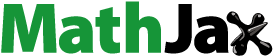
Abstract
Determination of the thermodynamic parameters of complex compounds of rare earth metals in aqueous solutions is of considerable interest for understanding the hydrometallurgical processes associated with the extraction and separation of yttrium and lanthanides from solutions of complex multicomponent composition. The composition of these solutions, as a rule, includes the following anions: SO42-, CO32-, PO43-, F-, which form strong complexes of the inner-sphere type, and anions, which form weak outer-sphere complexes, which primarily include complexes of the metal with halogens Cl-, Br-, I-. By the method of direct potentiometry with the use of a bromide-selective electrode while maintaining the ionic strength constant in the medium of sodium perchlorate, the stability constants (lgK) of the complexes of bromine with cerium, neodymium and samarium at the first stage were determined, the numerical values of which were 0,346; 0,257; 0,387, respectively. Based on the results obtained, the Gibbs energies of formation for the complexes under consideration are calculated. A method is proposed for extrapolating literature or experimental data, which makes it possible to calculate the values of thermodynamic stability constants for the entire series of lanthanides and yttrium. The fundamental possibility of using this method is shown by the example of calculating thermodynamic parameters for bromide complexes of rare-earth metals.
1. Introduction
One of the key challenges of the 21st century is the rational use of natural resources and the processing of existing waste and expired products (Innocenzi et al., Citation2018; Lucas, Lucas, Le Mercier, Rollat, & Davenport, Citation2015). Both in the man-made raw materials, represented by the technological dumps of production, and in the used devices, there is a significant amount of valuable elements that can be reused (Bykova, Alekseenko, Pashkevich, & Drebenstedt, Citation2021; Fialkovsky, Citation2020a; Savchenkov et al., Citation2020). These elements include the group of rare earth metals (REM), which includes 15 lanthanides, as well as yttrium and scandium, which are widely used in modern industry (Jha et al., Citation2016; Moulin, Hussonnois, Brillard, & Guillaumont, Citation1975; Omodara et al., Citation2019).
When processing rare-earth metals containing raw materials by hydrometallurgical methods, solutions of a complex water-salt composition are formed. In addition to valuable components, the multicomponent system usually includes the following cations: Al3+, Fe3+, Ca2+, Mg2+, Na+, K+ and anions: SO42-, NO3-, CO32-, HCO3-, PO43-, HPO42-, Cl-, Br-. These impurities can have a significant effect on the processes of extraction and separation of rare-earth metals, leading to a positive or negative synergistic effect (Fialkovsky, Citation2020b). For example, cations Al3+, Fe +, Ca2+, Mg2+ emulsify the organic phase in the processes of liquid extraction, and anions such as SO42-, NO3-, CO32- form complexes with the metal, reducing its extraction from aqueous solution (Fialkovsky, Citation2020a; Migdisov, Williams-Jones, Brugger, & Caporuscio, Citation2016; Qi, Citation2018). In addition to the complex composition, the processes of REM extraction are affected by the pH of the medium, on which the equilibrium in the hydrolysis reactions substantially depends. The influence of these factors on the distribution of equilibrium forms of rare-earth metals in solution must be quantitatively taken into account when designing various technological processes, and for this it is necessary to have data on the stability constants of complex compounds of rare-earth metals with various ligands formed in solution (Cheremisina, Cheremisina, Ponomareva, & Fedorov, Citation2020; Voropanova, Citation2016).
As a rule, the extraction and separation of rare earth metals from solutions are carried out by the methods of ion exchange sorption (Kurdiumov, Timofeev, Maltsev, & Lebed, Citation2020) or liquid extraction using various types of extractants (Savelyeva, Citation2011; Xie, Zhang, Dreisinger, & Doyle, Citation2014), of which the most economically feasible and environmentally safe, and, therefore, more preferable for processing secondary raw materials are carboxylic acids or organophosphorus extractants (Voropanova & Pukhova, Citation2018).
