1.5 μm. (h-i) Fasted animals (G0-S). (h) Fragment of apical regions in digestive cells. Mitochondria (m), cisterns of RER (RER), midgut lumen (l), autophagic structures (au). Scale bar = 0.7 μm. (i) a fragment of goblet cell (gc) with the cavity (star) and cytoplasmic projections (arrows) filled with mitochondria (m). Digestive cell (dc), nucleus (n), cisterns of RER (RER). Scale bar = 1 μm.
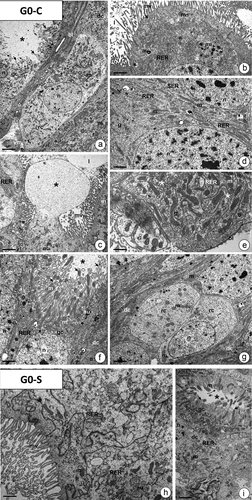
In animals from experimental group G0-24, no changes were observed in the cytoplasm of regenerative cells, goblet cells, and endocrine cells. An increase in the number of autophagic structures was observed in the apical cytoplasm of digestive cells, while no other alterations were detected in their cytoplasm ()). In the cytoplasm of digestive cells in the midgut epithelium of animals from the G0-48 experimental group, numerous vacuoles with electron-lucent content appear, and the autophagy is more intense than in the G0-24 experimental group. Degeneration of the remaining organelles in all cytoplasmic regions was not observed ()). Vacuoles and autophagic structures (autophagosomes, autolysosomes, residual bodies) were also detected in the cytoplasm of the goblet () and regenerative cells (). No alterations appeared in the cytoplasm of endocrine cells (not shown). Confocal microscopy confirmed the increase in autophagy intensity. Along with the longer consumption of PP, the strength of signals emitted by strongly acidic structures increased ()).
Figure 6. The midgut epithelium of G. mellonella 7th instar larva in experimental groups G0-24 (a-c) and G0-48 (d-g). TEM. Digestive cells (dc), goblet cells (gc), mitochondria (m), microvilli (mv), cytoplasmic projections of goblet cells (arrows), cisterns of RER (RER), autophagic structures (au), goblet cell cavity (stars), apocrine secretion arrowhead), midgut lumen (l). (a) Scale bar = 2.3 μm. (b) Scale bar = 1.9 μm. (c) Scale bar = 2.9 μm. (d) Scale bar = 1.1 μm. (e) Scale bar = 1.5 μm. (f) Scale bar = 1.3 μm. (g) Scale bar = 1.7 μm.
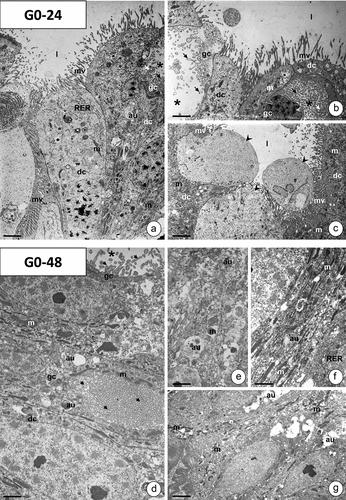
3.4.2. Silk gland
While the entire silk gland was used for quantitative analysis, the middle region (MSG) of this organ was used for TEM analysis. Cells of MSG in larvae from the G0-C group possessed a cytoplasm rich in cisterns of RER as well as mitochondria. The majority of mitochondria were distended with a small amount of mitochondrial crista and the electron-lucent matrix. The lobular-shaped nucleus was located in the central part of the cell. Some spheres of storage material and sporadic autophagic structures were present (). The apical cell membrane formed numerous cytoplasmic projections protruding into the gland lumen ()). The ultrastructure of the cells in MSG in specimens from the G0-S group did not show any differences compared to the controls ()). After 24 h of feeding (G0-24 experimental group) with PP, apart from the numerous autophagosomes observed, no changes at the ultrastructural level were detected ()), while in G0-48 experimental group sporadic vacuoles with electron-lucent content and numerous autophagosomes appeared ()). A confocal microscope revealed an increase in the signals emitted by acid structures in the G0-24 experimental group, while they decreased after 48 h of feeding on PP ()).
Figure 7. Middle region of silk gland (MSG) in G. mellonella 7th instar larva in experimental groups G0-C (a-b), G0-S (c-d), G0-24 (e-f), and G0-48 (g-h). TEM. Mitochondria (m), nuclei (n), cisterns of RER (RER), autophagic structures (au), storage material (sm), cytoplasmic projections (arrows), gland lumen (gl). (a) Scale bar = 3.4 μm. (b) Scale bar = 1.3 μm. (c) Scale bar = 1 μm. (d) Scale bar = 0.8 μm. (e) Scale bar = 0.9 μm. (f) Scale bar = 1.1 μm. (g) Scale bar = 1 μm. (h) Scale bar = 1.2 μm.
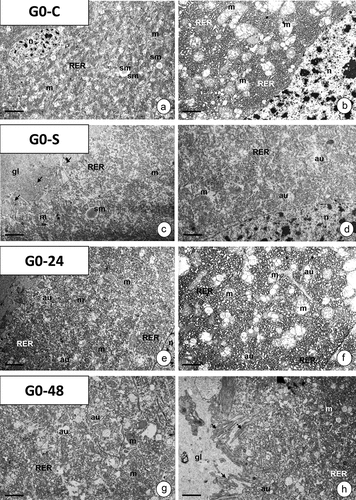
3.4.3. Fat body
The entire cytoplasm of trophocytes which form the fat body in the last instar larva of G. mellonella (G0-C) contains large spheres of storage material () with different electron densities. The lobular nucleus contained small amounts of heterochromatin distributed evenly throughout the nucleoplasm. In the vicinity of the nucleus, mitochondria and cisterns of RER and individual autophagic structures could be observed ()). In starved animals (G0-S experimental group) numerous spheres with the heterogenous material were observed ()). Feeding animals with PP bags (G0-24 and G0-48 experimental groups) did not cause any degenerative changes in organelles of trophocytes, but an increase in the number of autophagic structures was detected ()). A confocal microscope revealed a slight increase in the level of signals emitted by acid structures in the G0-S animals, while it was distinct in trophocytes of G0-24 and G0-48 experimental groups ()).
Figure 8. Fat body of G. mellonella 7th instar larva in experimental groups G0-C (a-b), G0-S (c-d), G0-24 (e-f) and G0-48 (g-h). TEM. Mitochondria (m), nuclei (n), cisterns of RER (RER), autophagic structures (au), storage material (sm). (a) Scale bar = 1.3 μm. (b) Scale bar = 3.4 μm. (c) Scale bar = 1.3 μm. (d) Scale bar = 1 μm. (e) Scale bar = 2.2 μm. (f) Scale bar = 1.4 μm. (g) Scale bar = 2.4 μm. (h) Scale bar = 1.2 μm.
