Abstract
Heavy metals cause environmental pollution and produce toxic effects on organisms. Nickel (Ni) is a common metallic pollutant of aquatic ecosystems and potentially can produce multifarious changes in the body of aquatic organisms. The average nickel content in rivers is about 0.7 μg/l. As a result, the homeostasis of the affected organism is disturbed, and processes that can counteract the changes are activated. To better understand the effects of Ni in the freshwater environment and its fauna, it is important to establish whether all changes caused in cells and tissues by Ni exposure are reversible when the animal returns to the non-polluted environment. Thus, the main aim of the study was to analyze changes that occur after Ni exposure and after it is returned to non-contaminated water at various levels of the animal’s body. The freshwater shrimp Neocaridina davidi (Crustacea) was selected as the subject of the study. As the organ for studies, we chose the midgut because it is a barrier against stressors that enter the organism. A concentration of 3.63 mg Ni/l was selected for the experiment, at which approximately 50% mortality of the population was observed after 14 days. The midgut was analyzed using light and transmission electron microscopy (TEM), confocal microscopy, and flow cytometry for qualitative and quantitative results. When the organisms were transferred to clean water, a prolong exposure resulted in a decrease in the values of the analyzed parameters (e.g. ROS, cell death, etc.) proportional to the purification time. The recovery time was insufficient to return to control values in most analyzed groups. Nevertheless, the occurrence of regenerative changes suggests that freshwater shrimps are relatively tolerant to nickel, when the exposure time is short and the recovery time is long.
1. Introduction
Nickel (Ni) is the 24th most abundant element on earth, accounting for 3% of its composition. However, in terms of size, it occupies fifth place among the most common elements (Coogan et al. Citation1989). Ni occurs in various concentrations in all ecosystems (Das et al. Citation2019), e.g., in seawater at concentrations from 0.1 to 0.5 μg/l (Szefer Citation2002), while the average nickel content in rivers is 0.7 μg/l (Gaillardet et al. Citation2003). In animals, 1–10% of Ni in the diet is absorbed through the digestive tract (Von Burg Citation1997; Haber et al. Citation2000). Animal studies have shown the carcinogenic potential of various Ni compounds, such as nickel disulfide, nickel chloride, nickel oxide, nickel sulfate, etc (Diwan et al. Citation1992). The toxic functions of Ni are probably primarily due to its ability to replace other metal ions in enzymes and proteins or to bind to cellular compounds containing O, S, and N atoms, which are then inhibited (Coogan et al. Citation1989). One possible way Ni causes cell death and/or damage may be through oxidative reactions such as Ni-induced lipid peroxidation (LPO) (Cempel & Nikel Citation2006).
Bioindication allows us not only to assess changes in the aquatic environment caused by the presence of numerous xenobiotics but also to assess the changes that a xenobiotic can cause in organisms living in an environment (Tomczak & Dominiak Citation2016). It is also important to ascertain the degenerative alterations that organisms may undergo due to the presence of a stressor. N. davidi (Crustacea, Decapoda) is a freshwater, omnivorous, ornamental shrimp native to Taiwan. Although this species comes from Asia, it is currently widely distributed in freshwater around the world, and in 2018 it was detected in the Lower Oder (Jabłońska et al. Citation2018). These shrimps show tolerance to starvation both in the early stages of development (Pantaleão et al. Citation2015) and as adults (Włodarczyk et al. Citation2019a, Citation2019b; Ostróżka et al. Citation2022), and show tolerance to a range of water temperatures (Tropea et al. Citation2015). These animals, due to the simplicity of breeding, are very popular among aquarists around the world (Gehrmann Citation2021). Like most crustaceans, N. davidi plays a key role in the aquatic ecosystem, as they provide a food source for other organisms and also participates in the recycling of nutrients by feeding on decaying vegetation (Weber & Traunspurger Citation2016). Changes occurring in the body related to the accumulation of metals may affect the subsequent links of the trophic chain. Thus, this species seems to be a promising one among freshwater invertebrates (Włodarczyk et al. Citation2017, Citation2019a, Citation2019b; Ostróżka et al. Citation2022).
The study aimed to describe the changes in the epithelial cells of the middle and endodermal region of the digestive system (intestine and hepatopancreas) of the freshwater shrimp N. davidi resulting from exposure of animals to Ni, as well as to analyze regenerative changes that may occur as a result of rearing animals in clean water after previous exposure to nickel. The midgut of animals is considered one of the main ways for xenobiotics to enter the body and constitutes a barrier against their harmful effects (Bonelli et al. Citation2019). Experimental studies related to the analysis of the impact of various stressors on the structure of the midgut of N. davidi have shown that this organ is involved in maintaining homeostasis of the body by activation e.g., apoptosis, necrosis, autophagy, mitochondrial changes (Włodarczyk et al. Citation2017, Citation2019a, Citation2019b; Ostróżka et al. Citation2022). Thus, the specific objectives of the research were: (1) to analyze and describe the ultrastructural changes that occur in the epithelium of the intestine and hepatopancreas as a result of exposure of animals to nickel and as a result of placing the animals in clean water after prior exposure to nickel; (2) to analyze the activation/inhibition of cell death processes (autophagy, apoptosis, necrosis) in the epithelium of the intestine and hepatopancreas caused by Ni or clean water after Ni exposure. From a toxicological point of view, these studies can be considered chronic toxicity studies where animals are exposed to a toxic substance at lower concentrations. Such a study then lasts longer than in the case of acute toxicity, and the results are observed after a long time. Because concentrations causing effects in chronic tests are also usually lower than in acute toxicity, these tests are much more sensitive and closer to natural conditions (Traczewska Citation2011).
2. Material and methods
2.1. Organisms
The study material consisted of adult specimens (females and males) of the freshwater shrimp N. davidi. The animals were purchased from local breeders and bred in laboratory conditions and were acclimated to laboratory conditions for 1 month. The following parameters were kept constant in the aquarium: temperature 21°C, pH 7 and water hardness (10°GH). The animals were fed JBL Novo Prawn food for shrimps.
2.2. Experiment
The first stage of research aimed at determining the effect of Ni on the midgut of N. davidi was to determine the appropriate concentration of metal (Ni) to use in the study. Based on literature data (Ali & Fishar Citation2005; Leonard et al. Citation2011), three concentrations of an aqueous solution of Ni in the form of NiCl2 were tested experimentally: 0.2 mg/l, 2 mg/l, 8 mg/l (represented as follows: 0.09 mg/l, 0.9 mg/l and 3.63 mg/l of Ni respectively). To enable the gradual observation of potentially occurring changes, a concentration of 3.63 mg Ni/l was selected for the experiment, at which approximately 50% mortality of the population was observed after 14 days (LD50). The period of 2 weeks was chosen as the final period in which degenerative changes could be expected, while the period of 1 week was chosen as half of the period, finally causing a lethal effect in 50% of the population. The next research stage was related to determining whether rearing animals in clean aquarium water (after periods of 1 and 2 weeks of exposure to nickel) activates repair processes and, if so, whether these processes will be enough for the animals to survive. It turned out that some animals that survived 1 and 2 weeks in water with 8 mg of NiCl2/L survived the next experiment periods, i.e. 1 or 2 weeks in pure water. The animals were therefore divided into the following experimental groups (, ): CT – control – the animals stayed in clean water throughout the experiment, in the conditions described above; Ni 1:0 and Ni 2:0 – shrimps bred in the aquarium water with the addition of Ni for 1 and 2 weeks respectively; Ni 1:1 and Ni 1:2 – shrimps after a week’s cultivation in the aquarium water with the addition of Ni and returned to clean water and cultured for 1 and 2 weeks respectively; Ni 2:1 and Ni 2:2 – shrimps after 2 weeks of cultivation in the aquarium water with the addition of Ni and returned to clean water for 1 and 2 weeks respectively. During the experiment, shrimps were separately cultured in plastic cups and were fed ad libitum as animals from the control group. Every day, 10% of the water was replaced, and the plastic containers were cleared of excrement. Containers were kept in a shaded room to avoid the development of algae (Włodarczyk et al. Citation2017, Citation2019a, Citation2019b; Ostróżka et al. Citation2022).
Figure 1. Scheme of experimental setup. (a, b) N. davidi specimens. Bar = 0.54 cm. (c) Isolated midgut composed of the intestine and hepatopancreas. Scale bar = 0.23 cm.
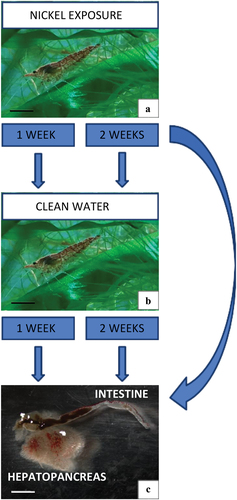
Table I. Representation of the experimental groups of adult specimens of N. davidi.
Before sectioning, the animals were anesthetized with chloroform and decapitated (Ostróżka et al. Citation2022). Two organs forming the midgut were isolated: the intestine and the hepatopancreas.
2.3. Total reflection X-ray fluorescence (TXRF) – analysis of the concentration of nickel in shrimp
The concentration of Ni in shrimp samples was determined with the use of total reflection X-ray fluorescence (TXRF) (S4 T-STAR spectrometer, Bruker, Germany). S4 T-STAR spectrometer is equipped with a 50 W power Mo target X-ray tube with a multilayer monochromator and an SSD detector. In order to estimate the concentration of Ni accumulated in shrimps as a result of the experiment, whole individuals (after removing their shells) were dried for 7 days at 110°C. Then the samples were mineralized at 210°C with the use of ETHOS UP Microwave Digestion mineralizer with a mixture of 4 mL of 65% nitric acid and 1 mL of hydrogen peroxide (30%) for 60 minutes (Merck Millipore, Darmstadt, Germany). Since TXRF is a reference method the addition of an internal standard is necessary to assess the quality of the quantitive results. For that matter 100 μL of 50 μg mL−1 Y was added to each sample before the mineralization step. Mineralized samples were then evaporated with the use of a heating plate at 140°C to the volume of approximately 1 mL. Subsequently, 10 μL of each sample was deposited on the surface of the siliconized quartz reflector and then dried for 10 minutes at 90°C with the use of a heating plate. Samples were analyzed by TXRF with Mo X-ray tube operating at 50 kV and 1000 µA. The measurements were conducted under ambient air conditions, with a counting time of 1000 seconds (Fernández‐Ruiz Citation2022). The accuracy of TXRF analysis was validated through the examination of three certified reference materials: Plankton BCR-414 (Joint Research Center), Lobster Hepatopancreas Reference Material for Trace Metals TORT-2 (National Research Council Canada), and Cod Tissue M-5 (Consortium “MODAS”). In the procedure, 60 mg of each material was digested using the ETHOS UP Microwave Digestion System (Milestone, Sorisole, Italy) with a mixture of 4 mL of 65% nitric acid and 1 mL of hydrogen peroxide (30%) at 210°C for 60 minutes. A 5 µg internal standard (Y) solution was added to the vessel before digestion. Subsequently, the digested solution was evaporated to a volume of approximately 1 mL at 140°C using a heating plate. 10-µL aliquots of each sample were then pipetted in three replicates on the surface of siliconized quartz reflectors and dried at 90°C before the TXRF analysis. Blank samples were prepared by addition of 4 mL of 65% nitric acid, 1 mL of hydrogen peroxide (30%), and 5 µg of Y and digested at 210°C for 60 minutes, so in the same way as certificates but without the addition of materials. The determined concentrations of Ni for BCR-414, TORT-2, and M-5 CodTis were 15.7 ± 0.1 mg/kg, 2.3 ± 0.5 mg/kg, and 0.16 ± 0.2 mg/kg, respectively. The certified values of Ni for BCR-414, TORT-2, and M-5 CodTis were 18.8 ± 0.8 mg/kg, 2.50 ± 0.19 mg/kg, and 0.136 mg/kg, respectively. The relative differences between the certified values and the determined concentrations did not exceed 15%, which is acceptable in the case of trace inorganic analysis.