There are many data in the literature on the study of the complexation of REM with such ligands as: SO42-, CO32-, PO43-, which form stable complexes with REM ions, these complexes have an inner-sphere nature (Persson, Citation2010; Persson, D'Angelo, De Panfilis, Sandström, & Eriksson, Citation2008). Thus, the formation of sulfate complexes of LnSO4+ and Ln(SO4)2- with rare-earth metals (where Ln3+ is a rare-earth metal cation) was considered in (McDowell and Coleman, Citation1972; Sekine et al., Citation1965). Phosphate complexes of the species LnH2PO42+, LnHPO4+ LnPO40 Ln(HPO4)2- are described in detail in (Bingler and Byrne, Citation1989; Rao, Citation1970). Carbonate complexes of REM LnCO3+, LnHCO32+ and Ln(CO3)- are considered in the following works (Cantrell and Byrne, Citation1987a, Citation1987b). Of the REM halide complexes, the complexes with fluorine are sufficiently well studied (Menon, Citation1987 ; Moulin et al., Citation1975; Walker and Choppin, 1967). The complexes with NO3-, Cl-, Br- are characterized by an outer-sphere structure, they are less stable and, therefore, less studied. The most complete data on the stability constants of chloride complexes are presented in the works (Breen and Horrocks, Citation1983; Moulin et al., Citation1975; Peppard et al., Citation1962; Sekine et al., Citation1965) for nitrate complexes in the works (Choppin and Graffeo, Citation1965; Tanaka and Yamashita, Citation1984; Wood, Citation1990), and data on the stability constants for bromide complexes are almost absent from the literature, and are available only for a small number of elements of the group of rare-earth metals (Pearson, Citation1988). In this work, the process of complexation of the bromine anion with various REM cations is considered.
In general, the formation of complexes of lanthanide ions with bromide anions at the first stage occurs according to reaction (1):
(1)
(1)
According to the law of mass action, the concentration constant of stability for the complex under consideration can be calculated by the formula (2):
(2)
(2)
where the expressions in square brackets denote the equilibrium molar concentrations of the corresponding ions in the solution.
Taking into account the activity of ions in solution, we can obtain expression (3) for the thermodynamic stability constant:
(3)
(3)
where
– is the activity coefficients of the corresponding ions.
Expression (4) connects the thermodynamic and concentration stability constant:
(4)
(4)
where Пγ – is the product of the activity coefficients of the corresponding ions.
2. Research methods
The stability constants of complex compounds of rare-earth metals in solutions can be obtained on the basis of experimental data. The most commonly used are potentiometric, conductometric, extraction and spectroscopic methods of analysis(Bataev & Krutous, Citation2000; Lobacheva & Dzhevaga, Citation2020; Lugina & Davidenko, Citation1977).
This study was carried out by the method of direct potentiometry by measuring the potential difference in an electrochemical cell consisting of bromide-selective and chlorine-silver electrodes. The concentration of rare-earth metal cations in the solution remained constant, and the concentration of bromide anions changed by the same value with each subsequent measurement(Byrne & Li, Citation1995).
During the experiment, the ionic strength of the solution was kept constant due to the presence of a background electrolyte in the solution. Traditionally, alkali metal or ammonium perchlorates are used as such an electrolyte. The perchlorate anion is not a complexing ligand for rare-earth metals; therefore, the presence of these anions in solution does not interfere with the correct measurement(Choppin, Citation1997; Dezhi, Citation2018). Chemical grade reagents were used to prepare solutions of sodium perchlorate NaClO4 and sodium bromide NaBr in various concentration ranges. Solutions with cations of rare-earth metals were obtained by dissolving salts of Ln(ClO4)3 grade "chemically pure", in order to avoid hydrolysis, the resulting solution was acidified with perchloric acid.
To measure the potential difference, we used an ESr-10103/3.5 silver chloride electrode and an ELIS-131Br ion-selective electrode with a crystal membrane. To keep the potential of the reference electrode constant over time, it was immersed in a saturated sodium chloride solution. The potential of the ion-selective electrode, strictly speaking, depends not only on the concentration of bromide ions in the solution, but also on the concentration of other halide ions, therefore, to eliminate the interfering effect of chlorine ions, the measurement was carried out through a salt bridge filled with a one-molar solution of sodium perchlorate. The use of this electrolyte was due to the need to minimize the diffusion of electrolytes from different parts of the electrolytic cell (Lobacheva & Dzhevaga, Citation2017; Voropanova, Citation2016). The measurements were carried out at a constant temperature. A schematic diagram of an electrochemical cell for measuring the potential difference at various concentrations of bromide anion is shown in .