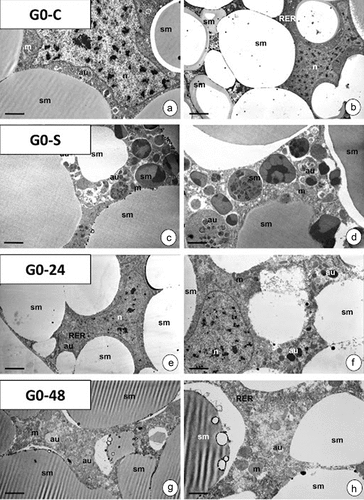
Figure 9. Autophagy in organs of 7th G. mellonella larvae: midgut, silk gland, and fat body. 3D representation of the accumulation of lysosomes and autolysosomes (red signals). Nuclei (blue signals). LysoTracker Red and DAPI staining. Confocal microscope. (a-c) G0-C group. (d-f) G0-S experimental group. (g-i) G0-24 experimental group. (j-l)) G0-48 experimental group. (a, d) Scale bar = 10 μm. (b, e) Scale bar = 14 μm. (c, f) Scale bar = 12 μm. (g, i, j, l) Scale bar = 13 μm. (h, k) Scale bar = 18 μm.
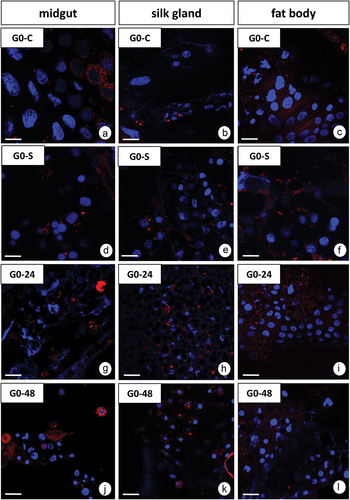
Figure 10. 3D representation of lipid accumulation (green signals, arrows) in organs of G. mellonella larvae: midgut, silk gland and fat body. Nuclei (blue signals). BODIPY and DAPI staining. Confocal microscope. G0-C, G0-S, G0-24 and G0-48 represent experimental groups described in methodology. Scale bar = 20 μm.
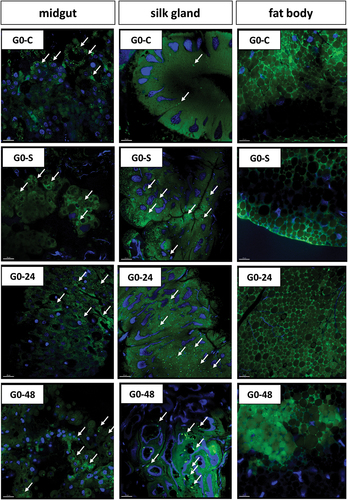
3.5. Cell count and viability in organs of G. mellonella larvae fed with PP
The average percentages of viable cells were high in all analyzed organs of control individuals: midgut (74.5% ± 1.74), silk glands (96.6% ± 0.64), and fat body (94.4% ± 0.59). 48 hours of PP feeding caused a significant decrease in the number of viable cells in the silk gland and fat body compared to the control group (23%, p = 0.0001; 65%, p = 0.0001), respectively, and exposed for 24 hours (8%, p = 0.01; 58%, p = 0.0001, respectively) (). Exposure to PP per 48 h did not cause a significant change in the number of viable cells in the midgut, while the percentage of viable cells in the midgut of individuals after 24-hour exposure to PP was significantly higher than in the control group (at 18%, p = 0.0001) (). The starvation (G0-S) did not cause a significant change in the number of viable cells in the silk glands compared with control group, while the percentages of viable cells in the midguts and fat bodies of individuals were similar to group G0-24 (). There were statistically significant correlations between the level of viability cells and the percentage of ROS+ cells in the midgut and fat body in foraging individuals
4. Discussion
Plastics in the environment decompose into micro- and nanoplastics, and such small particles can easily pass through the cellular barrier, which is constituted by cell membranes. Ultrastructural analysis of different nanoparticles reveals that they interact with the cell surface and could enter cells by endocytosis (Behzadi et al. Citation2017). In the cytoplasm, they resemble electron-dense granules or flocculent-granular patches (Købler et al. Citation2014; Karpeta-Kaczmarek et al. Citation2016). Different cell types may react differently to internalize the same NP (Xia et al. Citation2008; dos Santos et al. Citation2011). Numerous microorganisms in the digestive system of some animals can break down plastics such as PP, PE, and PS (Bonhomme et al. Citation2003; Yang et al. Citation2014; Huerta Lwanga et al. Citation2018; Auta et al. Citation2018; Skariyachan et al. Citation2018; Park & Kim Citation2019; Lou et al. Citation2020; Jeon et al. Citation2021). However, whether these plastics or their decomposition products will induce any changes in the ultrastructure of cells in different organs has not been thoroughly described. In our research, we evaluated, for the first time, the ability of PP-fed larvae to undergo metamorphosis and develop. We found that, regardless of whether G. mellonella larvae were fed with a standard diet or with PP bags, the number of adults that developed was comparable. A decrease in the survival of individuals or a decrease in the number of offspring after feeding on plastic has been described in, e.g., soil (Rodriguez-Seijo et al. Citation2017), marine (Messinetti et al. Citation2018), and freshwater invertebrates (Rehse et al. Citation2016; Schür et al. Citation2019; Huang et al. Citation2022). Larvae of T. molitor are able to survive when they eat polystyrene in comparison to starvation (Yang et al. Citation2018; Matyja et al. Citation2020). In addition, the survival rate of animals fed with polystyrene and supplemented with oatmeal was similar to controls (Matyja et al. Citation2020). It has been reported that adding certain supplements such as proteins or vitamins to the PP diet affected the development and growth of larvae in this species (Morales-Ramos et al. Citation2010; Matyja et al. Citation2020). Collectively, studies on T. molitor demonstrated that changes in the development of polystyrene-fed larvae make it difficult to complete the larval life cycle. This means that T. molitor larvae probably are not suitable for use in polystyrene biodegradation (Matyja et al. Citation2020). However, some research revealed that plastics do not affect the development or survival of invertebrates (Bruck & Ford Citation2018; Pflugmacher et al. Citation2020; Scopetani et al. Citation2020; Weber et al. Citation2018). Studies on G. mellonella showed that a polyethylene and polystyrene diet does not affect the short-term growth of its larvae (LeMoine et al. Citation2020; Lou et al. Citation2020). In this study, caterpillars of the greater wax moth were fed only with the PP diet to view changes that could be caused by plastic. Plastic-fed animals did not receive a standard nutrient solution. Therefore, in order to exclude the influence of lack of food on the described processes, we also examined animals completely deprived of food (G0-S group). Because of the fact that larvae could develop normally, we concluded that feeding G. mellonella with PP will not damage the cells in the larvae’s organs. Thus, we decided to analyze whether any changes appeared in different organs of G. mellonella caterpillars that could help elucidate their survival possibility.