2.4. Light microscope and histochemistry
Isolated organs were fixed in 2.5% glutaraldehyde (2 h, room temperature) and 1% osmium tetroxide in 0.1 M phosphate buffer (2 h, 4°C) and prepared according to standard methods for analysis using transmission electron microscopy (TEM) (Sonakowska et al. Citation2015, Citation2016; Sonakowska-Czajka et al. Citation2021). Epoxy resin blocks were cut on a Leica Ultracut UCT25 microtome. Semi-thin sections obtained (0.8 µm thick) were stained with 1% methylene blue in 0.5% borax (for visualization of the tissue topography). Semi-thin sections were stained with Schiff’s reagent (polysaccharide staining), bromophenol blue (BPB, protein staining), and Sudan black B (lipid staining) (Litwin Citation1985) according to the protocol described for N. davidi (Sonakowska et al. Citation2015; Ostróżka et al. Citation2022) and analyzed using the OLYMPUS B×60light microscope.
2.5. Transmission electron microscopy (TEM)
Ultrathin sections (70 nm thick) were cut on a Leica Ultracut EM UC7 RT ultramicrotome, and were transferred to copper grids and contrasted with uranyl acetate and lead citrate (Sonakowska et al. Citation2015, Citation2016). The material was analyzed in a Hitachi H500 electron microscope at 75 kV. The obtained results were documented on Kodak Electron Microscope Film 4489 negative films.
2.6. Qualitative analysis – confocal microscopy
2.6.1. Dihydroethidium (DHE) staining
Dihydroethidium (hydroethidine) is a dye commonly used to assess the location of reactive oxygen species (ROS) accumulation in cells and tissues (differentiation of ROS+ and ROS− cells). Isolated organs (intestine and hepatopancreas) were incubated with 30 μM of DHE (Invitrogen) and DAPI staining (1 μg/ml, 30 min, room temperature (RT), in the dark) and analyzed with an Olympus FluoView FV1000 confocal microscope with argon lasers: 405 nm laser (detection of DAPI) and 559 nm (detection of TMR). The result: blue fluorescence of nuclei and red fluorescence of localization of ROS (Rost-Roszkowska et al. Citation2022).
2.6.2. LysoTracker red staining
LysoTracker Red staining selectively accumulates in strongly acidic organelles, so it is commonly used to investigate lysosomes and autolysosomes. The dissected intestine and hepatopancreas of adult specimens of N. davidi from all experimental groups were incubated in 2.5 mmol/L LysoTracker Red DND-99 (Molecular Probes, L 7528; Thermo Fisher Scientific, Waltham, Massachusetts, USA) and 1 μg/ml DAPI (Sigma-Aldrich) as described by Poprawa et al. (Citation2022). The organs were observed using an Olympus FluoView FV1000 confocal microscope using a 559 nm laser for LysoTracker Red and a 405 nm laser for the DAPI dye. The result: blue fluorescence of nuclei and red fluorescence of strongly acid structures.
2.7. Quantitative analysis – flow cytometry
The dissected organs isolated from individuals from each experimental group were mechanically fragmented and homogenized to obtain a cell suspension. The procedure of preparing the cell suspension in PBS (phosphate-buffered saline) was described in our previous paper (Rost-Roszkowska et al. Citation2020).
2.7.1. Annexin V-FITC – viability assessment of cells
For quantitative analysis (the number of live cells, cells at early and late stages of apoptosis, and the number of necrotic cells in the midgut of N. davidi), the Annexin V-FITC apoptosis detection kit was used (Abcam, № ab14085). Quantitative measures of viable, early, and late apoptotic and necrotic cells in analyzed organs were obtained with the Annexin V-FITC (fluorescein isothiocyanate) Apoptosis Detection Kit (Abcam, № ab14085). This method is used to detect the early stages of apoptosis when the translocation of phosphatidylserine (PS) groups from the inner to the outer leaflet of the plasma membrane occurs. Green fluorescence origins form cells bound to the FITC-labeled Annexin V, while the red fluorescence origins form propidium iodide (PI). Thus, the distinction between necrotic cells (Annexin V-FITC–/PI +) and apoptotic cells (early apoptotic cells: Annexin V-FITC + /PI–; late apoptotic cells: Annexin V-FITC + /PI +) was enabled. Labeling was performed in the dark according to the manufacturer’s protocol. The cell suspensions were analyzed in a Beckman Coulter CytoFLEX flow cytometer using CytExpert Acquisition and Analysis Software (Beckman Coulter, United States). Light emission was induced in 5,000 cells from each experimental sample (Wilczek et al. Citation2023).
2.7.2. Muse® MultiCaspase kit
The Muse® MultiCaspase Kit (Merck Millipore, № MCH100109) is used in the quantitative analysis of live, caspase(+), caspase(+) and dead, total caspase(+), and dead cells. Thus, it was applied to analyze cell death processes: apoptosis and necrosis, as well as the participation of apoptotic caspases in the functioning of cells. The intestine and hepatopancreas isolated from N. davidi individuals from all study groups were prepared without fixation after preparing the homogenate for analysis according to the manufacturer’s protocol (https://welcome.cytekbio.com/hubfs/Amnis-and-Guava-Products/Muse-MultiCaspase-Kit-RSP.pdf) and analyzed in the Muse® cytometer.
2.7.3. Muse® oxidative stress kit
The Muse® Oxidative Stress Kit (Merck Millipore, № MCH100111) was used for the quantitative analysis of cell populations undergoing oxidative stress. It distinguishes two types of cell populations: live cells, which are ROS(−), and cells exhibiting ROS (ROS+ cells). The procedure was conducted according to the manufacturer’s protocol, and the measurements were performed using the Muse® Cell Analyzer (Rost-Roszkowska et al. Citation2022).
2.8. Statistical analysis
Statistical analyses were performed using the STATISTICA 13.1 software package. Normality was checked using the Shapiro-Wilk test. The data were tested for homogeneity of variance using Levene’s test of equality of error variances. The significance of the differences in the levels of analyzed quantitative parameters among experimental groups within the investigated organs was assessed using the NIR test, p < 0.05. The significance of differences in the levels of analyzed parameters between complementary experimental groups at both metal exposure times was checked with Student’s t-test, p < 0.05.
3. Results
3.1. TXRF analysis – concentration of nickel in shrimps
Contamination of the living environment of shrimps with nickel causes its accumulation in their bodies. The highest values were observed after one week of exposure to nickel (in the Ni 1:0 group). After two weeks of exposure (Ni 2:0 group), the concentration of the element was significantly lower than after one week (p = 0.00001), which may indicate that the shrimp was “getting used to” and adapting to the presence of the xenobiotic, with activation of detoxification processes. When shrimps after one week of exposure to nickel were returned to clean water, the concentration of Ni decreased significantly compared with the Ni 1:0 group but it was still present in the bodies of the animals. In the case of shrimps after two-week exposure to nickel, returning to clean water caused smaller changes of metal in their body ().
Table II. The average concentration of nickel (mean ± SD) in the body of N. davidi specimens using TXRF. Different letters (a, b, c, d) indicate statistically significant differences among experimental groups (NIR, p < 0.05, N = 6); *asterisks indicate significant differences compared to the control group (CT) (Student’s t–test, p < 0.05, N = 6).
3.2. Changes in ultrastructure of midgut epithelium of N. davidi
3.2.1. Changes in ultrastructure of intestine caused by nickel in the environment
3.2.1.1. Intestine of control specimens
The monolayer cylindrical epithelium of the N. davidi intestine consisted predominantly of digestive cells (D-cells) and regenerative cells (E-cells) located in the anterior part of the intestine (). A detailed description of the ultrastructure of N. davidi intestinal epithelial cells in control specimens can be found in our previous paper (Sonakowska et al. Citation2015; Sonakowska-Czajka et al. Citation2021), and degenerative processes (necrosis, apoptosis, autophagy) were described by Sonakowska et al. (Citation2016). During the analyses, the changes observed in the intestine concerned only D-cells. No ultrastructural changes were observed in E-cells. Therefore, the following description applies only to D-cells.
Figure 2. Ultrastructure of the intestine epithelium of N. davidi in experimental groups: CT (a–c), Ni 1:0 (d,e), Ni 2:0 (f–h). TEM. DC - digestive cells, n - nucleus, ER - cisterns of endoplasmic reticulum, mv - microvilli, l – midgut lumen, mvb - multivesicular bodies, m - mitochondria, EC - embryonic cell, bl - basal lamina, circle - degenerating mitochondria, star - vacuolated area of cytoplasm, v - vacuole, au - autophagosome, vm - visceral muscles. Scale bar: (a) 1.4 μm, (b) 0.7 μm, (c) 1.7 μm, (d) 0.8 μm, (e) 0.7 μm, (f) 0.8 μm, (g) 1.1 μm, (h) 2 μm.
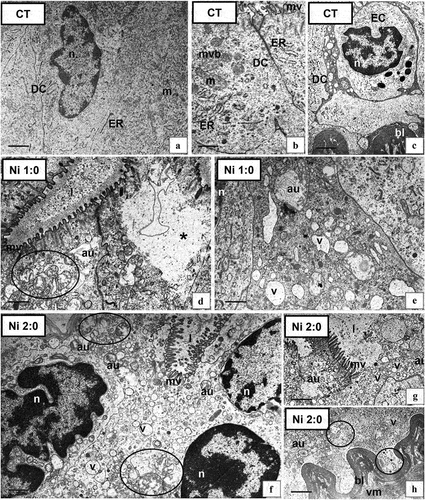
3.2.1.2. Intestine – changes caused by the presence of Ni in the environment
Ni exposure affected the ultrastructure of the digestive cells of N. davidi. Degenerative changes intensified with increasing exposure time to xenobiotics. After a week of exposure to nickel (Ni 1:0), most digestive cells showed no changes at the ultrastructural level. In some of the D-cells, vacuolated areas of the cytoplasm, shortened microvilli (), autophagosomes (), and degenerated mitochondria () were observed. After 2 weeks of exposure to nickel (Ni 2:0), numerous autophagosomes occurred in the cytoplasm of D-cells (). Mitochondria were damaged, distended, and lacking crista (). In addition, distended RER and SER cisterns and electron-lucent vacuoles were observed (). The apical membranes of some cells lost their continuity, which resulted in the release of the contents of the digestive cells into the lumen of the intestine (). In contrast, the basal lamina of the epithelium and the underlying visceral muscles did not show ultrastructural changes (). As a result of exposure of animals to Ni, intensification of the autophagy process was noted along with prolonged exposure of shrimps to the xenobiotic. Single necrotic changes occurred in the Ni 1:0 group, while they strongly increased in the Ni 2:0 group. During the ultrastructural studies, no apoptotic cells were observed in the intestinal epithelium.