Figure 1. Electrochemical cell for measuring the potential difference at various concentrations of bromide - anion.
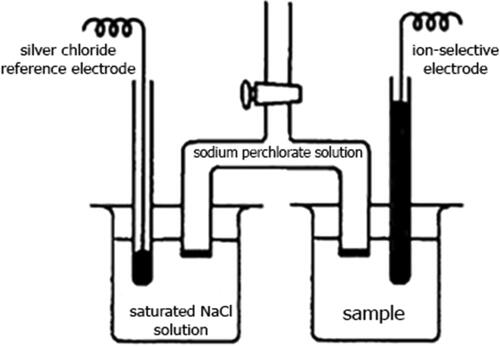
For each element (samarium, neodymium, cerium), two experiments were carried out: in the first, the concentration of rare earth metals was 0.1 mol/L, in the second 0.05 mol/L, while the concentration of the bromide anion varied. The range of changes in the concentration of the bromide anion in the first experiment was in the range from 0.1 mol/L to 0.3 mol/L, and in the second from 0.2 mol/L to 0.4 mol/L.
To ensure the reliability of the data obtained, the value of the potential difference was measured 30 minutes after the establishment of a constant value.
The calculation of the equilibrium concentration of the bromide anion was carried out through the equation of the calibration dependence, for the construction of which a series of standard solutions of sodium bromide was used. The resulting calibration dependence is shown in .
3. Results of potentiometric determination of stability constants
The equation of the calibration dependence (5) for a given cell has the form:
(5)
(5)
where E is the value of the potential difference in the cell, mV.
The equilibrium concentrations of the bromide anion in the measured solutions were calculated using EquationEquation (5)(5)
(5) . shows the potential difference values measured at different concentrations of bromide anion for two experiments for each metal cation with different ionic strength.
Table 1. Measured values of the potential difference during the experiments.
Bjerrum's approach is a classical method for solving problems of determining the concentration stability constants. This method involves the determination of the average coordination number for ligands and metal ions prone to stepwise complexation. The average coordination number, also called the Bjerrum formation function, ranges from zero, for the case when the metal is practically not caked, to the maximum coordination number possible for a given complexing ion.
The average coordination number () for a system containing only one metal cation, assuming that the complexes are mononuclear, can be expressed by EquationEquation (6)
(6)
(6) .
(6)
(6)
where k1, k2, k3 are the formation constants for the first, second and third stages, respectively
As a result of the transformation, expression (6) can be reduced to the form (7):
(7)
(7)
Also, the Bjerrum formation function can be calculated according to the formula (8):
(8)
(8)
In the case of studying the outer-sphere complexes, which also include complexes of rare-earth metals with bromide anions, the stability constants for the second and third steps can be neglected due to the low stability of these complexes (Omodara et al., Citation2019). Thus, expression (7) can be reduced to the form (9):
(9)
(9)
From the obtained equilibrium concentrations of the ligand, taking into account the activity coefficients, the thermodynamic constants of stability for the first stage were calculated for bromide complexes of samarium, cerium and neodymium, the values for which are presented in .
Table 2. Thermodynamic stability constants and lgK values for the complexes CeBr2+, SmBr2+, NdBr2+.
The Gibbs energies of formation of the CeBr2+, SmBr2+, NdBr2+ complexes were calculated using the formula (10):
(10)
(10)
where T is the temperature, K; R is the universal gas constant, J/(mol · K).
The values obtained for the Gibbs energies of the formation of bromide complexes are presented in .
Table 3. Gibbs energies of formation of bromide complexes of cerium, samarium, and neodymium.