The numerous xenobiotics that are toxic to cells and tissues will cause an increase in the level of reactive oxygen species (ROS). While low levels of ROS are required for the proper functioning of cells and numerous cellular mechanisms, high levels of ROS will activate proliferation, cell differentiation, or even cell death (oxidative stress) or the mechanisms leading to their neutralization (Zhou et al. Citation2014; Oropesa et al. Citation2017; Tarnawska et al. Citation2019; Włodarczyk et al. Citation2019; Rost-Roszkowska et al. Citation2021, Citation2022). The number of ROS-positive cells in the G. mellonella larvae midgut increased after 24 h of the experiment. After 48 hours, a decrease in the number of these cells could be observed compared to the 24-hour experiment, but the level was still higher than in the control specimens. In the silk gland, after 24 h, an increase and then a strong decrease in the level of ROS in the cells of this organ were observed. On the other hand, in the fat body, the number of positive ROS cells decreased regardless of the duration of PP consumption. This proves that the midgut is the most sensitive to this xenobiotic or its decomposition products, protecting other internal organs (Leonard et al. Citation2009; Janeh et al. Citation2019). The decrease in the level of ROS in the cells of the other two organs may be related to the activation of ROS-neutralizing mechanisms. ROS act as a kind of enhancer, the work of which is to intensify unfavorable changes in cells to provide a faster response from the whole organism. The best example is the activation of several enzyme proteins involved in maintaining cellular energy homeostasis. Thanks to this, it can slow down anabolic processes, while accelerating the secretion of energy in catabolic transformations (Li & Marbán Citation2010). Changes in the ROS level may indicate changes taking place in cellular organelles, especially mitochondria, nuclei, lysosomes, or Golgi complexes (Sokolova Citation2004; Auten & Davis Citation2009; Hödl et al. Citation2010; Repnik & Turk Citation2010; Jiang et al. Citation2011; Faron et al. Citation2015; Zorova et al. Citation2018; Rost-Roszkowska et al. Citation2021). In addition, different organs and their cellular organelles in the animal body may react differently to the same stressor (Rost-Roszkowska et al. Citation2020a, Citation2020b, Citation2022; Dziewięcka et al. Citation2020; Poprawa et al. Citation2022). Thus, describing changes in the ultrastructure of different organs in G. mellonella larvae fed with PP should explain whether the increase or decrease of ROS-positive cells is connected with degenerative/regenerative processes. The decrease in the ROS level after 48 hours of the experiment may also be associated with intensively occurring autophagy. In all three analyzed organs – midgut, silk gland, and fat body – no degenerative hanges in cellular organelles were observed with the prolonged consumption of PP. Only an increase in the intensity of autophagy was observed, especially after 48 hours of PP consumption (midgut and fat body), or 24 hours of the experiment (silk gland). Induction of autophagy or its dysfunction is considered to be the effect of nanoplastics (NPs) on cellular functioning (Ma et al. Citation2011; Wang et al. Citation2017). As a result of autophagy, numerous toxic substances or damaged cell structures and organelles are degraded. After their enclosure in autophagosomes, and then the formation of autolysosomes, they are digested, and the processes of cell death (apoptosis, necrosis) are not activated. Thus, in this case, autophagy acts as a survival pathway (Klionsky & Emr Citation2000; Kourtis & Tavernarakis Citation2009; Franzetti et al. Citation2012; Gunay & Goncu Citation2021). Autophagy has been described in the midgut of many invertebrates as a process by which homeostasis is maintained in tissues and cells (Lipovsek & Novak Citation2016; Włodarczyk et al. Citation2017; Lipovšek et al. Citation2018; Rost-Roszkowska et al. Citation2018, Citation2019, Citation2020a, Citation2020b, Citation2022). The analysis of the G0-S group showed that autophagy in the studied species was not activated under the influence of the lack of a standard diet. Thus, we can conclude that in G. mellonella larvae fed with PP, autophagy is intensified, which proves its protective role. Thus, no damaged or transformed organelles have been observed. However, in the analyzed larval organs, we also observed changes in the vacuolization of the cytoplasm. Irreversible vacuolization may be a symptom of necrosis as the cytopathological process begins. However, during transient vacuolization, the formation of vacuoles is caused by the balance of osmotic pressure among different cytoplasmic compartments and by water diffusion across organelle membranes (Aki et al. Citation2012; Shubin et al. Citation2016). It reversibly affects cell physiology and e.g., the cell cycle, proliferation, and cell migration (Morissette et al. Citation2008). While during transient necrosis, only acidic organelles are affected, the irreversible vacuolization may cause changes in the entire endosomal-lysosomal system as well as Golgi complexes (Shubin et al. Citation2016). Vacuolization was evident in the organs of the examined G. mellonella larvae fed with PP. Mainly after 48 h of PP consumption by caterpillars, an increase in the number of vacuoles with an electron-lucent interior (midgut and silk gland) was observed. Vacuoles and autophagic structures (autophagosomes, autolysosomes, residual bodies) also appeared in the cytoplasm of the goblet and regenerative cells of the midgut epithelium, whereas they were not detected (regenerative cells) or were sporadic (goblet cells) in the control animals. However, no degenerative changes in other organelles were observed. Hence, it is very likely that this process does not lead to the typically irreversible necrosis after PP ingestion. In addition, it may be confirmed by the fact of increasing the number of autophagic structures, which are strongly acidic. To confirm this, further qualitative and quantitative studies must analyze what specific type of cell death occurs in the organs of PP-consuming larvae.
The study of cell viability in the organs of G. mellonella larvae showed a decrease in the number of live cells in all analyzed organs after 48 h. However, it may be related to the physiological changes taking place in the caterpillar’s body before its transformation into a pupa (Uwo et al. Citation2002; Tettamanti et al. Citation2007; Denton et al. Citation2012; Franzetti et al. Citation2012; Romanelli et al. Citation2016; Jorjão et al. Citation2018; Bonelli et al. Citation2019; Gunay & Goncu Citation2021). The study used 7th instar larvae of G. mellonella. On the 5th-6th day of this final instar the animals stop feeding before formation of the cocoon and pupation. Before the experiment, the larvae fasted for 24 h. The experiment was conducted for 48 h. After 72 h, we observed that the larvae were preparing to transform and stopped eating. The analysis of the G0-S group confirmed that the fasting of animals had no effect of larval survival. Our research indicates that eating PP does not alter the cells of the larval organs. Despite the initially increasing level of ROS-positive cells, autophagy and cell vacuolization will protect organs against the effects of PP decomposition products. These results provide a positive sign for the future use of G. mellonella larvae in the biodegradation of plastics. However, it is necessary to carry out further research, especially regarding the activation of apoptosis and necrosis, or the triggered mechanisms that turn on enzymes such as dismutases, catalases, and even proteins responsible for cell protective functions. It is also necessary to conduct further research to count individuals in subsequent generations and analyze the structure and ultrastructure of internal organs in larvae of the next generations Negative effects such as reduced growth, fertility, or degenerative changes of organs may lead to long-term population effects. In addition, after contact with the stressor, changes may appear in subsequent generations in different invertebrate species (Schür et al. Citation2019; Augustyniak et al. Citation2020; Babczyńska et al. Citation2020; Dziewięcka et al. Citation2020).