3.2.1.3. Intestine – changes caused by the return of animals to an uncontaminated environment after previous exposure to Ni
Returning the shrimp to clean water resulted in a reduction in the intensity of degenerative changes in the D-cells. Epithelial regeneration was more effective with 2 weeks of reintroduction to clean water in both animals raised in nickel water for 1 and 2 weeks. The ultrastructure of the D-cells in the Ni 1:1 () shrimps was similar to Ni 1:0. Few autophagosomes and distorted mitochondria were observed (). The microvilli of some D-cells were still shortened (). However, in many cells, the mitochondria and ER cisterns had a normal structure. In the Ni 1:2 group, when the lifetime in clean water was extended by another week, the cytoplasm of the D-cells resembled the cytoplasm of the control group (). Necrotic cells with the cytoplasm poor in organelles were still observed (). The intestinal epithelium of individuals from the Ni 2:1 group was predominantly completely degenerated, resembling the Ni 2:0 group. The cells showed strong necrotic changes (). The presence of numerous autophagosomes was still observed, but the intensity of autophagy strongly decreased (). There was an increase in the number of degenerated mitochondria (). In the Ni 2:2 group, many D-cells were still necrotic (), had an electron-lucent cytoplasm with single autophagosomes (), damaged and distended mitochondria (), and bloated cisterns of ER. However, cells with the proper ultrastructure could be found, in which only the mitochondria were distended and had shortened cristae (). Despite the prolonged period of rearing animals in clean water after 2 weeks of exposure to Ni, degenerative changes at the ultrastructural level still occurred, including necrosis.
Figure 3. Ultrastructure of the intestine epithelium of N. davidi in experimental groups: Ni 1:1 (a,b), Ni 1:2 (c–e), Ni 2:1 (f–h), Ni 2:2 (i,j). TEM. n - nucleus, ER - cisterns of endoplasmic reticulum, mv - microvilli, l – midgut lumen, m - mitochondria, bl - basal lamina, circle - degenerating mitochondria, star - vacuolated area of cytoplasm, au - autophagosome, vm - visceral muscles. Scale bar: (a) 0.57 μm, (b) 0.83 μm, (c) 1.1 μm, (d) 1.1 μm, (e) 1 μm, (f) 1 μm, (g) 1.6 μm, (h) 0.6 μm, (i) 1.4 μm, (j) 1.2 μm.
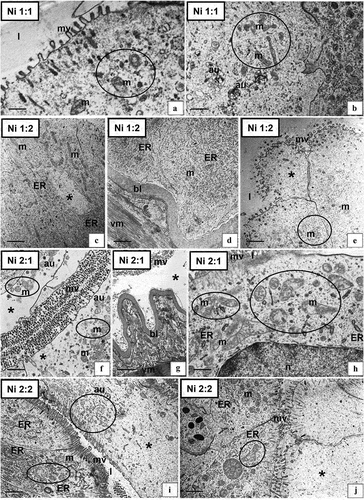
3.2.2. Changes in the ultrastructure of hepatopancreas caused by Ni in the environment
3.2.2.1. Hepatopancreas of control specimens
The hepatopancreas of the studied species consisted of two diverticula branching into numerous blind-end tubules. Within the tubules, 3 areas could be distinguished: distal, middle, and proximal. The distal zone, occupying the terminal part of tubules, was characterized by regenerative cells (E-cells) (), and the median zone by undifferentiated cells, while there were three types of cells in the proximal zone. The analysis did not show any changes in the cells occupying the distal and middle regions of the tubules of this organ. Therefore, the following description applied only to the cells present in the proximal region: F-, R-, and B-cells (). A detailed description of the ultrastructure of hepatopancreatic in control specimens can be found in our previous papers (Sonakowska et al. Citation2015; Sonakowska-Czajka et al. Citation2021).
Figure 4. Ultrastructure of the hepatopancreas of N. davidi in experimental groups: CT (a–c), Ni 1:0 (d,e), Ni 2:0 (f–h). TEM. EC - embryonic cell, FC - F-cell, RC - R- cell, BC - B-cell, n - nucleus, ER - cisterns of endoplasmic reticulum, mv - microvilli, l – midgut lumen, m - mitochondria, rm - reserve material, au - autophagosome, v – vacuole. Scale bar: (a) 1 μm, (b) 1 μm, (c) 0.86 μm, (d) 2.4 μm, (e) 1.4 μm, (f) 1 μm, (g) 0.8 μm, (h) 0.8 μm.
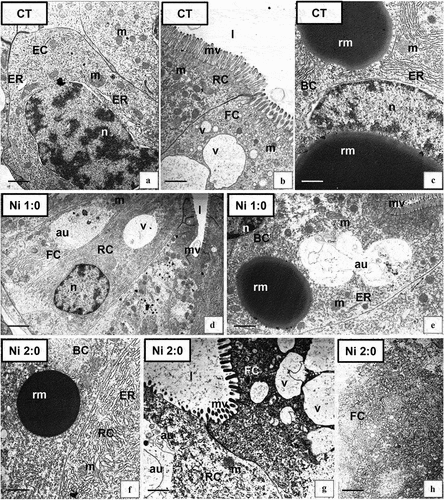
3.2.2.2. Hepatopancreas – changes caused by the presence of Ni in the environment
The presence of Ni in the environment of N. davidi affected the ultrastructure of the cells that built the proximal region of the hepatopancreatic tubules, i.e., the F, B, and R-cells. No changes were observed in the E-cells; therefore, further research concerns only the F, B, and R-cells. After a week of exposure to Ni (group Ni 1:0) single degenerative changes, vacuolated areas of the cytoplasm, as well as a slight increase in the number of autophagosomes were observed in the cytoplasm of F, B, and R-cells (). No changes related to storage materials accumulated in the B-cell cytoplasm appeared (). However, with prolonged exposure to the xenobiotic (2 weeks, Ni 2:0), the intensity of the observed degenerative changes increased (), as well as an increase in the number of autophagosomes in all the described cell types (). F-cells had strongly shortened microvilli, and their cytoplasm was characterized by numerous autophagosomes, degenerated mitochondria, distended cisterns of ER, and the presence of vacuolated areas (). B-cells and R-cells showed vacuolation within the cytoplasm and the presence of distended cisterns of ER (). TEM analysis did not show the presence of apoptosis in the described cell types.
3.2.2.3. Hepatopancreas – changes caused by the return of animals to an uncontaminated environment after previous exposure to Ni
Returning the shrimps to clean water after a week of Ni exposure resulted in partial cell regeneration at the ultrastructural level, especially after 2 weeks of living in clean water, where the ultrastructure of all cells forming the proximal tubular zone of this organ in Ni 1:2 animals resembled the control group. In the Ni 1:1 and Ni 1:2 groups, single degenerative changes were still observed (), indicating a necrotic process. In the Ni 1:1 and Ni 1:2 groups, an increase in the number of autophagic structures was observed, as well as a decrease in the number of spheres with electron-dense storage material. After transferring animals to pure water for 1 week (Ni 2:1), strong degenerative (necrotic) changes still affected all cell types of the proximal zone of hepatopancreatic tubules (). Thus, many distended organelles of B, F, and R-cells appeared in the electron-lucent cytoplasm. Strongly vacuolated areas of the cytoplasm were observed in the F-cells but vacuolization was not as strong as in the case of the Ni 2:0 group. Numerous spheres of storage material appeared in the cytoplasm of B-cells (), while the cytoplasm still showed strong vacuolization, distended cisterns of ER, and numerous autophagosomes. Two weeks of living in clean water after 2 weeks of xenobiotic exposure (Ni 2:2) were also insufficient for full tissue regeneration (). The number of vacuoles in the cytoplasm of F-cells decreased. In contrast, numerous autophagosomes and distended cisterns of ER were still observed (). B-cells were distinguished by the presence of storage materials during digestion, which did not occur in the Ni 2:1 group, and some large autophagosomes ().
Figure 5. Ultrastructure of the hepatopancreas of N. davidi in experimental groups: Ni 1:1 (a–c), Ni 1:2 (d,e), Ni 2:1 (f–h), Ni 2:2 (i–k). TEM. FC - F-cell, RC - R- cell, BC - B-cell, n - nucleus, ER - cisterns of endoplasmic reticulum, mv - microvilli, l – midgut lumen, m - mitochondria, rm - reserve material, au - autophagosome, v – vacuole. Scale bar: (a) 1.9 μm, (b) 1.3 μm, (c) 1.7 μm, (d) 0.8 μm, (e) 0.5 μm, (f) 0.7 μm, (g) 1 μm, (h) 1 μm, (i) 1 μm, (j) 1.7 μm, (k) 1 μm.
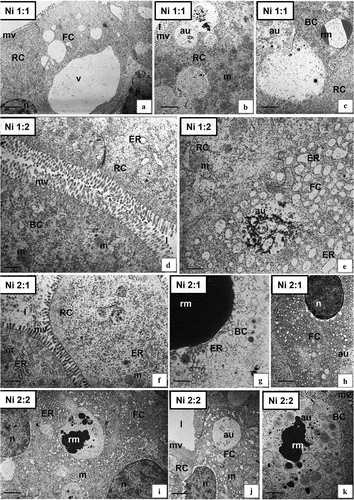
3.3. Histochemistry – analysis of changes in N. davidi intestinal and hepatopancreatic storage materials during the experiment
Treatment of shrimps with Ni did not affect the accumulation of storage materials within the epithelial cells in the intestine. These cells in all experimental groups, as in the control group, did not accumulate storage materials and were PAS-negative, BPB-negative, and Sudan Black B-negative (). The presence of Ni in the environment affected the storage materials accumulated in the cells. In the control group, B-cells accumulated storage materials in the form of polysaccharides and lipids. In the Ni 1:0 and Ni 2:1 groups, apart from the material containing lipids and polysaccharides, accumulation of proteins (BPB-positive) was observed, while in the other groups, storage materials were significantly reduced ().
Figure 6. Histochemical staining of the midgut of N. davidi. Light microscope. Scale bars: (A6) 7 μm, (F2) 12 μm, (A4, E1, F3, F7) 13 μm, (C2, C7, D5) 14 μm, (B1, F6) 14.5 μm, (A5, C4, E3, F4) 15 μm, (C3, D6, E2, E6) 16 μm, (A2, C1, D2, E4, E7) 17 μm, (A1, C6, D7, F1) 18 μm, (B2, B4, B6, D1) 19 μm, (D4) 21 μm, (A3, B3, B5, C5, F5) 22 μm, (A7) 23 μm, (B7) 26 μm.