4. Mathematical modeling
In the course of potentiometric measurements, values were obtained for the thermodynamic stability constants of bromide complexes of neodymium, samarium, and cerium. In order to verify the reliability of the data obtained and to extrapolate them to the entire series of rare earth metals, mathematical methods were used. One of the methods for making such an assessment is the data extrapolation method proposed in (Lee & Byrne, Citation1992; Siziakova, Ivanov, & Nikitina, Citation2019; Siziakova & Ivanov, Citation2020).
After analyzing the literature data, an almost complete absence of values for the stability constants of lanthanide bromide complexes was found, and therefore they became the main object of this study. The literature contains data for only three complexes: CeBr2+ (Spahiu & Bruno, Citation1995), EuBr2+ (Spahiu & Bruno, Citation1995), and GdBr2+ (Glushko, Citation2004). However, even these scarce data for individual elements can be used for a sufficiently accurate assessment of the stability constants of the entire series of rare earth metals.
The closeness of the chemical properties of rare earth metals determines the existing trends and patterns in the processes of complex formation. The method is based on the construction of linear regression equations of the form (11):
(11)
(11)
where Мi - denotes a metal cation,
- are the values of the stability constants for the first step for complexes of two metals in the REM series,
- are the parameters of the linear regression equation for a pair of metals under consideration.
To evaluate the stability constants with a specific ligand according to this method, it is necessary to have only one stability constant in the series, obtained experimentally, as well as an array of data on the stability constants with other ligands. On this basis, to assess the stability constants of bromide complexes of rare-earth metals, a sample was made based on the data available in the literature on various inorganic complexes.
shows a typical dependence of the stability constants of inorganic complexes on the example of Sm3+ and Eu3+.
Figure 3. Linear regression of the dependence of the stability constants of inorganic ligands for the Eu3+/Sm3+ pair.
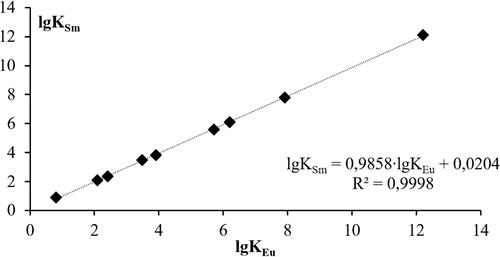
The parameters of the linear regression equation S and I, obtained as a result of calculation through the stability constants of the complexes of europium, cerium, and gadolinium, are presented in .
Table 4. Coefficients of the linear regression equation S and I for the pairs Eu/Ln, Ce/Ln and Gd/Ln.
The evaluation of stability constants can be carried out for a relatively large number of elements, with a sufficient amount of necessary data. By introducing an additional term into EquationEquation (11)(11)
(11) , the accuracy of the method can be increased. When evaluating the stability constant in terms of two elements, EquationEquation (11)
(11)
(11) takes the form (12):
(12)
(12)
Where - are the values of the stability constants for the first step for complexes of three metals in the REM series,
- are the parameters of the linear regression equation for the three metals under consideration.
The parameters of the linear regression equation S and I, calculated through the stability constants of the complexes of europium, cerium, and gadolinium, are presented in .
Table 5. Coefficients of the linear regression Equationequation S1, S2(1)
(1) and I for triples of elements.
To test this method, carbonate complexes were selected, since data for the entire series of lanthanides are known for them. Europium was chosen as M2 in EquationEquation (11)(11)
(11) , as an element located in the middle of the lanthanide series. shows a comparison of the results of mathematical modeling with data from literary sources (Cheremisina et al., Citation2020; Moulin et al., Citation1975; Spahiu & Bruno, Citation1995). shows that the constructed model correlates well enough with the experimental data, however, in the region of light REM, the model shows some excess over the literature values, and in the region of heavy REM, the opposite trend is observed.
Figure 4. Comparison of literature data with modeling data for stability constants of carbonate complexes (red - literature data; blue - simulation result).