Conclusions
The histological studies on G. mellonella larvae fed with PP bags showed that the diet containing plastics did not affect internal organs at the ultrastructural level. Thus, cells in the three analyzed organs – midgut, silk gland, and fat body – showed no degenerative changes after consumption of PP. Autophagy and vacuolization probably act as survival pathways. However, more advanced studies must be conducted. These processes probably have an impact on the survival rate of the last larvae of the greater wax moth, making them a potentially useful organism in the biodegradation of plastics made of PP.
Acknowledgments
We are very grateful to Michalina Komandera (University of Silesia in Katowice, Poland) for her technical assistance and Richard Ashcroft (http://www.anglopolonia.com/home.html) for language correction.
Disclosure statement
No potential conflict of interest was reported by the author(s).
Additional information
Funding
References
- Aki T, Nara A, Uemura K. 2012. Cytoplasmic vacuolization during exposure to drugs and other substances. Cell Biology and Toxicology 28(3):125–131. DOI: 10.1007/s10565-012-9212-3.
- Andrady AL. 2011. Microplastics in the marine environment. Marine Pollution Bulletin 62(8):1596–1605. DOI: 10.1016/j.marpolbul.2011.05.030.
- Anguissola S, Garry D, Salvati A, O’Brien PJ, Dawson KA, Hussain S. 2014. High content analysis provides mechanistic insights on the pathways of toxicity induced by amine-modified polystyrene nanoparticles. PLoS ONE 9(9):e108025. DOI: 10.1371/journal.pone.0108025.
- Augustyniak M, Tarnawska M, Dziewięcka M, Kafel A, Rost-Roszkowska M, Babczyńska A. 2020. DNA damage in Spodoptera exigua after multigenerational cadmium exposure - a trade-off between genome stability and adaptation. The Science of the Total Environment 745:1–10. DOI: 10.1016/j.scitotenv.2020.141048.
- Auta HS, Emenike CU, Jayanthi B, Fauziah SH. 2018. Growth kinetics and biodeterioration of polypropylene microplastics by Bacillus sp. and Rhodococcus sp. isolated from mangrove sediment Mar. Marine Pollution Bulletin 127:15–21. DOI: 10.1016/j.marpolbul.2017.11.036.
- Auten R, Davis J. 2009. Oxygen toxicity and reactive oxygen species: The devil is in the details. Pediatric Research 66(2):121–127. DOI: 10.1203/PDR.0b013e3181a9eafb.
- Azad N, Iyer AKV. 2014. Reactive oxygen species and apoptosis. In: Laher I, editor. Systems biology of free radicals and antioxidants. Berlin, Heidelberg: Springer. pp. 113–136. DOI: 10.1007/978-3-642-30018-9_15.
- Babczyńska A, Nowak A, Kafel A, Łozowski B, Rost-Roszkowska M, Tarnawska M, Augustyniak M, Sawadro M, Molenda A. 2020. Autophagy: A necessary defense against extreme cadmium intoxication in a multigenerational 2D experiment. Scientific Reports 10(1):21141. DOI: 10.1038/s41598-020-78316-z.
- Barnes DKA, Galgani F, Thompson RC, Barlaz M. 2009. Accumulation and fragmentation of plastic debris in global environments. Philosophical Transactions of the Royal Society B3641985–1998. DOI: 10.1098/rstb.2008.0205.
- Behzadi S, Serpooshan V, Tao W, Hamaly MA, Alkawareek MY, Dreaden EC, Brown D, Alkilany AM, Farokhzad OC, Mahmoudi M. 2017. Cellular uptake of nanoparticles: Journey inside the cell. Chemical Society Reviews 46(14):4218. DOI: 10.1039/c6cs00636a.
- Bilal H, Raza H, Bibi H, Bibi T. 2021. Plastic biodegradation through insects and their symbionts microbes: A review. JBM 8(4):95–103. DOI: 10.35691/JBM.1202.0206.
- Bombelli P, Howe CJ, Bertocchini F. 2017. Polyethylene biodegradation by caterpillars of the wax moth Galleria mellonella. Current Biology: CB 27(8):R292–R293. DOI: 10.1016/j.cub.2017.02.060.
- Bonelli M, Bruno D, Caccia S, Sgambetterra G, Cappellozza S, Jucker C, Tettamanti G, Casartelli M. 2019. Structural and functional characterization of Hermetia illucens larval midgut. Frontiers in Physiology 10:204. DOI: 10.3389/fphys.2019.00204.
- Bonhomme S, Cuer A, Delort A-M, Lemaire J, Sancelme M, Scott C. 2003. Environmental biodegradation of polyethylene. Polymer Degradation & Stability 81(3):441–452. DOI: 10.1016/S0141-3910(03)00129-0.
- Brandon AM, Gao S-H, Tian R, Ning D, Yang S-S, Zhou J, Wu WM, Criddle CS. 2018. Biodegradation of polyethylene and plastic mixtures in mealworms (Larvae of Tenebrio molitor) and effects on the gut microbiome. Environmental Science and Technology 52(11):6526–6533. DOI: 10.1021/acs.est.8b02301.
- Bruck S, Ford AT. 2018. Chronic ingestion of polystyrene microparticles in low doses has no effect on food consumption and growth to the intertidal amphipod Echinogammarus marinus? Environmental Pollution 233:1125–1130. DOI: 10.1016/j.envpol.2017.10.015.
- Büyükgüzel E, Büyükgüzel K, Snela M, Erdem M, Radtke K, Ziemnicki K, Adamski Z. 2013. Effect of boric acid on antioxidant enzyme activity, lipid peroxidation, and ultrastructure of midgut and fat body of Galleria mellonella. Cell Biology and Toxicology 29(2):117–129. DOI: 10.1007/s10565-013-9240-7.
- Cassone BJ, Grove HC, Kurchaba N, Geronimo P, LeMoine CMR. 2022. Fat on plastic: Metabolic consequences of an LDPE diet in the fat body of the greater wax moth larvae (Galleria mellonella). Journal of Hazardous Materials 425:127862. DOI: 10.1016/j.jhazmat.2021.127862.
- Chae Y, Kim D, Kim SW, An Y. 2018. Trophic transfer and individual impact of nano-sized polystyrene in a four-species freshwater food chain. Scientific Reports 8(1):284. DOI: 10.1038/s41598-017-18849-y.
- Cox KD, Covernton GA, Davies HL, Dower JF, Juanes F, Dudas SE. 2019. Human consumption of microplastics. Environmental Science and Technology 53(12):7068–7074. DOI: 10.1021/acs.est.9b01517.