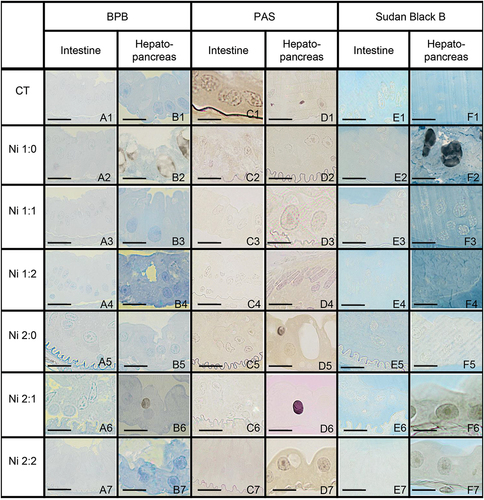
3.4. Autophagy in the intestinal and hepatopancreatic epithelium of N. davidi – qualitative analysis
Confocal microscopy analysis confirmed the presence of highly acidic structures, including autophagic structures in the cytoplasm of intestinal D cells. The presence of Ni in the shrimp’s environment influenced the intensification of the autophagy process, so the strongest signals came from D-cells in both organs of animals exposed to Ni for 2 weeks (Ni 2:0). In animals returned to uncontaminated water, a decrease in the intensity of signals emitted by strongly acid structures was observed. These signals were weaker, according to the time of purification ().
Figure 7. Localization of acid structures (red signals) in the intestine and hepatopancreas of N. davidi. Blue signals - nuclei. Confocal microscopy. Scale bar = 45 μm.
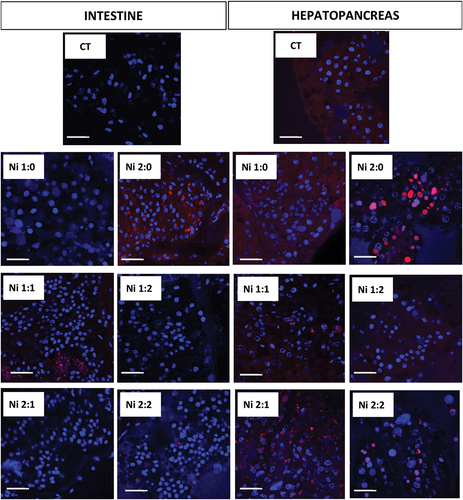
In the hepatopancreas, the intensity of signals from strongly acid structures was observed with increasing time of exposure of animals to Ni. Bringing animals back to life in clean water after previous exposure to the xenobiotic reduced the intensity of signals coming from strongly acid structures, although in groups Ni 1:1 and Ni 1:2 these signals were still strong (). Restoration to pure water in the case of the hepatopancreas resulted in cytoplasmic diffuse red signals in the Ni 1:1 group, smaller, numerous, clustered red signals in the Ni 2:1 group, and large concentrated red signals in the Ni 2:2 group. In the Ni 1:2 group, the red signals were weakening. Comparing both organs, signals were more numerous in the hepatopancreas than in the intestinal epithelium ().
3.5. Cell death in the intestine and hepatopancreas of N. davidi – a quantitative analysis
The quantitative analysis revealed a higher number of early and late apoptotic cells in the hepatopancreas than in the intestine, regardless of the duration of metal exposure (). After one and two weeks of metal exposure, the average percentages of apoptotic cells in the hepatopancreas were as follows: 5.1% and 6.5% for early apoptotic cells and 14.1% and 30.8% for late apoptotic cells (). In contrast, the percentage values in the intestine were, on average, nearly 5 times lower for early apoptotic cells and 7 and 15 times lower for late apoptotic cells after one and two weeks of metal exposure, respectively (Ni: 1 week and Ni: 2 weeks) (). Meanwhile, the average percentage of cells showing necrotic features in the hepatopancreas was 12.3% and 10.7%, while in the intestine, it was 9.5% and 8.3% after 1 week and 2 weeks of Ni exposure, respectively (). Only in the case of the hepatopancreas these differences were significant compared to the control group, regardless of the duration of metal exposure (Ni: 1 week: p = 0.001; Ni: 2 weeks: p = 0.01) (). Staying in clean water caused a gradual decrease in the number of apoptotic, late apoptotic, and necrotic cells in the hepatopancreas, reaching control levels, while in the intestine, their numbers increased after one or two weeks of exposure to uncontaminated water (). Quantitative analysis using the Muse® MultiCaspase Kit revealed that the presence of Ni affects the activation of caspases, which indicates the activation of the apoptotic process in the cell. After one week of Ni exposure, the level of caspase-positive live cells in the hepatopancreas increased threefold, while it did not increase in the intestine. Restoration to clean water contributed to a reduction in the level of active caspases, especially in the hepatopancreas (). The lowest percentage of live cells (LL) was observed in the intestines of individuals subjected to two weeks of Ni exposure and one week of purification (p < 0.00001). The level of this parameter in the intestine cells of Ni-exposed individuals was 31% lower than in the control group (). The lowest percentage of live caspase-positive cells (LR) was observed in the intestines of shrimps from Ni 1:2 group (p = 0.00001) (). In the remaining groups subjected to one week of exposure, the level of the analyzed parameter was also lower, on average by 40%, compared to the control group (p = 0.023). Conversely, the percentage of live caspase-positive cells in the intestine cells of individuals from Ni 2:1 group was nearly five times higher than in the other groups subjected to two-week metal exposure (p < 0.00001). Statistically significant differences were observed in the level of this parameter between the complementary purification groups at both exposure times (). The smallest percentage of caspase-positive dead cells, five times lower than in the control group, was observed in the intestines of individuals from Ni 1:2 group (p = 0.00001) (). In the remaining groups subjected to one-week exposure, the level of the analyzed parameter was lower than in the control group, but only in the case of shrimps from the Ni-exposed group not undergoing cleansing were these differences statistically significant compared to the control (p = 0.00003). Conversely, the percentage of caspase-positive dead cells in the intestines of individuals from Ni 2:1 group was nearly 0.5% higher than in the controls (CT) (p = 0.0007). The lowest value of this parameter (0.37%) was noted in the intestines of individuals exposed to Ni for two weeks and cleansed for two weeks (Ni 2:2). Statistically significant differences were found in the level of this parameter between complementary cleansing groups after one week of cleansing (p = 0.00004) (). In both times of exposure to Ni (one week/two weeks), the lowest percentage of dead cells (UR) was observed in the intestines of shrimps undergoing two-week cleansing (Ni 1:2, Ni 2:2). The obtained values differed significantly from the control values (0.38%, p = 0.00003; 0.26%, p = 0.00001, respectively) (). In the case of the hepatopancreas, regardless of the duration of Ni exposure, the highest percentage of live cells (LL) was observed in individuals undergoing two-week cleansing (Ni 1:2, Ni 2:2). In shrimps subjected to one-week exposure to Ni (Ni 1:0), the level of LL in the hepatopancreas of individuals was 6% higher than in the controls, and 5% in the case of individuals from Ni 2:0 group. Conversely, the smallest percentage of live cells was found in the hepatopancreas of individuals exposed to Ni for one week (75.8%) (). The highest percentage of live caspase-positive cells (LR) was observed in the hepatopancreases of individuals from Ni 1:0 group (p = 0.000001) (). Conversely, the smallest percentage of live caspase-positive cells (LR), on average seven times lower than in the control, was observed in the hepatopancreas of shrimps from Ni 1:2 group (p = 0.000001). One-week cleansing (Ni 1:1) caused a reduction in the value of this parameter to the control level (). On the other hand, the percentage of live caspase-positive cells in the hepatopancreas of individuals from Ni 2:1 group was nearly seven times higher than in the control group (p = 0.00001). Statistically significant differences were found in the level of this parameter between complementary cleansing groups in both exposure times (). The lowest percentage of caspase-positive dead cells, ten times lower than in the controls, was observed in the hepatopancreas of Ni 1:0 group (p = 0.016) (). Two weeks of exposure to Ni did not cause significant changes in the magnitude of this parameter compared to the control group. The highest percentage of caspase-positive dead cells, over twice as high as the control value, was found in the hepatopancreases in Ni 1:1 group (p = 0.00005). Statistically significant differences were observed in the level of this parameter between complementary purification groups after one week of purification (p = 0.00001) (). The percentage of dead cells (UR) in the hepatopancreases in Ni 1:1 or Ni 1:2 groups was significantly higher than in the controls, on average three times higher (). Statistically significant differences were found in the level of this parameter between complementary groups undergoing two-week purification (p = 0.025) ().
Figure 8. Quantitative cell death analysis in the midgut of N. davidi. (a,b) Percentage of early apoptotic cells (mean ± SE) in the intestines (a) and hepatopancreases (b) Subjected to nickel exposure for 1 week (Ni: 1 week) or 2 weeks (Ni: 2 weeks). (c,d) Percentage of late apoptotic cells (mean ± SE) in the intestines (c) and hepatopancreases (d) of N. davidi individuals subjected to nickel exposure for 1 week (ni: 1 week) or 2 weeks (Ni: 2 weeks). (e,f) Percentage of necrotic cells (mean ± SE) in the intestines (e) and hepatopancreases (f) of N. davidi individuals subjected to nickel exposure for 1 week (Ni: 1 week) or 2 weeks (Ni: 2 weeks). Different letters (a, b) indicate statistically significant differences between experimental groups: CT (control), Ni (nickel exposure), Ni:1 (nickel exposure and 1-week purification), Ni:2 (nickel exposure and 2-week purification), within each exposure time (1 or 2 weeks) using the NIR test, p < 0.05. Asterisks (*) indicate statistically significant differences between complementary experimental groups at both metal exposure times (Student’s t-test, p < 0.05).
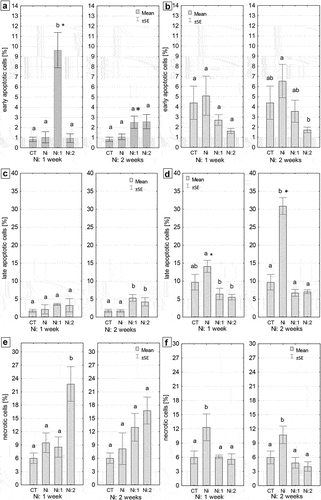
Figure 9. Quantitative analysis of caspase activity in the midgut of N. davidi. (a–d) The percentage of viable cells (a) Viable caspase-positive cells (b) Dead caspase-positive cells (c) and dead cells (d) in the intestine of N. davidi individuals exposed to nickel for 1 week (Ni: 1 week) or 2 weeks (Ni: 2 weeks). (e–h) The percentage of viable cells (e) Viable caspase-positive cells (f) Dead caspase-positive cells (g), and dead cells (h) in the hepatopancreas of N. davidi individuals exposed to nickel for 1 week (Ni: 1 week) or 2 weeks (Ni: 2 weeks). Different letters (a, b, c) indicate statistically significant differences between the experimental groups (CT - control; Ni - nickel exposure; Ni: 1 - nickel exposure and 1-week purification; Ni: 2 - nickel exposure and 2-week purification) within each exposure time (1 or 2 weeks), using the NIR test, p < 0.05. Asterisks (*) indicate statistically significant differences between complementary experimental groups at both metal exposure times (Student’s t-test, p < 0.05).