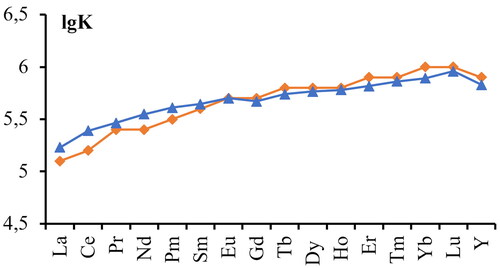
Further modeling was carried out using experimentally obtained thermodynamic stability constants of bromide complexes. In this case, good convergence of the obtained stability constants was observed for the entire series with the values known from the literature.
To estimate the variance of the values of stability constants obtained by the two methods, the standard deviation (SD) was calculated by formula (13):
(13)
(13)
shows the result of calculating the stability constants for REM bromide complexes, as well as the values of the standard error of the arithmetic mean (SEM), which were calculated using the formula (14):
(14)
(14)
Table 6. Summary values for the calculated stability constants of bromide complexes calculated by two methods.
For the resulting values, the following regularities are observed: the stability of the complexes decreases from La to Lu, Y - in this case, it exhibits properties close to heavy lanthanides. This dependence can be explained from the standpoint of the principle of hardness - softness of Pearson's acids and bases(Cheremisina, Schenk, Cheremisina, Ponomareva, & Saint Petersburg Mining University, 2019; Pouillon & Doyle, Citation1988). According to this principle, the bromide anion is a transition base, and REM cations are hard acids, while using this atomic number of the element, due to the effect of lanthanide compression caused by the filling of the 4f orbital by an increased ionic radius, which leads to an increase in charge density and hardness of the cation (Bazhin, Aleksandrova, Kotova, & Suslov, Citation2019). In order for the ligand to displace the water molecule from the hydration shell of the ion, the ligand must displace the water molecule from the hydration shell of the ion, so that the ligand displaces the water molecule from the hydration shell of the ion, so that the ligand displaces the water molecule from the hydration shell of the ion, it is necessary to use crystallizer. The water molecule, according to Pearson, is a more rigid base than the bromide anion, its direct displacement from the first hydration shell of the metal cation is difficult, and therefore, for bromides, only the formation of less stable outer-sphere complexes is possible. Moreover, due to the increase in their rigidity, their rigidity increases.
The Gibbs energies of formation of bromide complexes of rare-earth metals were calculated using the averaged values of thermodynamic stability constants. The data obtained are presented in .
Table 7. Average values of Gibbs energies of formation of bromide complexes of REM.
5. Conclusion
In the course of experimental studies by potentiometric methods the values of thermodynamic constants of stability of bromide complexes were obtained: Values of lgK were 0.3461 0.2548, 0.3874, respectively.
The values of the stability constant for the complex are in good agreement with the value from the thermodynamic database, which is 0.3793.
The method of extrapolation of the experimental data for calculating the stability constants for the whole REM series based on the data set on the complexation of REM with different inorganic ligands and at least one value of the stability constant for the REM complex within the sought series is proposed. The values of linear regression equations I and S coefficients necessary for calculations of stability constants for REM complexes with the considered inorganic ligands are given in table no.
Using this method, the stability constants of REM bromide complexes in the first step and their Gibbs energies of formation were calculated. The obtained values of lgK were 0.389 - for 0.349 - for
0.252 - for
The calculated standard deviation for the obtained values of the stability constants of bromide complexes, taking into account one available series term and two series terms, show that the greatest dispersion of values is observed for the following elements: La, Er, Ho, and Yb. Such behavior of the calculated values can be explained by less stability of the outer sphere complexes of heavy REM and as a result by a wide scatter of the literature data which was the base of the statistical evaluation. The presence of lanthanum in this series may be associated with its extreme position in the REM series (the first member of the series). Dispersion values for light elements take the lowest value in the REM series, which may be associated with greater stability of their complexes, it may be due to the fact that extrapolation was performed through the stability constants for Ce, Eu and Gd, which belong to the light REM.
Values of stability constants obtained by potentiometric method for complexes of neodymium, samarium and cerium are in good agreement with data obtained by extrapolation of values from literature sources.
Based on the thermodynamic stability constants obtained in the course of modeling, the Gibbs energies of formation were calculated for all REMs; the obtained values can be used in various thermodynamic calculations in the future.