- Denton D, Chang T-K, Nicolson S, Shravage B, Simin R, Baehrecke EH, Kumar S. 2012. Relationship between growth arrest and autophagy in midgut programmed cell death in Drosophila. Cell Death & Differentiation 19(8):1299–1307. DOI: 10.1038/cdd.2012.43.
- Desai AV, Siddhapara MR, Patel PK, Prajapati AP. 2019. Biology of greater wax moth, on artificial diet. Journal of Experimental Zoology, India 22:1267–1272.
- dos Santos T, Varela J, Lynch I, Salvati A, Dawson KA, Schnur JM. 2011. Effects of transport inhibitors on the cellular uptake of carboxylated polystyrene nanoparticles in different cell lines. PLoS ONE 6(9):e24438. DOI: 10.1371/journal.pone.0024438.
- Dreaden EC, Kong YW, Morton SW, Correa S, Choi KY, Shopsowitz KE, Renggli K, Drapkin R, Yaffe MB, Hammond PT. 2015. Tumor-targeted synergistic blockade of MAPK and PI3K from a layer-by-layer nanoparticle. Clinical Cancer Research 21(19):4410–4419. DOI: 10.1158/1078-0432.CCR-15-0013.
- Dziewięcka M, Flasz B, Rost - Roszkowska M, Kędziorski A, Kochanowicz A, Augustyniak M. 2020. Graphene oxide as a new anthropogenic stress factor - multigenerational study at the molecular, cellular, individual and population level of Acheta domesticus. Journal of Hazardous Materials 396:122775. DOI: 10.1016/j.jhazmat.2020.122775.
- Faron J, Bernaś T, Sas-Nowosielska H, Klag J. 2015. Analysis of the behavior of mitochondria in the ovaries of the earthworm Dendrobaena veneta Rosa 1839. PLoS ONE 10(2):e0117187. DOI: 10.1371/journal.pone.0117187.
- Fasasi KA, Malaka SLO. 2006. Life cycle and impact of greater Wax Moth. Gallenia niellonella L (Lepidoptera: Pyralidae), feeding on stored beeswax. Nigerian Journal of Entomology 23(1):13–17. DOI: 10.36108/NJE/6002/32.0130.
- Franzetti E, Huang ZJ, Shi YX, Xie K, Deng XJ, Li JP, Li Q-R, Yang W-Y, Zeng W-N, Casartelli M, Deng H-M, Cappellozza S, Grimaldi A, Xia Q, Tettamanti G, Cao Y, Feng Q. 2012. Autophagy precedes apoptosis during the remodeling of silkworm larval midgut. Apoptosis 17(3):305–324. DOI: 10.1007/s10495-011-0675-0.
- Ghatge S, Yang Y, Ahn JH, Hur H-G. 2020. Biodegradation of polyethylene: A brief review. Applied Biological Chemistry 63(1):27. DOI: 10.1186/s13765-020-00511-3.
- Gunay B, Goncu E. 2021. Role of autophagy in midgut stem cells of silkworm Bombyx mori, during larval–pupal metamorphosis. Archives of Insect Biochemistry and Physiology 108(1):e21832. DOI: 10.1002/arch.21832.
- Hödl E, Felder E, Chabicovsky M, Dallinger R. 2010. Cadmium stress stimulates tissue turnover in Helix pomatia: Increasing cell proliferation from metal tolerance to exhaustion in molluscan midgut gland. Cell and Tissue Research 341:159–171. DOI: 10.1007/s00441-010-0980-x.
- Huang C-H, Chu T-W, Kuo C-H, Hong M-C, Chen Y-Y, Chen B. 2022. Effects of microplastics on reproduction and growth of freshwater live feeds Daphnia magna. Fishes 7(4):181. DOI: 10.3390/fishes7040181.
- Huerta Lwanga E, Thapa B, Yang X, Gertsen H, Salánki T, Geissen V, Garbeva P. 2018. Decay of low-density polyethylene by bacteria extracted from earthworm’s guts: A potential for soil restoration. The Science of the Total Environment 624:753–757. DOI: 10.1016/j.scitotenv.2017.12.144.
- Jâms IB. 2020. Estimating the size distribution of plastics ingested by animals. Nature Communications 11(1):1234567890. DOI: 10.1038/s41467-020-15406-6.
- Janeh M, Osman D, Kambris Z. 2019. Comparative analysis of midgut regeneration capacity and resistance to oral infection in three disease-vector mosquitoes. Scientific Reports 9(1):4556. DOI: 10.1038/s41598-019-50994-4.
- Jeon J-M, Park S-J, Choi T-R, Park J-H, Yang Y-H, Yoon J-J. 2021. Biodegradation of polyethylene and polypropylene by Lysinibacillus species JJY0216 isolated from soil grove. Polymer Degradation & Stability 191:109662. DOI: 10.1016/j.polymdegradstab.2021.109662.
- Jiang Z, Hu Z, Zeng L, Lu W, Zhang H, Li T, Xiao H. 2011. The role of the Golgi apparatus in oxidative stress: Is this organelle less significant than mitochondria? Free Radic. Free Radical Biology & Medicine 50(8):907–917. DOI: 10.1016/j.freeradbiomed.2011.01.011.
- Jorjão AL, Oliveira LD, Scorzoni L, Figueiredo-Godoi MLA, Prata MCA, Jorge AOC, Junqueira JC. 2018. From moths to caterpillars: Ideal conditions for Galleria mellonella rearing for in vivo microbiological studies. Virulescence 9(1):383–389. DOI: 10.1080/21505594.2017.1397871.
- Karpeta-Kaczmarek J, Augustyniak M, Rost-Roszkowska M. 2016. Ultrastructure of the gut epithelium in Acheta domesticus after long-term exposure to nanodiamonds supplied with food. Arthropod Structure & Development 45(3):253–264. DOI: 10.1016/j.asd.2016.02.002.
- Khyade V. 2018. Review on biodegradation of plastic through waxworm (Order: Lepidoptera; Family: Pyralidae). International Academic Journal of Economics 5(01):84–91. DOI: 10.9756/IAJE/V5I1/1810008.
- Kik K, Bukowska B, Sicińska P. 2020. Polystyrene nanoparticles: Sources, occurrence in the environment, distribution in tissues, accumulation and toxicity to various organisms. Environmental Pollution 262:114297. DOI: 10.1016/j.envpol.2020.114297.
- Klionsky DJ, Emr SD. 2000. Autophagy as a regulated pathway of cellular degradation. Science 290(5497):1717–1721. DOI: 10.1126/science.290.5497.1717.
- Købler C, Saber AT, Jacobsen NR, Wallin H, Vogel U, Qvortrup K, Mølhave K. 2014. FIB-SEM imaging of carbon nanotubes in mouse lung tissue. Analytical & Bioanalytical Chemistry 406(16):3863–3873. DOI: 10.1007/s00216-013-7566-x.