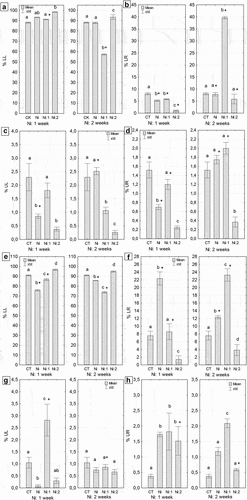
3.6. ROS in the intestine and hepatopancreas of N. davidi
Qualitative analysis using confocal microscopy (DHE staining) showed that the exposure of shrimps to Ni contributes to the intensification of oxidative stress in cells of the intestine and hepatopancreas. Red signals indicating the presence of ROS within the cytoplasm of cells or cell nuclei were numerous in the experimental groups of both analyzed organs. Returning the shrimp to clean water for a week did not reduce the intensity of the signals emitted by ROS-positive cells in both organs. Comparing the intestine and hepatopancreas, the oxidative stress caused by exposure of shrimps to Ni was higher in the hepatopancreas in all experimental groups. In both organs in the Ni 2:1 group, a strong increase in signals emitted by ROS-positive cells was observed (). The obtained results from qualitative analysis of oxidative stress are consistent with the results from quantitative analysis using a cytometer (Muse® Oxidative Stress Kit). Regardless of the duration of metal exposure, the percentage of ROS-positive cells in both organs was significantly higher compared to the control value (): approximately 2- and 3-fold higher in the intestine and 2- and 5-fold higher in the hepatopancreas from Ni 1:0, and Ni 2:0 groups (). After placing the animals in clean water, the percentage of ROS-positive cells in both organs was significantly higher compared to the control group and similar to the groups exposed to metal for one or two weeks. Only in the hepatopancreas of individuals exposed to Ni for one week was the percentage of ROS-positive cells significantly lower compared to individuals re-exposed to uncontaminated water.
Figure 10. (a) Localization of reactive oxygen species (ROS) (red signals) in the intestine and hepatopancreas of N. davidi. Blue signals - nuclei. Confocal microscopy. Scale bar = 22.5 μm. (b,c) The percentage (mean ± SE) of reactive oxygen species-positive (ROS+) cells in the intestine (b) and hepatopancreas (c) of N. davidi individuals subjected to nickel exposure for 1 week (Ni: 1 week) or 2 weeks (Ni: 2 weeks). Different letters (a, b, c) indicate statistically significant differences between experimental groups: CT (control), Ni (nickel exposure), Ni:1 (nickel exposure and 1-week purification), Ni:2 (nickel exposure and 2-weeks purification) within each exposure time (1 or 2 weeks) NIR test, p < 0.05. Asterisks (*) indicate statistically significant differences between complementary experimental groups at both metal exposure times (Student’s t-test, p < 0.05).
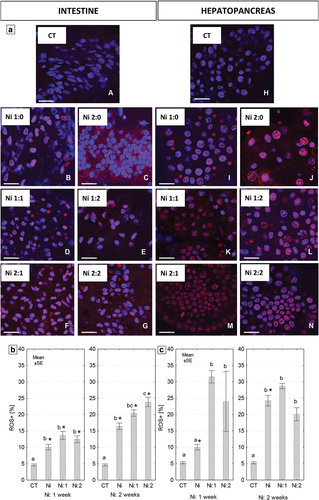
4. Discussion
4.1. Degenerative changes in the midgut epithelium of N. davidi under the influence of Ni
The midgut of invertebrates is considered a good model organ for the analysis of degenerative or regenerative processes triggered by the contact of cells with xenobiotics (Bonelli et al. Citation2019). Ultrastructural studies related to the influence of Ni on the midgut epithelial cells showed changes only in the D-cells of the intestine and the cells of the proximal section of the hepatopancreatic tubules. No changes were observed in the E-cells of the intestine and hepatopancreas. Similar observations were described in the experimental studies related to the starvation of adult N. davidi individuals (Włodarczyk et al. Citation2017, Citation2019a, Citation2019b) or treatment with a pesticide (Ostróżka et al. Citation2022). Thus, the results of studies using Ni confirm that regenerative cells must be protected by additional mechanisms, thanks to which they are not subject to degenerative processes.
Because N. davidi specimens were treated with Ni, analyses of the content of this metal were carried out to check whether it accumulates in the animals’ bodies and whether restoring them to life in clean water will affect the removal of Ni from the body. In control specimens, the level of Ni in the body was below the detection level. As a result of a week’s exposure to Ni, the shrimps were observed to accumulate this metal. Moreover, in this group, higher concentrations of xenobiotics accumulated in the body of shrimps were noted in comparison to the Ni 2:0 group. This phenomenon can be explained by the fact that the metabolism of animals begins to mobilize for detoxification processes. Ultrastructurally, however, after a week of Ni treatment of the animals, most of the cells that make up the midgut of N. davidi resembled the control group. It has been shown that Ni, as well as other metals such as Cd, Pb, Mn, and Co, can accumulate in the bodies of animals. Ezemonye et al. (Citation2019) estimated the concentration of Ni in the Benin River in Nigeria and also measured the element level in the shrimp Macrobrachium macrobrachion. With a Ni concentration in the river of 0.42 mg/L, the Ni values were recorded in the bodies of animals as 33.03 mg/kg. Among crustaceans, Ni accumulation has also been reported in the crayfish Astacus leptodactylus (Zarnescu et al. Citation2017) and the shrimp Penaeus semisulcatus (Yılmaz & Yılmaz Citation2007).
Ultrastructural analysis of N. davidi midgut showed a gradual increase in the intensity and frequency of the degenerative changes in the midgut (D-cells of the intestine and F, B, and R-cells of the hepatopancreas). The presence of stressors in the living environment of animals may be associated with the appearance of changes at the ultrastructural level of tissues. This may be influenced by the type of stressor and the duration of the stimulus. Similar observations also concerned other invertebrates exposed to heavy metals (Bednarska et al. Citation2016; Rost-Roszkowska et al. Citation2021b, Citation2022; Poprawa et al. Citation2022), including freshwater crustaceans (Schuwerack & Lewis Citation2003; Chiodi Boudet et al. Citation2015; Wu et al. Citation2015; Naboka et al. Citation2018) and marine crustaceans (Zhu et al. Citation2018; Vogt Citation2020; Barathkumar et al. Citation2022). Many studies have shown histological/histopathological changes in the midgut of insects, which were caused by the presence of metals in the animal’s living environment. Exposure to Cd of the freshwater shrimp Palaemon argentinus caused necrosis of the hepatopancreatic tubular epithelium (Chiodi Boudet et al. Citation2015). A similar effect of cadmium was observed in the freshwater crab Sinopotamon henanense (Wu et al. Citation2015) and Potamonautes warreni (Schuwerack & Lewis Citation2003). Studies on the exposure of freshwater crayfish Procambarus virginalis to Ni, lead (Pb), and manganese (Mn) showed that among the heavy metals studied, Ni ions had the most negative impact on mortality and changes in the hepatopancreas of crustaceans (Naboka et al. Citation2018).
Histochemical analysis of the intestine and hepatopancreas of N. davidi exposed to Ni compared to the control group showed a lack of storage materials in the D-cells of the intestine, while in the hepatopancreas, in the B-cells, the polysaccharides and lipids were significantly reduced. The reduction of reserve materials under the influence of an emerging stressor with the simultaneous activation of autophagy is considered to be a process that provides energy used to launch various detoxification processes in organs (Lipovšek & Novak Citation2016; Lipovšek et al. Citation2018; Włodarczyk et al. Citation2019a). For example, lipids are metabolized during lipophagy (selective autophagy) or lipolysis and, as a result, will affect cell physiology (Zechner et al. Citation2017). During autophagy, the degradation of various organelles and other cytoplasmic components takes place by surrounding them with a membranous structure, forming the autophagosome, then the autolysosome, and the residual body. There may also be digestion of reserve materials, the energy source for numerous biochemical changes in the cell. Thus, autophagy is a process that protects cells from death (Klionsky Citation2016, Citation2021). In the midgut of many invertebrates, the role of autophagy in maintaining the homeostasis of tissues and organs has been described (Lipovšek et al. Citation2018; Włodarczyk et al. Citation2019a; Rost-Roszkowska et al. Citation2021a, Citation2022). However, when too many autophagic structures are accumulated in the cytoplasm of cells, cell death is activated. In this case, intense autophagy triggers a degenerative process (Giusti et al. Citation2007; Franzetti et al. Citation2012). Studies on the effect of cadmium on the ultrastructure of the midgut cells of soil invertebrates have shown an increase in the intensity of degenerative processes or changes in the number of storage materials correlated with autophagy with the increasing duration of the animal’s exposure to the stressor (Rost-Roszkowska et al. Citation2020a, Citation2020b, Citation2021b, Citation2022). Necrosis is generally characterized as uncontrollable and energy-independent cell death, occurring due to severe trauma or a sudden stressor, as a result of which the cell cannot function and reacts with swelling (D’arcy Citation2019). Unlike necrosis, apoptosis is a highly regulated and controlled process that provides benefits during the life cycle of the organism (Menze et al. Citation2010). Caspases (cysteine proteases) play an important role in apoptosis (Genchi et al. Citation2020). The Ni ions release cytochrome C from the mitochondria, where cytochrome C activates the formation of caspase-9, activating caspases 3, 6, and 7. On the cell surface, Ni ions promote interaction between Fas (first apoptotic signal) and FasL (Fas ligand) to form a death-inducing signaling complex that includes FADD and procaspase-8 and −10. Thus, successive caspases (8, 10, 3, 6, and 7) are gradually activated (Genchi et al. Citation2020). The hepatopancreas of N. davidi seems to be the organ more sensitive to Ni compared to the shrimp intestine. Degenerative epithelial changes, both apoptosis and necrosis, appear faster in this organ. Compared with autophagy, which occurs in the hepatopancreas more intensively than in the intestine, it can be assumed that additional mechanisms will have to be activated in the intestine to protect the epithelium against the activation of cell death. However, taking into account the fact that too many autophagic structures (especially residual bodies) will activate cell death (Lipovšek & Novak Citation2016, Włodarczyk et al. Citation2019a; Rost-Roszkowska et al. Citation2021b, Citation2022), it can be concluded that in the hepatopancreas, as a result of intensive autophagy, apoptosis, and necrosis were activated faster than in the case of N. davidi intestine.
Oxidative stress plays an important role in the activation of cell death processes, occurring as a result of an increase in the level of ROS (Lobo et al. Citation2010). They perform important physiological functions by participating in intra- and extracellular signaling pathways involved in cell proliferation, differentiation, growth, aging, and cell apoptosis (Zuo et al. Citation2015). However, when excess ROS is not neutralized, oxidative stress may develop within cells, which may cause oxidative damage to many molecules, such as lipids, proteins, and nucleic acids (Sies Citation2015). As a result of the exposure of N. davidi specimens to Ni, a gradual increase in the level of ROS in both analyzed organs was observed with the increasing time of exposure of animals to Ni. Comparing the intestine and the hepatopancreas, it can be seen that this increase was stronger in the case of the latter organ, which would confirm more intensive degenerative processes in the hepatopancreas. Cd exposure of the freshwater shrimp Macrobrachium nipponense contributed to an increase in ROS, oxidative stress, and apoptosis in the hepatopancreas (Wang et al. Citation2021), which was also observed in the freshwater crayfish Procambarus clarkii (Wei et al. Citation2020) and crabs Cardisoma armarum, Goniopsis pelli, Callinectes amnicola, and Portunus validus (Lawal-Are et al. Citation2019). In N. davidi individuals exposed to Ni, it can be concluded that the increase in the level of ROS in the cells of both organs impacted the activation of degenerative processes. Numerous literature data indicate the influence of Ni on organelles and cellular structures in animals living in an environment contaminated with this metal. The increase in ROS levels then causes damage to DNA, lipids, and proteins. Consequently, organelles and cell structures are damaged, as a result of which cell death processes are initiated, both apoptotic and necrotic (Sah et al. Citation2017; Hołyńska-Iwan et al. Citation2023). Four hypotheses can explain Ni toxicity: Ni replaces another metal in metalloenzymes, Ni combines with non-metallic enzymes, Ni activates oxidative stress or causes changes, e.g., in DNA, proteins, and lipids (Macomber & Hausinger Citation2011). In the case of studies conducted on N. davidi, apoptosis and necrosis were observed, in which DNA is always damaged, but also an increase in the level of ROS. The main mechanism of Ni-induced oxidative stress in crabs has been described as involving the inhibition of oxidative defence markers (Blewett & Wood Citation2015). This additionally suggests for N. davidi the involvement of the last two mechanisms mentioned above. However, it is necessary to conduct further research related to changes in the levels of antioxidant enzymes (e.g., dismutase, peroxidase, catalase), metallothioneins, glutathione, or changes in mitochondrial activity (Blewett & Leonard Citation2017).