Acknowledgment
This research was done at the expense of subsidies for carrying out state task in the field of scientific activity for 2021 № FSRW-2020-0014.
Disclosure statement
No potential conflict of interest was reported by the authors.
References
- Bataev, I.M., & Krutous, A.I. (2000). Problems of stability of halide complex compounds of rare earth elements in alcohols. Bull. Far East. Fed. Univ. Econ. Manag, 15, 96–109.
- Bazhin, V.Y., Aleksandrova, T.A., Kotova, E.L., & Suslov, A.P. (2019). A modern view of anomalies in the metal groups of the periodic system of D.I.Mendeleev. Journal of Mining Institute, 239(5), 520–527. doi:10.31897/pmi.2019.5.520
- Bingler, L.S., & Byrne, R.H. (1989). Phosphate complexation of gadolinium(III) in aqueous solution. Polyhedron, 8(10), 1315–1320. doi:10.1016/S0277-5387(00)86529-8
- Breen, P. J., & Horrocks, W. D. (1983). Europium(III) luminescence excitation spectroscopy. Inner-sphere complexation of europium(III) by chloride, thiocyanate, and nitrate ions. Inorganic Chemistry, 22(3), 536–540. doi:10.1021/ic00145a034
- Bykova, M. V., Alekseenko, A. V., Pashkevich, M.A., & Drebenstedt, C. (2021). Thermal desorption treatment of petroleum hydrocarbon-contaminated soils of tundra, taiga, and forest steppe landscapes. Environmental Geochemistry and Health, 43(6), 2331–2346. doi:10.1007/s10653-020-00802-0
- Byrne, R.H., & Li, B. (1995). Comparative complexation behavior of the rare earths. Geochimica et Cosmochimica Acta, 59(22), 4575–4589. doi:10.1016/0016-7037(95)00303-7
- Cantrell, K. J., & Byrne, R. H. (1987a). Rare earth element complexation by carbonate and oxalate ions. Geochimica et Cosmochimica Acta, 51(3), 597–605. doi:10.1016/0016-7037(87)90072-X
- Cantrell, K. J., & Byrne, R. H. (1987b). Temperature dependence of europium carbonate complexation. Journal of Solution Chemistry, 16(7), 555–566. doi:10.1007/BF00646333
- Cheremisina, O., Cheremisina, E., Ponomareva, M., & Fedorov, А. (2020). Sorption of rare earth coordination compounds. Journal of Mining Institute, 244, 474–481. doi:10.31897/pmi.2020.4.10
- Cheremisina, O. V., Schenk, J., Cheremisina, E.A., & Ponomareva, M.A. (2019). Thermodynamic model of ion-exchange process as exemplified by cerium sorption from multisalt solutions. Journal of Mining Institute, 237(3), 307–316. doi:10.31897/pmi.2019.3.307
- Choppin, G. R. (1997). Factors in Ln(III) complexation. Journal of Alloys and Compounds., 249(1-2), 1–8. doi:10.1016/S0925-8388(96)03016-2
- Choppin, G. R., & Graffeo, A. J. (1965). Complexes of trivalent lanthanide and actinide ions. II. Inner-sphere complexes. Inorganic Chemistry, 4(9), 1254–1257. doi:10.1021/ic50031a004
- Dezhi, Q. (Ed.). (2018). Hydrometallurgy of rare earths. pp. 1–185; Elsevier.
- Fialkovsky, I.S. (2020a). Study of the distribution of equilibrium forms of cerium and ytterbium in solutions of complex water-salt composition. Nat. Tech. Sci, 144, 38–46.
- Fialkovsky, I.S. (2020b). Physicochemical study of the effect of the halide ion on the extraction of cerium (III), europium (III) and gadolinium (III) from nitrate media with naphthenic acid. Nat. Tech. Sci, 140, 40–46.
- Glushko, V.P. (2004). Thermodynamic properties of simple substances. Moscow: Publishing House “Science”.