- Kong H, Kim H, Chung J, Jun J, Lee S, Kim H, Jeon S, Park S, Bhak J, Ryu C. 2019. The Galleria mellonella hologenome supports microbiota-independent metabolism of long-chain hydrocarbon beeswax. Cell Reports 26(9):2451–2465. DOI: 10.1016/j.celrep.2019.02.018.
- Kourtis N, Tavernarakis N. 2009. Autophagy and cell death in model organisms. Cell Death & Differentiation 16(1):21–30. DOI: 10.1038/cdd.2008.120.
- Lee Y, Park J, Ryu C, Gang KS, Yang W, Park Y-K, Jung J, Hyun S. 2013. Comparison of biochar properties from biomass residues produced by slow pyrolysis at 500°C. Bioresource Technology 148:196–201. DOI: 10.1016/j.biortech.2013.08.135.
- LeMoine C, Grove H, Smith CM, Cassone B. 2020. A very hungry caterpillar: Polyethylene metabolism and lipid homeostasis in larvae of the greater wax moth (Galleria mellonella). Environmental Science and Technology 54(22):14706–14715. DOI: 10.1021/acs.est.0c04386.
- Leonard EM, Pierce LM, Gillis PL, Wood CM, O’Donnell MJ. 2009. Cadmium transport by the gut and malpighian tubules of Chironomus riparius. Aquatic Toxicology (Amsterdam, Netherlands) 92(3):179–186. DOI: 10.1016/j.aquatox.2009.01.011.
- Li T-S, Marbán E. 2010. Physiological levels of reactive oxygen species are required to maintain genomic stability in stem cells. Stem Cells 28(7):1178–1185. DOI: 10.1002/stem.438.
- Lipovšek S, Leitinger G, Novak T, Janžekovič F, Gorgoń S, Kamińska K, Rost-Roszkowska M. 2018. Changes in the midgut cells in the European cave spider, Meta menardi, during starvation in spring and autumn. Histochemistry and Cell Biology 149:245–260. DOI: 10.1007/s00418-017-1623-z.
- Lipovsek S, Novak T. 2016. Autophagy in the fat body cells of the cave cricket Troglophilus neglectus Krauss, 1878 (Rhaphidophoridae, Saltatoria) during overwintering. Protoplasma 253(2):457–466. DOI: 10.1007/s00709-015-0824-3.
- Lipovšek S, Novak T, Dariš B, Hofer F, Leitinger G, Letofsky-Papst I. 2022. Ultrastructure of spherites in the midgut diverticula and Malpighian tubules of the harvestman Amilenus aurantiacus during the winter diapause. Histochemistry and Cell Biology 157:107–118. DOI: 10.1007/s00418-021-02046-0.
- Lou Y, Pererva E, Shan-Shan Y, Baiyun L, Bingfeng L, Nanqi R, Corvini PF-X, Defeng X. 2020. Biodegradation of polystyrene by greater wax moth larvae (Galleria mellonella L.) and the effect of co-diet supplementation on the core gut microbiome. Environmental Science and Technology 54(5):2821–2831. DOI: 10.1021/acs.est.9b07044.
- Luo L, Wang Y, Guo H, Yang Y, Qi N, Zhao X, Gao S, Zhou A. 2021. Biodegradation of foam plastics by Zophobas atratus larvae (Coleoptera: Tenebrionidae) associated with changes of the gut digestive enzymes activities and microbiome. Chemosphere 282:131006. DOI: 10.1016/j.chemosphere.2021.131006.
- Ma X, Wu Y, Jin S, Tian Y, Zhang X, Zhao Y, Yu L, Liang X-J. 2011. Gold nanoparticles induce autophagosome accumulation through size-dependent nanoparticle uptake and lysosome impairment. Agricultural Science & Technology Nano 5(11):8629–8639. DOI: 10.1021/nn202155y.
- Mattsson K, Ekvall MT, Hansson L-A, Linse S, Malmendal A, Cedervall T. 2015. Altered behavior, physiology, and metabolism in fish exposed to polystyrene nanoparticles. Environmental Science and Technology 49(1):553–561. DOI: 10.1021/es5053655.
- Matyja K, Rybak J, Hanus-Lorenz B, Wróbel M, Rutkowski R. 2020. Effects of polystyrene diet on Tenebrio molitor larval growth, development and survival: Dynamic energy budget (DEB) model analysis. Environmental Pollution 264:114740. DOI: 10.1016/j.envpol.2020.114740.
- Messinetti S, Mercurio S, Parolini M, Sugni M, Pennati R. 2018. Effects of polystyrene microplastics on early stages of two marine invertebrates with different feeding strategies. Environmental Pollution 237:1080–1087. DOI: 10.1016/j.envpol.2017.11.030.
- Mikulak E, Gliniewicz A, Przygodzka M, Solecka J. 2018. Galleria mellonella l. as model organism used in biomedical and other studies. Przegląg Epidemiologiczny 72(1):57–73.
- Mor R, Sivan A. 2008. Biofilm formation and partial biodegradation of polystyrene by the actinomycete Rhodococcus ruber. Biodegradation 19(6):851–858. DOI: 10.1007/s10532-008-9188-0.
- Morales-Ramos JA, Rojas MG, Shapiro-Ilan DI, Tedders WL. 2010. Developmental plasticity in Tenebrio molitor (Coleoptera: Tenebrionidae): Analysis of instar variation in number and development time under different diets. Journal of Entomological Science 45(2):75–90. DOI: 10.18474/0749-8004-45.2.75.
- Morissette G, Lodge R, Marceau F. 2008. Intense pseudotransport of a cationic drug mediated by vacuolar ATPase: procainamide-induced autophagic cell vacuolization. Toxicology & Applied Pharmacology 228(3):364–377. DOI: 10.1016/j.taap.2007.12.031.
- Morse RA. 1978. Honey bee pests, predators and diseases. Ithaca: Comstock Publishing/Cornell University Press.
- Oropesa AL, Floro AM, Palma P. 2017. Toxic potential of the emerging contaminant nicotine to the aquatic ecosystem. Environmental Science and Pollution Research 24(20):16605–16616. DOI: 10.1007/s11356-017-9084-4.
- Ostróżka A, Tiffert Z, Wilczek G, Rost-Roszkowska M. 2022. Can insecticide-free clean water regenerate the midgut epithelium of the freshwater shrimp after dimethoate treatment? Micron 155:103162. DOI: 10.1016/j.micron.2021.103162.
- Park SY, Kim CG. 2019. Biodegradation of micro-polyethylene particles by bacterial colonization of a mixed microbial consortium isolated from a landfill site. Chemosphere 222:527–533. DOI: 10.1016/j.chemosphere.2019.01.159.
- Peng B-Y, Chen Z, Chen J, Yu H, Zhou X, Criddle CS, Wu W-M, Zhang Y. 2020. Biodegradation of polyvinyl chloride (PVC) in Tenebrio molitor (Coleoptera: Tenebrionidae) larvae. Environment International 145:106106. DOI: 10.1016/j.envint.2020.106106.