4.2. Changes in the midgut epithelium after restoring N. davidi to clean water after prior exposure to Ni
Ultrastructural analysis of cells within the intestine of N. davidi showed that returning shrimp to live in clean water after previous exposure to Ni was associated with a decrease in the intensity of degenerative changes in the D-cells. In individuals exposed to Ni for 1 week, it was observed that along with the longer time of purification of animals, cell organelles were gradually regenerated. However, in the case of animals living in water with Ni for 2 weeks, necrotic changes were still observed. Staining with the LysoTracker reagent also showed a decrease in the intensity of signals emitted by strongly acidic structures, proportional to the purification time. This proves that autophagy is no longer a process in the intestine required to further protect cells from the effects of the toxic metal (Rost-Roszkowska et al. Citation2020a, Citation2022). Restoring the shrimp to clean water also partially regenerates the cells that comprise the N. davidi hepatopancreas. Similar relationships were observed in N. davidi exposed to dimethoate and restored to clean water, where 1 week in clean water was not sufficient for complete recovery, especially with longer exposures (Ostróżka et al. Citation2022). Extending the time of returning to control conditions was associated with decreased frequency and intensity of the observed ultrastructural changes. However, especially with longer exposure to the stressor, sometimes even the same recovery time to control conditions is not sufficient, and degenerative changes are still present. During N. davidi purification, autophagy was suppressed in the intestine, while still intense in the hepatopancreas. In the shrimp Artemesia longinaris, the effect of salinity on the ultrastructure of hepatopancreatic cells was investigated. The animals were gradually and rapidly exposed to different salinity values at different intervals and then returned to baseline values for 30 days. Lowering the salinity level was associated with high mortality in shrimps, while in the case of small changes in salinity and reinstatement of control conditions, the hepatopancreas regained its unchanged structure (Masson et al. Citation2012). In the beetle P. oblongopunctatus, after 6 days of purification after previous exposure to Ni, the first symptoms of midgut regeneration appeared, manifested by more regular crypts in the midgut. The epithelium was also not regenerated when these animals were returned to an uncontaminated diet after zinc exposure. It is suggested that the results obtained from both the concentrations of accumulated metals and the state of the midgut may differ due to inter-individual variability (Bednarska et al. Citation2016).
The accumulation of reserve materials may be related to organisms living in a polluted environment or exposure to stressors that increase the animal’s energy expenditure related to detoxification and excretion of harmful substances (Sibly & Calow Citation1989). This can lead to the accumulation of storage substances in the organs (Bednarska et al. Citation2013). Some analyses related to energy budgets (accumulation of lipids, proteins, and polysaccharides) have been performed on several aquatic (Smolders et al. Citation2003; Verslycke et al. Citation2004; Graham & Thompson Citation2009) and terrestrial (Donker Citation1992; Bednarska et al. Citation2013) organisms. Changes in cells and tissues under the influence of toxic substances are more easily regenerated due to increased energy expenditure (Pook et al. Citation2009; Bednarska et al. Citation2013; Bednarska & Stachowicz Citation2013). The appearance of proteins in the Ni 2:1 group in the hepatopancreas of N. davidi may be associated with the intensification of synthesis of numerous enzymes, including those involved in cell death and detoxification processes (Sukharev et al. Citation1997). This is evidenced by a large increase in caspase-positive viable cells in the hepatopancreas. A similar increase was observed in the intestines of the same experimental group. However, the D-cells of the N. davidi intestine do not store reserve substances, and the proteins required for the synthesis of appropriate enzymes, including caspases, will be delivered from the organs storing them (here: the hepatopancreas).
During apoptosis, before any changes occur at the ultrastructural level, a whole cascade of processes is triggered to activate apoptotic enzymes (Zhang et al. Citation2018; Genchi et al. Citation2020). In the hepatopancreas, different relationships were observed between the time of restoring the animals to uncontaminated water and the activation of apoptosis and necrosis compared to the intestine. Returning to clean water resulted in N. davidi in a gradual and progressive decrease in the number of apoptotic, late-apoptotic, and necrotic cells in the hepatopancreas to control levels, while in the intestine, they increased after one or two weeks in uncontaminated water. Comparing the intensity of autophagy and apoptosis/necrosis, it can be concluded that in the intestine during the purification, the intensity of autophagy decreases, while apoptosis and necrosis are still intense. On the other hand, in the hepatopancreas, the opposite phenomenon was observed: an increase in autophagy and a decrease in the number of apoptotic/necrotic cells along with the prolonged life of animals in uncontaminated water. It can be concluded that in the hepatopancreas during the purification, intense autophagy will play a protective role, causing a decrease in the intensity of cell death processes. Thus, autophagy will play an important role in protecting the cells of this organ, which is known to be part of the survival pathway (Klionsky Citation2016, Citation2021). In the intestine, autophagy will not be a sufficient process to protect cells from further degeneration. However, because the intestine is considered to be one of the first organs (together with the epidermis covering the body) that constitutes a barrier for the whole organism against the influence of xenobiotics (Das Dores Teixeira et al. Citation2013), it can be assumed that the death of damaged cells in this organ will protect other organs against, for example, inflammation. This could also explain the gradual and faster regeneration of the hepatopancreas. In an experiment involving the exposure of P. oblongopunctatus beetles to heavy metals, the return to control conditions was associated with the appearance of metal-dependent apoptotic changes (Bednarska et al. Citation2016). Autophagy and apoptosis should not be treated as mutually exclusive phenomena but as cooperating processes in creating a cell response to changes in environmental conditions (Bednarska et al. Citation2016; Rost-Roszkowska et al. Citation2022). Here, autophagy mediates the induction of apoptosis (Eisenberg-Lerner et al. Citation2009; Franzetti et al. Citation2012).
The above-described results of research on Ni-treated shrimp, however, do not translate into the results of studies using Annexin V, which is used to analyze apoptosis at both its early and late stages. The lack of correlation between the results obtained using Annexin V and the Muse® MultiCaspase Kit can be explained by the adaptation of shrimps to the changing environment from Ni to clean water. Due to the lack of need to carry out intensive degenerative/regenerative processes, because the exposure to the xenobiotic ended, regulatory processes had to take place to stop the cell’s apoptosis. The high level of caspase-positive cells during the experiment on shrimps grown in Ni water, not corresponding to the high level of cells at the early and late stages of apoptosis, can also be explained by the fact that Annexin measures the level of apoptosis in two ways. The presence of phosphatidylserine on the surface of the cell membrane, which on the outer membrane appears due to the activity of caspase 3 (early stage of apoptosis), can be measured and, on the other hand, impaired permeability of the cell membrane (late apoptosis) can be analyzed. However, the overall level of caspases within the cytoplasm measured with the Muse® MultiCaspase Kit may increase due to the presence of other caspases, e.g., initiator caspases or executive caspases other than caspase-3, which will make the cell caspase-positive in action. The high level of caspases, which does not correspond to the high results obtained from the analysis using Annexin V, can also be explained by the fact that activated caspases are involved in cellular events other than apoptosis. The cleavage of a limited number of selected substrates plays a major role in the non-apoptotic functions of caspases: multi-cell proliferation, cell cycle regulation, and differentiation of various cells. In some cell types, differentiation may morphologically appear as incomplete apoptosis (Schwerk & Schulze-Osthoff Citation2003).
Qualitative and quantitative analysis of ROS levels in shrimps treated with Ni and reared in clean water after previous exposure to metal showed that reintroduction to uncontaminated water did not reduce the number of signals emitted by ROS(+) cells. A similar dependence of high ROS levels, despite being restored to control conditions, was observed by researchers checking the effect of restoring normal conditions to Litopenaeus vannamei shrimp previously stressed in insufficiently oxygenated water and water with high carbon dioxide levels. It has been suggested to block the expression of antioxidant genes, thus leaving the shrimp vulnerable to ROS-induced damage (Kniffin et al. Citation2014). The additional production of ATP due to stress suggests an increase in the body’s need for energy to maintain homeostasis in the organs. The antioxidant response resulting from the increase in ROS concentration due to the use of various stressors may vary depending on the stressor itself, the time of exposure, and recovery. Thus, it is necessary to conduct further research on the functioning of mitochondria to determine their role in the detoxification of both organs after exposure to Ni, or activation of various antioxidant enzymes such as catalase or dismutase.
5. Conclusions
Exposure to Ni proportionally intensified the degenerative changes in the analyzed parameters both in the intestine and in the hepatopancreas of the N. davidi digestive system. However, in the intestine, the changes were more intense than in the hepatopancreas, which may be related to the function of hepatopancreas in detoxification/regenerative processes. A prolonged return to clean water after previous exposure to Ni was associated with a decrease in the cell death proportional to the purification time. However, the recovery time was insufficient to return to the state characteristic for control animals. The occurrence of regenerative changes suggests that freshwater shrimps are relatively tolerant to Ni, provided the exposure time is short and the recovery time is long.
Acknowledgments
We thank Dr. Danuta Urbańska-Jasik (University of Silesia in Katowice, Poland) for her technical assistance and Richard Ashcroft (http://www.anglopolonia.com/home.html) for language correction.
Disclosure statement
No potential conflict of interest was reported by the author(s).
Additional information
Funding
References
- Ali MHH, Fishar MRA. 2005. Accumulation of trace metals in some benthic invertebrate and fish species revelant to their concentration in water and sediment of lake Qarun, Egypt. The Egyptian Journal of Aquatic Research 31(1):289–301.
- Barathkumar S, Padhi RK, Parida PK, Marigoudar SR. 2022. In vivo appraisal of oxidative stress response, cell ultrastructural aberration and accumulation in Juvenile Scylla serrata exposed to uranium. Chemosphere 300:134561. DOI: 10.1016/j.chemosphere.2022.134561.
- Bednarska AJ, Laskowski R, Pyza E, Semik D, Świątek Z, Woźnicka O. 2016. Metal toxicokinetics and metal-driven damage to the gut of the ground beetle Pterostichus oblongopunctatus. Environmental Science and Pollution Research 23(21):22047–22058. DOI: 10.1007/s11356-016-7412-8.
- Bednarska AJ, Stachowicz I. 2013. Costs of living in metal polluted areas: Respiration rate of the ground beetle Pterostichus oblongopunctatus from two gradients of metal pollution. Ecotoxicology 22(1):118–124. DOI: 10.1007/s10646-012-1008-y.