- Innocenzi, V., Ippolito, N.M., Pietrelli, L., Centofanti, M., Piga, L., & Vegliò, F. (2018). Application of solvent extraction operation to recover rare earths from fluorescent lamps. Journal of Cleaner Production, 172, 2840–2852. doi:10.1016/j.jclepro.2017.11.129
- Jha, M.K., Kumari, A., Panda, R., Rajesh Kumar, J., Yoo, K., & Lee, J.Y. (2016). Review on hydrometallurgical recovery of rare earth metals. Hydrometallurgy, 165, 2–26. doi:10.1016/j.hydromet.2016.01.035
- Kurdiumov, V., Timofeev, K., Maltsev, G., & Lebed, A. (2020). Sorption of nickel (II) and manganese (II) ions from aqueous solutions. Journal of Mining Institute, 242, 209. doi:10.31897/pmi.2020.2.209
- Lee, J.H., & Byrne, R.H. (1992). Examination of comparative rare earth element complexation behavior using linear free-energy relationships. Geochimica et Cosmochimica Acta, 56(3), 1127–1137. doi:10.1016/0016-7037(92)90050-S
- Lobacheva, O., & Dzhevaga, N. (2017). Rare-earth elements recovery on the example of Europium (III) from lean technogenic raw materials. Journal of Ecological Engineering, 18(6), 122–126. doi:10.12911/22998993/76827
- Lobacheva, O., & Dzhevaga, N. (2020). Method for removing valuable components from technogenic solutions by the example of rare earth elements. Journal of Physics: Conference Series, 1679(4), 042016. in: . doi:10.1088/1742-6596/1679/4/042016
- Lucas, J., Lucas, P., Le Mercier, T., Rollat, A., & Davenport, W. (2015). Rare earth electronic structures and trends in properties. In Rare earths (pp. 123–139). Elsevier. doi:10.1016/B978-0-444-62735-3.00008-5
- Lugina, L.N., & Davidenko, N.A. (1977). Study of the composition and structure of europium aqua complexes by electron spectroscopy. Journal of Coordination Chemistry, 3, 190–196.
- McDowell, W.J., & Coleman, C.F. (1972). The sulfate complexes of some trivalent transplutonium actinides and europium. Journal of Inorganic and Nuclear Chemistry, 34(9), 2837–2850. doi:10.1016/0022-1902(72)80590-6
- Menon, M.P. (1987). Studies on the solubility and complexation of lanthanum and neodymium fluoride-water systems. Lanthanide and Actinide Research, 19(11), 49–66.
- Migdisov, A., Williams-Jones, A.E., Brugger, J., & Caporuscio, F.A. (2016). Hydrothermal transport, deposition, and fractionation of the REE: Experimental data and thermodynamic calculations. Chemical Geology., 439, 13–42. doi:10.1016/j.chemgeo.2016.06.005
- Moulin, N., Hussonnois, M., Brillard, L., & Guillaumont, R. (1975). Fonctions thermodynamiques de complexes halogénés de Sm, Eu, Gd, Tb et Dy. Journal of Inorganic and Nuclear Chemistry., 37(12), 2521–2524. doi:10.1016/0022-1902(75)80884-0
- Omodara, L., Pitkäaho, S., Turpeinen, E.-M., Saavalainen, P., Oravisjärvi, K., & Keiski, R.L. (2019). Recycling and substitution of light rare earth elements, cerium, lanthanum, neodymium, and praseodymium from end-of-life applications - A review. Journal of Cleaner Production, 236, 117573. doi:10.1016/j.jclepro.2019.07.048
- Pearson, R.G. (1988). Absolute electronegativity and hardness: Application to inorganic chemistry. Inorganic Chemistry, 27(4), 734–740. doi:10.1021/ic00277a030
- Persson, I. (2010). Hydrated metal ions in aqueous solution: How regular are their structures? Pure and Applied Chemistry, 82(10), 1901–1917. doi:10.1351/PAC-CON-09-10-22
- Persson, I., D'Angelo, P., De Panfilis, S., Sandström, M., & Eriksson, L. (2008). Hydration of lanthanoid(III) ions in aqueous solution and crystalline hydrates studied by EXAFS spectroscopy and crystallography: The myth of the “Gadolinium break". Chemistry (Weinheim an Der Bergstrasse, Germany), 14(10), 3056–3066. doi:10.1002/chem.200701281
- Peppard, D.F., Mason, G.W., & Hucher, I. (1962). Stability constants of certain lanthanide(III) and actinide(III) chloride and nitrate complexes. Journal of Inorganic and Nuclear Chemistry, 24(7), 881–888. doi:10.1016/0022-1902(62)80109-2
- Pouillon, D., & Doyle, F.M. (1988). Solvent extraction of metals with carboxylic acids — Theoretical analysis of extraction behaviour. Hydrometallurgy, 19(3), 269–288. doi:10.1016/0304-386X(88)90035-7
- Qi, D. (2018). Extractants used in solvent extraction-separation of rare earths: Extraction mechanism, properties, and features. In Hydrometallurgy of rare earths (pp. 187–389). Elsevier. doi:10.1016/B978-0-12-813920-2.00002-7
- Rao, V.K. (1970). Studies on the phosphate complexes of actinium and lanthanum. Radiochimica Acta, 19, 31–34.