- Peydaei A, Bagheri H, Gurevich L, de Jonge N, Nielsen JL. 2020. Impact of polyethylene on salivary glands proteome in Galleria melonella. Comparative Biochemistry & Physiology Part D, Genomics & Proteomics 34:100678. DOI: 10.1016/j.cbd.2020.100678.
- Peydaei A, Bagheri H, Gurevich L, de Jonge N, Nielsen JL. 2021. Mastication of polyolefins alters the microbial composition in Galleria mellonella. Environmental Pollution 280:116877. DOI: 10.1016/j.envpol.2021.116877.
- Pflugmacher S, Huttunen JH, Von Wolff MA, Penttinen OP, Kim YJ, Kim S, Mitrovic SM, Esterhuizen-Londt M. 2020. Enchytraeus crypticus avoid soil spiked with microplastic. Toxics 8(1):10. DOI: 10.3390/toxics8010010.
- Pitt JA, Trevisan R, Massarsky A, Kozal JS, Levin ED, Di Giulio RT. 2018. Maternal transfer of nanoplastics to offspring in zebrafish (Danio rerio): A case study with nanopolystyrene. The Science of the Total Environment 643:324–334. DOI: 10.1016/j.scitotenv.2018.06.186.
- Poprawa I, Chajec Ł, Chachulska-Żymełka A, Wilczek G, Student S, Leśniewska M, Rost-Roszkowska M. 2022. Ovaries and testes of Lithobius forficatus (Myriapoda, Chilopoda) react differently to the presence of cadmium in the environment. Scientific Reports 12:6705. DOI: 10.1038/s41598-022-10664-4.
- Poyraz Tinartas E, Goncu E, Koc K. 2021. Apoptotic and autophagic characteristics of perivisceral fat body remodeling of the greater wax moth Galleria mellonella and effects of juvenile hormone analog, fenoxycarb, on these processes. Archives of Insect Biochemistry and Physiology 107(1):e21780. DOI: 10.1002/arch.21780.
- Rehse S, Kloas W, Zar C. 2016. Zar short-term exposure with high concentrations of pristine microplastic particles leads to immobilisation of Daphnia magna. Chemosphere 153:91–99. DOI: 10.1016/j.chemosphere.2016.02.133.
- Repnik U, Turk B. 2010. Lysosomal–mitochondrial cross-talk during cell death. Mitochondrion 10(6):662–669. DOI: 10.1016/j.mito.2010.07.008.
- Riudavets J, Salas I, Pons MJ. 2007. Damage characteristics produced by insect pests in packaging film. Journal of Stored Products Research 43(4):564–570. DOI: 10.1016/j.jspr.2007.03.006.
- Rodriguez-Seijo A, Lourenço J, Rocha-Santos TAP, da Costa J, Duarte AC, Vala H, Pereira R. 2017. Histopathological and molecular effects of microplastics in Eisenia andrei Bouché. Environmental Pollution 220:495–503. DOI: 10.1016/j.envpol.2016.09.092.
- Romanelli D, Casartelli M, Cappellozza S, de Eguileor M, Tettamanti G. 2016. Roles and regulation of autophagy and apoptosis in the remodelling of the lepidopteran midgut epithelium during metamorphosis. Scientific Reports 9(6):32939. DOI: 10.1038/srep32939.
- Rost-Roszkowska M, Poprawa I, Chajec Ł, Chachulska-Żymełka A, Leśniewska M, Student S. 2020a. Effects of short- and long-term exposure to cadmium on salivary glands and fat body of soil centipede Lithobius forficatus (Myriapoda, Chilopoda): Histology and ultrastructure. Micron 137:102915. DOI: 10.1016/j.micron.2020.102915.
- Rost-Roszkowska M, Poprawa I, Chajec Ł, Chachulska-Żymełka A, Wilczek G, Skowronek M, Student S, Leśniewska M. 2022. Hazards related to the presence of cadmium in food – Studies on the European soil centipede, Lithobius forficatus. Science of the Total Environment 845(1):157298. DOI: 10.1016/j.scitotenv.2022.157298.
- Rost-Roszkowska M, Poprawa I, Chajec Ł, Chachulska-Żymełka A, Wilczek G, Wilczek P, Student S, Skowronek M, Nadgórska-Socha A, Leśniewska M. 2020b. Influence of soil contaminated with cadmium on cell death in the digestive epithelium of soil centipede Lithobius forficatus (Myriapoda, Chilopoda). European Zoological Journal 87(1):242–262. DOI: 10.1080/24750263.2020.1757168.
- Rost-Roszkowska M, Poprawa I, Chajec Ł, Chachulska-Żymełka A, Wilczek G, Wilczek P, Tarnawska M, Student S, Leśniewska M. 2021. Effects of cadmium on mitochondrial structure and function in different organs: studies on the soil centipede Lithobius forficatus (Myriapoda, Chilopoda). European Zoological Journal 88(1):632–648. DOI: 10.1080/24750263.2021.1912199.
- Rost-Roszkowska M, Vilimová J, Tajovský K, Chachulska-Żymełka A, Sosinka A, Kszuk-Jendrysik M, Ostróżka A, Kaszuba F. 2019. Autophagy and apoptosis in the midgut epithelium of millipedes. Microscopy and Microanalysis 25:1004–1016. DOI: 10.1017/S143192761900059X.
- Rost-Roszkowska MM, Janelt K, Poprawa I. 2018. The role of autophagy in the midgut epithelium of Parachela (Tardigrada). Zoomorphology 37:501–509. DOI: 10.1007/s00435-018-0407-x.
- Schirinzi GF, Pérez-Pomeda I, Sanchís J, Rossini C, Farré M, Barceló D. 2017. Cytotoxic effects of commonly used nanomaterials and microplastics on cerebral and epithelial human cells. Environmental Research 159:579–587. DOI: 10.1016/j.envres.2017.08.043.
- Schür C, Zipp S, Thalau T, Wagner M. 2019. Microplastics but not natural particles induce multigenerational effects in Daphnia magna. Environmental Pollution 113904. DOI: 10.1016/j.envpol.2019.113904.
- Scopetani C, Esterhuizen M, Cincinelli A, Pflugmacher S. 2020. Microplastics exposure causes negligible effects on the oxidative response enzymes glutathione reductase and peroxidase in the oligochaete Tubifex tubifex. Toxics 8:14. DOI: 10.3390/toxics8010014.
- Sehnal F. 1966. A critical study of the biome and biometry of the wax moth Galleria mellonella raised in varying conditions. Zeitschrift fur wissenschartliche Zoologie 174:53–82.
- Sharma S, Chatterjee S. 2017. Microplastic pollution, a threat to marine ecosystem and human health: A short review. Environmental Science and Pollution Research 24(27):21530–21547. DOI: 10.1007/s11356-017-9910-8.