- Bednarska AJ, Stachowicz I, Kuriańska L. 2013. Energy reserves and accumulation of metals in the ground beetle Pterostichus oblongopunctatus from two metal-polluted gradients. Environmental Science and Pollution Research 20(1):390–398. DOI: 10.1007/s11356-012-0993-y.
- Blewett TA, Leonard EM. 2017. Mechanisms of nickel toxicity to fish and invertebrates in marine and estuarine waters. Environmental Pollution 223:311–322. DOI: 10.1016/j.envpol.2017.01.028.
- Blewett TA, Wood CM. 2015. Low salinity enhances Ni-mediated oxidative stress and sub-lethal toxicity to the green shore crab (Carcinus maenas). Ecotoxicology and Environmental Safety 122:159–170. DOI: 10.1016/j.ecoenv.2015.07.019.
- Bonelli M, Bruno D, Caccia S, Sgambetterra G, Cappellozza S, Jucker C, Tettamanti G, Casartelli M. 2019. Structural and functional characterization of Hermetia illucens larval midgut. Frontiers in Physiology 10(204):1–18. DOI: 10.3389/fphys.2019.00204.
- Cempel M, Nikel GJPJS. 2006. Nickel: A review of its sources and environmental toxicology. Polish Journal of Environmental Studies 15(3):375–382.
- Chiodi Boudet LN, Polizzi P, Romero MB, Robles A, Marco-Vecchio JE, Gerpe MS. 2015. Histopathological and biochemical evidence of hepatopancreatic toxicity caused by cadmium in white shrimp, Palaemonetes argentinus. Ecotoxicology & Environmental Safety 113:231–240. DOI: 10.1016/j.ecoenv.2014.11.019.
- Coogan TP, Latta DM, Snow ET, Costa M, Lawrence A. 1989. Toxicity and carcinogenicity of nickel compounds. CRC Critical Reviews in Toxicology 19(4):341. DOI: 10.3109/10408448909029327.
- D’arcy MS. 2019. Cell death: A review of the major forms of apoptosis, necrosis and autophagy. Cell Biology International 43(6):582–592. DOI: 10.1002/cbin.11137.
- Das Dores Teixeira A, Fialho MDCQ, Zanuncio JC, de Souza Ramalho F, Serrão JE. 2013. Degeneration and cell regeneration in the midgut of Podisus nigrispinus (Heteroptera: Pentatomidae) during post-embryonic development. Arthropod Structure & Development 42(3):237–246. DOI: 10.1016/j.asd.2013.02.004.
- Das KK, Reddy RC, Bagoji IB, Das S, Bagali S, Mullur L, Khodnapur JP, Biradar MS. 2019. Primary concept of nickel toxicity–An overview. Journal of Basic and Clinical Physiology and Pharmacology 30(2):141–152. DOI: 10.1515/jbcpp-2017-0171.
- Diwan BA, Kasprzak KS, Rice JM. 1992. Transplacental carcinogenic effects of nickel(II) acetate in the renal cortex, renal pelvis and adenohypophysis in F344/NCr rats. Carcinogenesis 13:1351–1357. DOI: 10.1093/carcin/13.8.1351.
- Donker MH. 1992. Energy reserves and distribution of metals in populations of the isopod Porcellio scaber from metal-contaminated sites. Functional Ecology 6(4):445–454. DOI: 10.2307/2389282.
- Eisenberg-Lerner A, Bialik S, Simon HU, Kimchi AJCD. 2009. Life and death partners: Apoptosis, autophagy and the cross-talk between them. Cell Death & Differentiation 16(7):966–975. DOI: 10.1038/cdd.2009.33.
- Ezemonye LI, Adebayo PO, Enuneku AA, Tongo I, Ogbomida E. 2019. Potential health risk consequences of heavy metal concentrations in surface water, shrimp (Macrobrachium macrobrachion) and fish (Brycinus longipinnis) from Benin River, Nigeria. Toxicology Reports 6:1–9. DOI: 10.1016/j.toxrep.2018.11.010.
- Fernández‐Ruiz R. 2022. TXRF spectrometry in the bioanalytical sciences: A brief review. X‐Ray Spectrometry 51(3):279–293. DOI: 10.1002/xrs.3243.
- Franzetti E, Huang ZJ, Shi YX, Xie K, Deng XJ, Li JP, Li Q, Yang W-Y, Zeng W-N, Casartelli M, Deng H-M, Cappellozza S, Grimaldi A, Xia Q, Tettamanti G, Cao Y, Feng Q. 2012. Autophagy precedes apoptosis during the remodeling of silkworm larval midgut. Apoptosis 17(3):305–324. DOI: 10.1007/s10495-011-0675-0.
- Gaillardet J, Viers J, Dupré B. 2003. Trace elements in river waters. In: Holland HD, Turekian KK, editors Treatise on geochemistry. Vol. 5. Oxford: Elsevier. pp. 225–272.
- Gehrmann S. 2021. Allgemeine Süßwasserkrebspraxis: Flusskrebse, Garnelen, Krabben, Mittelkrebse & Pfeilschwanzkrebse des Süß- und Brackwassers. Germany: BoD – Books on Demand.
- Genchi G, Carocci A, Lauria G, Sinicropi MS, Catalano A. 2020. Nickel: Human health and environmental toxicology. International Journal of Environmental Research and Public Health 17(3):679. DOI: 10.3390/ijerph17030679.
- Giusti F, Dallai L, Beani L, Manfredini F, Dallai R. 2007. The midgut ultrastructure of the endoparasite Xenos vesparum (Rossi) (Insecta, Strepsiptera) during post-embryonic development and stable carbon isotopic analyses of the nutrient uptake. Arthropod Structure & Development 36:183–197. DOI: 10.1016/j.asd.2007.01.001.
- Graham ER, Thompson JT. 2009. Deposit-and suspension-feeding sea cucumbers (Echinodermata) ingest plastic fragments. Journal of Experimental Marine Biology and Ecology 368(1):22–29. DOI: 10.1016/j.jembe.2008.09.007.
- Haber LT, Erdreicht L, Diamond GL, Maier AM, Ratney R, Zhao Q, Dourson ML. 2000. Hazard identification and dose response of inhaled nickel-soluble salts. Regulatory Toxicology and Pharmacology 31(2):210–230. DOI: 10.1006/rtph.2000.1377.
- Hołyńska-Iwan I, Sobiesiak M, Kowalczyk W, Wróblewski M, Cwynar A, Szewczyk-Golec A. 2023. Nickel ions influence the transepithelial sodium transport in the trachea, intestine and skin. Scientific Reports 13:6931. DOI: 10.1038/s41598-023-33690-2.
- Jabłońska A, Mamos T, Gruszka P, Szlauer-Łukaszewska A, Grabowski M. 2018. First record and DNA barcodes of the aquarium shrimp, Neocaridina davidi, in Central Europe from thermally polluted River Oder canal, Poland. Knowledge and Management of Aquatic Ecosystems 419(14):1–5. DOI: 10.1051/kmae/2018004.
- Klionsky D. 2016. Guidelines for the use and interpretation of assays for monitoring autophagy (3rd edition). Autophagy 12:1–222. DOI: 10.1080/15548627.2015.1100356.
- Klionsky D. 2021. Guidelines for the use and interpretation of assays for monitoring autophagy (4th edition). Autophagy 17:1–382. DOI: 10.1080/15548627.2020.1797280.
- Kniffin CD, Burnett LE, Burnett KG. 2014. Recovery from hypoxia and hypercapnic hypoxia: Impacts on the transcription of key antioxidants in the shrimp Litopenaeus vannamei. Comparative Biochemistry and Physiology Part B: Biochemistry and Molecular Biology 170:43–49. DOI: 10.1016/j.cbpb.2014.01.006.
- Lawal-Are AO, Moruf RO, Oluseye-Are SO, Isola TO. 2019. Antioxidant defense system alternations in four crab species as a bio-indicator of environmental contamination. Bulletin of the University of Agricultural Sciences & Veterinary Medicine Cluj-Napoca. Horticulture 76(1):73–80. DOI: 10.15835/buasvmcn-vm:2019.0001.
- Leonard EM, Barcarolli I, Silva KR, Wasielesky W, Wood CM, Bianchini A. 2011. The effects of salinity on acute and chronic nickel toxicity and bioaccumulation in two euryhaline crustaceans: Litopenaeus vannamei and Excirolana armata. Comparative Biochemistry and Physiology Part C: Toxicology & Pharmacology 154(4):409–419. DOI: 10.1016/j.cbpc.2011.07.011.
- Lipovšek S, Leitinger G, Novak T, Janžekovič F, Gorgoń S, Kamińska K, Rost-Roszkowska MM. 2018. Changes in the midgut cells in the European cave spider, Meta menardi, during starvation in spring and autumn. Histochemistry and Cell Biology 149(3):245–260. DOI: 10.1007/s00418-017-1623-z.
- Lipovšek S, Novak T. 2016. Autophagy in the fat body cells of the cave cricket Troglophilus neglectus Krauss, 1878 (Rhaphidophoridae, Saltatoria) during overwintering. Protoplasma 253(2):457–466. DOI: 10.1007/s00709-015-0824-3.
- Litwin JA. 1985. Light microscopic histochemistry on plastic sections. Progress in Histochemistry and Cytochemistry 16(2):1–84. PMID: 2417278. DOI: 10.1016/s0079-6336(85)80001-2.
- Lobo V, Patil A, Phatak A, Chandra N. 2010. Free radicals, antioxidants and functional foods: Impact on human health. Pharmacognosy Reviews 4(8):118–126. DOI: 10.4103/0973-7847.70902.
- Macomber L, Hausinger RP. 2011. Mechanisms of nickel toxicity in microorganisms. Metallomics 3(11):1153–62. DOI: 10.1039/c1mt00063b.
- Masson I, Díaz AC, Petriella AM. 2012. Effect of salinity changes on the midgut gland of Artemesia longinaris (Decapoda, Penaeidae). Latin American Journal of Aquatic Research 40(2):358–366. DOI: 10.3856/vol40-issue2-fulltext-10.
- Menze MA, Fortner G, Nag S, Hand SC. 2010. Mechanisms of apoptosis in Crustacea: What conditions induce versus suppress cell death? Apoptosis 15(3):293–312. DOI: 10.1007/s10495-009-0443-6.
- Naboka A, Marenkov O, Kovalchuk J, Shapovalenko Z, Nesterenko O, Dzhobolda B. 2018. Parameters of the histological adaptation of Marmorkrebs Procambarus virginalis (Lyko, 2017) (Decapoda, Cambaridae) to manganese, nickel and lead ions pollution. International Letters of Natural Sciences 70:24–33. DOI: 10.56431/p-tzw2qo.
- Ostróżka A, Tiffert Z, Wilczek G, Rost-Roszkowska M. 2022. Can insecticide-free clean water regenerate the midgut epithelium of the freshwater shrimp after dimethoate treatment? Micron 155(103162):1–16. DOI: 10.1016/j.micron.2021.103162.
- Pantaleão JAF, Barros-Alves SDP, Tropea C, Alves DF, Negreiros-Fransozo ML, López-Greco LS. 2015. Nutritional vulnerability in early stages of the freshwater ornamental “Red Cherry shrimp” Neocaridina davidi (Caridea: Atyidae). Journal of Crustacean Biology 35(5):676–681. DOI: 10.1163/1937240X-00002357.