- Savchenkov, S. A., Bazhin, V. Y., & Povarov, V. G. (2020). Research on the process of gadolinium recovery from the melt of salts on formation of Mg-Zn-Gd master alloys for manufacturing of magnesium and aluminium spesial-purpose alloys. Non-Ferrous Metals, 1, 35–40. doi:10.17580/nfm.2020.01.06
- Savelyeva, I.L. (2011). Rare-earth industry of Russia: Current state, resource development conditions. Geography and Natural Resources, 1, 122–129.
- Sekine, T., Nord, S., Sjöberg, B., Mellander, O., & Hinton, M. (1965). Solvent extraction study of trivalent actinide and lanthanide complexes in aqueous solutions. I. Chloride complexes of La(III), Eu(III), Lu(III), and Am(III) in 4 M Na(ClO4)/T. Acta Chemica Scandinavica, 19, 1435–1444. doi:10.3891/acta.chem.scand.19-1435
- Siziakova, E. V., & Ivanov, P. V. (2020). On the role of hydrated calcium carboaluminate in the improvement of the production technology of alumina from nephelines. Journal of Physics: Conference Series, 1515(2), 022048. doi:10.1088/1742-6596/1515/2/022048
- Siziakova, E. V., Ivanov, P. V., & Nikitina, T.Y. (2019). Effect of coal-containing additives on the rheological properties of limestone-nepheline charge in the technology of alumina production, in. Journal of Physics: Conference Series, 1384(1), 012048. doi:10.1088/1742-6596/1384/1/012048
- Spahiu, K., & Bruno, J. (1995). A selected thermodynamic database for REE to be used in HLNW performance assessment exercises. SKB TR 95-35, Svensk Kärnbränslehantering AB.
- Tanaka, F., & Yamashita, S. (1984). Luminescence lifetimes of aqueous europium chloride, nitrate, sulfate, and perchlorate solutions. Studies on the nature of the inner coordination sphere of europium(III) ion. Inorganic Chemistry, 23(14), 2044–2046. doi:10.1021/ic00182a013
- Voropanova, L. (2016). The extraction of silver ions from hydrochloric acid solutions of TBP. Journal of Mining Institute , 218, 220–224.
- Voropanova, L. A., & Pukhova, V. P. (2018). Extraction of copper, cobalt and nickel ions from aqueous solutions by extractant cyanex. Journal of Mining Institute, 233, 498–505. doi:10.31897/PMI.2018.5.498
- Walker, J.B. (1967). Thermodynamic parameters of fluoride complexes of the lanthanides. Advanced Chemistry, 71, 127–140.
- Wood, S.A. (1990). The aqueous geochemistry of the rare-earth elements and yttrium. Chemical Geology, 82, 159–186. doi:10.1016/0009-2541(90)90080-Q
- Xie, F., Zhang, T.A., Dreisinger, D., & Doyle, F. (2014). A critical review on solvent extraction of rare earths from aqueous solutions. Minerals Engineering., 56, 10–28. doi:10.1016/j.mineng.2013.10.021