- Shubin AV, Demidyuk IV, Komissarov AA, Rafieva LM, Kostrov SV. 2016. Cytoplasmic vacuolization in cell death and survival. Oncotarget 7(34):55863–55889. DOI: 10.18632/oncotarget.10150. PMID: 27331412; PMCID: PMC5342458.
- Skariyachan S, Patil AA, Shankar A, Manjunath M, Bachappanavar N, Kiran S. 2018. Enhanced polymer degradation of polyethylene and polypropylene by novel thermophilic consortia of Brevibacillus sps. and Anerinibacillus sp. screened from waste management landfills and sewage treatment plants polym. Degradation and Stability 149:52–68. DOI: 10.1016/j.polymdegradstab.2018.01.018.
- Sokolova M. 2004. Cadmium effects on mitochondrial function are enhanced by elevated temperatures in a marine poikilotherm, Crassostrea virginica Gmelin (Bivalvia: Ostreidae). The Journal of Experimental Biology 207(15):2639–2648. DOI: 10.1242/jeb.01054.
- Tarnawska M, Kafel A, Augustyniak M, Rost-Roszkowska M, Babczyńska A. 2019. Microevolution or wide tolerance? Level of stress proteins in the beet armyworm Spodoptera eqigua Hübner (Lepidoptera: Noctuidae) exposed to cadmium for over 150 generations. Ecotoxicology & Environmental Safety 178:1–8. DOI: 10.1016/j.ecoenv.2019.04.017.
- Tettamanti G, Grimaldi A, Pennacchio F, de Eguileor M. 2007. Lepidopteran larval midgut during prepupal instar: Digestion or self-digestion? Autophagy 3(6):630–631. DOI: 10.4161/auto.4908.
- Thompson RC, Swan SH, Moore CJ, Vom Saal FS. 2009. Our plastic age. Philosophical Transactions of the Royal Society B3641973–1976. DOI: 10.1098/rstb.2009.0054.
- Uwo MF, Ui‐Tei K, Park P, Takeda M. 2002. Replacement of midgut epithelium in the greater wax moth, Galleria mellonella, during larval‐pupal moult. Cell and Tissue Research 308(2):319–331. DOI: 10.1007/s00441-002-0515-1.
- Wang J, Yu Y, Lu K, Yang M, Li Y, Zhou X, Sun Z. 2017. Silica nanoparticles induce autophagy dysfunction via lysosomal impairment and inhibition of autophagosome degradation in hepatocytes. International Journal of Nanomedicine 12:809–825. DOI: 10.2147/IJN.S123596.
- Weber A, Scherer C, Brennholt N, Reifferscheid G, Wagner M. 2018. PET microplastics do not negatively affect the survival, development, metabolism and feeding activity of the freshwater invertebrate gammarus pulex. Environmental Pollution 234:181–189. DOI: 10.1016/j.envpol.2017.11.014.
- Wilczek G, Rost-Roszkowska M, Wilczek P, Babczyńska A, Szulińska E, Sonakowska L, Marek-Swędzioł M. 2014. Apoptotic and necrotic changes in the midgut glands of the wolf spider Xerolycosa nemoralis (Lycosidae) in response to starvation and dimethoate exposure. Ecotoxicology & Environmental Safety 101:157–167. DOI: 10.1016/j.ecoenv.2013.09.034.
- Włodarczyk A, Sonakowska L, Kamińska K, Marchewka A, Wilczek G, Wilczek P, Student S, Rost-Roszkowska M. 2017. The effect of starvation and re-feeding on mitochondrial potential in the midgut of Neocaridina davidi (Crustacea, Malacostraca). PloS One 12(3):e0173563. DOI: 10.1371/journal.pone.0173563.
- Włodarczyk A, Student S, Rost-Roszkowska M. 2019. Autophagy and apoptosis in starved and refed Neocaridina davidi (Crustacea, Malacostraca) midgut. Canadian Journal of Zoology 97:294–303. DOI: 10.1139/cjz-2018-0104.
- Wojda I, Staniec B, Sułek M, Kordaczuk J. 2020. The greater wax moth Galleria mellonella: Biology and use in immune studies. Pathogens and Disease 78(9):ftaa057. DOI: 10.1093/femspd/ftaa057.
- Wrońska N, Szlaur M, Zawadzka K, Lisowska K. 2022. The synergistic effect of triterpenoids and flavonoids—new approaches for treating bacterial infections? Molecules 27:847. DOI: 10.3390/molecules27030847.
- Xia T, Kovochich M, Liong M, Zink JI, Nel AE. 2008. Cationic polystyrene nanosphere toxicity depends on cell-specific endocytic and mitochondrial injury pathways. Agricultural Science & Technology Nano 2:85–96. DOI: 10.1021/nn700256c.
- Yamada-Onodera K, Mukumoto H, Katsuyaya Y, Saiganji A, Tani Y. 2001. Degradation of polyethylene by a fungus, Penicillium simplicissimum YK. Polymer Degradation & Stability 72(2):323–327. DOI: 10.1016/S0141-3910(01)00027-1.
- Yang J, Yang Y, Wu W-M, Zhao J, Jiang L. 2014. Evidence of polyethylene biodegradation by bacterial strains from the guts of plastic-eating waxworms. Environmental Science & Technology 48(23):13776–13784. DOI: 10.1021/es504038a.
- Yang L, Zhang Y, Kang S, Wang Z, Wu C. 2021. Microplastics in soil: A review on methods, occurrence, sources, and potential risk. The Science of the Total Environment 780:146546. DOI: 10.1016/j.scitotenv.2021.146546.
- Yang S-S, Brandon AM, Flanagan JCA, Yang J, Ning D, Cai S-Y, Fan H-Q, Wang Z-Y, Ren J, Benbow E, Ren N-Q, Waymouth RM, Zhou J, Criddle CS, Wu W-M. 2018. Biodegradation of polystyrene wastes in yellow mealworms (larvae of Tenebrio molitor Linnaeus): Factors affecting biodegradation rates and the ability of polystyrene-fed larvae to complete their life cycle. Chemosphere 191:979–989. DOI: 10.1016/J.CHEMOSPHERE.2017.10.117.
- Zaffagnini G, Martens S. 2016. Mechanisms of selective autophagy. Journal of Molecular Biology 428(9):1714–1724. DOI: 10.1016/j.jmb.2016.02.004.
- Zhou D, Shao L, Spitz DR. 2014. Reactive oxygen species in normal and tumor stem cells. Advances in Cancer Research 122:1–67.
- Zorova LD, Popkov VA, Plotnikov EY, Silachev DN, Pevzner IB, Jankauskas SS, Babenko VA, Zorov SD, Balakireva AV, Juhaszova M, Sollott SJ, Zorov DB. 2018. Mitochondrial membrane potential. Analytical Biochemistry 552:50–59. DOI: 10.1016/j.ab.2017.07.009.