- Pook C, Lewis C, Galloway T. 2009. The metabolic and fitness costs associated with metal resistance in Nereis diversicolor. Marine Pollution Bulletin 58(7):1063–1071. DOI: 10.1016/j.marpolbul.2009.02.003.
- Poprawa I, Chajec Ł, Chachulska-Żymełka A, Wilczek G, Student S, Leśniewska M, Rost-Roszkowska M. 2022. Ovaries and testes of Lithobius forficatus (Myriapoda, Chilopoda) react differently to the presence of cadmium in the environment. Scientific Reports 12(1):6705. DOI: 10.1038/s41598-022-10664-4.
- Rost-Roszkowska MM, Poprawa I, Chajec Ł, Chachulska-Żymełka A, Wilczek G, Wilczek P, Student S, Skowronek M, Nadgórska-Socha A, Leśniewska M. 2020a. Influence of soil contaminated with cadmium on cell death in the digestive epithelium of soil centipede Lithobius forficatus (Myriapoda, Chilopoda). European Zoological Journal 87(1):242–262. DOI: 10.1080/24750263.2020.1757168.
- Rost-Roszkowska MM, Janelt K, Poprawa I. 2020b. Ultrastructure of the midgut epithelium in three species of Macrobiotidae (Tardigrada: Eutardigrada: Parachela). Zoological Journal of the Linnean Society 188(3):788–796. DOI: 10.1093/zoolinnean/zlz052.
- Rost-Roszkowska MM, Vilimová J, Tajovský K, Šustr V, Ostróżka A, Kaszuba F. 2021a. Structure of the midgut epithelium in four diplopod species: Histology, histochemistry and ultrastructure. Arthropod Systematics & Phylogeny 79:295–308. DOI: 10.3897/asp.79.e67022.
- Rost-Roszkowska MM, Poprawa I, Chajec Ł, Chachulska-Żymełka A, Wilczek G, Wilczek P, Leśniewska M. 2021b. Effects of cadmium on mitochondrial structure and function in different organs: studies on the soil centipede Lithobius forficatus (Myriapoda, Chilopoda). European Zoological Journal 88(1):632–648. DOI: 10.1080/24750263.2021.1912199.
- Rost-Roszkowska MM, Poprawa I, Chajec Ł, Chachulska-Żymełka A, Wilczek G, Skowronek M, Leśniewska M. 2022. Hazards related to the presence of cadmium in food–studies on the European soil centipede, lithobius forficatus. Science of the Total Environment 845:157298. DOI: 10.1016/j.scitotenv.2022.157298.
- Sah D, Verma PK, Kumari KM, Lakhani A. 2017. Chemical partitioning of fine particle-bound As, Cd, Cr, Ni Co, Pb and assessment of associated cancer risk due to inhalation, ingestion and dermal exposure. Inhalation Toxicology 29:483–493. DOI: 10.1080/08958378.2017.1406563.
- Schuwerack PMM, Lewis JW. 2003. The mode of action of acute and chronic concentrations of waterborne Cd in the digestive gland of the acclimated infested freshwater crab (Potamonautes warreni). Cell and Tissue Research 312:249–263. DOI: 10.1007/s00441-002-0630-z.
- Schwerk C, Schulze-Osthoff K. 2003. Non-apoptotic functions of caspases in cellular proliferation and differentiation. Biochemical Pharmacology 66(8):1453–1458. DOI: 10.1016/S0006-2952(03)00497-0.
- Sibly RM, Calow P. 1989. A life-cycle theory of responses to stress. Biological Journal of the Linnean Society 37(1–2):101–116. DOI: 10.1111/j.1095-8312.1989.tb02007.x.
- Sies H. 2015. Oxidative stress: A concept in redox biology and medicine. Redox Biology 4:180–183. DOI: 10.1016/j.redox.2015.01.002.
- Smolders R, De Boeck G, Blust R. 2003. Changes in cellular energy budget as a measure of whole effluent toxicity in zebrafish (Danio rerio). Environmental Toxicology and Chemistry: An International Journal 22(4):890–899. DOI: 10.1002/etc.5620220429.
- Sonakowska L, Włodarczyk A, Poprawa I, Binkowski M, Śróbka J, Kamińska K, Kszuk-Jendrysik M, Chajec Ł, Zajusz B. 2015. Structure and ultrastructure of the endodermal region of the alimentary tract in the freshwater shrimp Neocaridina heteropoda (Crustacea, Malacostraca). PLoS One 10(5):e0126900. DOI: 10.1371/journal.pone.0126900.
- Sonakowska L, Włodarczyk A, Wilczek G, Wilczek P, Student S, Rost-Roszkowska MM. 2016. Cell Death in the Epithelia of the Intestine and Hepatopancreas in Neocaridina heteropoda (Crustacea, Malacostraca). PLoS One 11(2):e0147582. DOI: 10.1371/journal.pone.0147582.
- Sonakowska-Czajka L, Śróbka J, Ostróżka A, Rost-Roszkowska MM. 2021. Postembryonic development and differentiation of the midgut in the freshwater shrimp Neocaridina davidi (Crustacea, Malacostraca, Decapoda) larvae. Journal of Morphology 282:48–65. DOI: 10.1002/jmor.21281.
- Sukharev SA, Pleshakova OV, Sadovnikov VB. 1997. Role of proteases in activation of apoptosis. Cell Death & Differentiation 4(6):457–462. DOI: 10.1038/sj.cdd.4400263.
- Szefer P. 2002. Trace metals in the environment 5. Metals, metalloids and Radionuclides in the Baltic sea ecosystem. London: Elsevier. pp. 699–703.
- Tomczak E, Dominiak A. 2016. Organizmy żywe w systemie biomonitoringu jakości wody. Proceedings of ECOpole 10(1):315–323.
- Traczewska TM. 2011. Biologiczne metody oceny skażenia środowiska. Wrocław: Oficyna Wydawnicza Politechniki Wrocławskiej.
- Tropea C, Stumpf L, López Greco LS. 2015. Effect of temperature on biochemical composition, growth and reproduction of the ornamental Red Cherry shrimp Neocaridina heteropoda heteropoda (Decapoda, Caridea). PLoS One 10(3):e0119468. DOI: 10.1371/journal.pone.0119468.
- Verslycke T, Roast SD, Widdows J, Jones MB, Janssen CR. 2004. Cellular energy allocation and scope for growth in the estuarine mysid Neomysis integer (Crustacea: Mysidacea) following chlorpyrifos exposure: A method comparison. Journal of Experimental Marine Biology and Ecology 306(1):1–16. DOI: 10.1016/j.jembe.2003.12.022.
- Vogt G. 2020. Cytopathology and immune response in the hepatopancreas of decapod crustaceans. Diseases of Aquatic Organisms 138:41–88. DOI: 10.3354/dao03443.
- Von Burg R. 1997. Toxicology update. Journal of Applied Toxicology 17:425–431. DOI: 10.1002/(SICI)1099-1263(199711/12)17:6<425:AID-JAT460>3.0.CO;2-R.
- Wang L, Feng J, Wang G, Guan T, Zhu C, Li J, Wang H. 2021. Effects of cadmium on antioxidant and non-specific immunity of Macrobrachium nipponense. Ecotoxicology and Environmental Safety 224:112651. DOI: 10.1016/j.ecoenv.2021.112651.
- Weber S, Traunspurger W. 2016. Influence of the ornamental red cherry shrimp Neocaridina davidi (Bouvier, 1904) on freshwater meiofaunal assemblages. Limnologica 59:155–161. DOI: 10.1016/j.limno.2016.06.001.
- Wei K, Wei Y, Song C. 2020. The response of phenoloxidase to cadmium-disturbed hepatopancreatic immune-related molecules in freshwater crayfish Procambarus clarkii. Fish & Shellfish Immunology 99:190–198. DOI: 10.1016/j.fsi.2020.02.012.
- Wilczek G, Rost-Roszkowska M, Homa J, Szulińska E, Student S, Chajec Ł, Wiśniewska K, Surmiak-Stalmach K. 2023. How cadmium and copper change the sensitivity of the hemocytes of Steatoda grossa spider on immunostimulation: Qualitative and quantitative analysis. European Zoological Journal 90(2):624–642. DOI: 10.1080/24750263.2023.2237989.
- Włodarczyk A, Sonakowska L, Kamińska K, Marchewka A, Wilczek G, Wilczek P, Student S, Rost-Roszkowska M. 2017. The effect of starvation and re-feeding on mitochondrial potential in the midgut of Neocaridina davidi (Crustacea, Malacostraca). PLoS One 12(3):e0173563. DOI: 10.1371/journal.pone.0173563.
- Włodarczyk A, Student S, Rost-Roszkowska M. 2019a. Autophagy and apoptosis in starved and re-fed Neocaridina davidi (Crustacea, Malacostraca) midgut. Canadian Journal of Zoology 97(4):294–303. DOI: 10.1139/cjz-2018-0104.
- Włodarczyk A, Wilczek G, Wilczek P, Student S, Ostróżka A, Tarnawska M, Rost-Roszkowska M. 2019b. Relationship between ROS production, MnSOD activation and periods of fasting and re-feeding in freshwater shrimp Neocaridina davidi (Crustacea, Malacostraca). PeerJ 7:e7399. DOI: 10.7717/peerj.7399.
- Wu H, Li Y, Lang X, Wang L. 2015. Bioaccumulation, morphological changes, and induction of metallothionein gene expression in the digestive system of the freshwater crab Sinopotamon henanense after exposure to cadmium. Environmental Science and Pollution Research 22(15):11585–11594. DOI: 10.1007/s11356-015-4419-5.
- Yılmaz AB, Yılmaz L. 2007. Influences of sex and seasons on levels of heavy metals in tissues of green tiger shrimp (Penaeus semisulcatus de Hann, 1844). Food Chemistry 101(4):1664–1669. DOI: 10.1016/j.foodchem.2006.04.025.
- Zarnescu O, Petrescu AM, Gaspar A, Craciunescu O. 2017. Effect of sublethal nickel chloride exposure on crayfish, Astacus leptodactylus ovary: An ultrastructural, autometallographic, and electrophoretic analyses. Microscopy and Microanalysis 23(3):668–678. DOI: 10.1017/S1431927617000496.
- Zechner R, Madeo F, Kratky D. 2017. Cytosolic lipolysis and lipophagy: Two sides of the same coin. Nature Reviews Molecular Cell Biology 18(11):671–684. DOI: 10.1038/nrm.2017.76.
- Zhang Y, Chen X, Gueydan C, Han J. 2018. Plasma membrane changes during programmed cell deaths. Cell Research 28(1):9–21. DOI: 10.1038/cr.2017.133.
- Zhu QH, Zhou ZK, Tu DD, Zhou YL, Wang C, Liu Z-P, Gu W-B, Chen Y-Y, Shu M-A. 2018. Effect of cadmium exposure on hepatopancreas and gills of the estuary mud crab (Scylla paramamosain): Histopathological changes and expression characterization of stress response genes. Aquatic Toxicology (Amsterdam, Netherlands) 195:1–7. DOI: 10.1016/j.aquatox.2017.11.020.
- Zuo L, Zhou T, Pannell BK, Ziegler AC, Best TM. 2015. Biological and physiological role of reactive oxygen species – The good, the bad and the ugly. Acta Physiologica 214:329–348. DOI: 10.1111/apha.12515.