Abstract
Tramadol is a widely prescribed analgesic for pain management in adults, but its safety during pregnancy remains a subject of concern. While previous research has highlighted potential risks associated with Tramadol use during gestation, there is a need for a comprehensive investigation into its effects on foetal brain development. This preclinical study sought to address this gap in knowledge by examining the impact of Tramadol exposure on the cerebral and cerebellar cortices in a murine model. Pregnant mice were subjected to controlled exposure to varying doses of Tramadol during the initial stages of gestation. The foetal brains were examined through a combination of histopathological analysis, assessment of apoptotic changes, and evaluation of amyloid precursor protein (APP) expression. These investigative methods allowed for a detailed examination of structural alterations, cell death patterns, and molecular changes within the foetal brain tissues. The study’s findings were notable for several significant observations. Firstly, there was clear evidence of global shrinkage within the cerebral and cerebellar cortices of foetuses exposed to Tramadol. This shrinkage was associated with disrupted cellular organization, marked apoptosis of neural cells, and a notable upregulation in the expression of APP, particularly within the cerebral cortex. Specifically, a 23% reduction in cerebral cortex size was observed in Tramadol-exposed foetuses compared to the control group. Furthermore, a dose-dependent relationship was established, indicating that higher Tramadol doses corresponded to more pronounced alterations in foetal brain structure and molecular profile. Prenatal tramadol treatment induces neurodegenerative changes in newborn cerebellum with agenesis in the Purkinje cells layer and up regulation of APP in response to the neurotoxic effect that could be considered a neuroprotective role for neurons.
1. Introduction
Opioid exploration of the human body’s multifaceted effects, particularly through the perspective of tramadol’s pharmacological profile, is important in neuroscience and pharmacotherapy (Dhesi et al., Citation2023). Tramadol is an analgesic that acts centrally and has been used as part of the medical armamentarium for pain management since it was introduced more than three decades ago; this statement does not exaggerate its importance (Dhesi et al., Citation2023). The physiological function of tramadol is unique; it activates opioid receptors while at the same time inhibiting reuptake of neurotransmitters like serotonin and catecholamines thereby qualifying it as a compound whose therapeutic benefits are worth studying as well as potential neurological implications (Dhesi et al., Citation2023; Zebala et al., Citation2019).
Tramadol relieves patients’ pain by modulating its pathway via opiate receptors (ORs) and affecting synaptic transmissions involved in understanding pain information across multiple clinical settings that might be relevant to diverse nociceptive stimuli (Edinoff et al., Citation2021). However, these very mechanisms underlying its effectiveness in relieving pain also result in interactions within the Central Nervous System (CNS) which extend beyond simple analgesia. This includes reduced reuptakes of serotonin and catecholamine implying influence on mood, anxiety and cognitive processes involving these neurotransmitters’ control functions (Dhesi et al., Citation2023; Zebala et al., Citation2019).
The worries regarding tramadol are with long term use and have been a subject of continuous study and discussion. A conspicuous case of interest in this area is the possibility that it can cause structural changes in neurons of the brain. Such modifications may be very important for neural function as well as plasticity, which might lead to cognitive deficiencies or alterations in mood and behaviour (Ahmadimanesh et al., Citation2020). Furthermore, addiction risk is another caveat considered by prescribers when prescribing opioids due to the fact that it has led to tramadol being used carefully while monitoring closely its clinical practice (Ahmadimanesh et al., Citation2020).
Of great concern is the impact of Tramadol during pregnancy, where it has been shown to cause temporary withdrawal symptoms in newborns associated with neonatal adaptation syndrome and increased chance of miscarriage. These findings necessitate a re-assessment of the safety profile for tramadol within particular patient populations and accentuate the importance of strict evidence-based guidelines for its use (Ahmadimanesh et al., Citation2020).
Given these, it is important to look further into the bigger biological perspectives of tramadol. A comprehensive knowledge of how tramadol interacts with nerve pathways and proteins is required not only to mitigate possible side effects but also for maximum not limited to, effects on neurotransmitter systems, neural plasticity as well as expression and functionality of major proteins implicated in neural health and illness (Grond & Sablotzki, Citation2004). The scientific community can therefore unravel all intricacies pertaining to this drug’s effect towards CNS which will help us strike a balance between optimally using it for pain relief while minimizing risks associated with it usage. This research exercise will additionally contribute towards our understanding of the pharmacodynamics of tramadol and provide valuable information about other effects that opioids have on neurological functions and diseases.
Central to this is the amyloid precursor protein (APP), which is one of the most important molecules investigated in neurodegenerative diseases, especially Alzheimer’s disease (AD), being at the centre of this investigation. APP is a one-pass transmembrane protein which plays a critical part in the development of amyloid plaques that are typical to AD pathology (Zheng & Koo, Citation2011). Its divergent extracellular domain, transmembrane segment and small intracellular domain are created as a result of alternative splicing processes of its gene located on chromosome 21. This results into three major isoforms, viz: APP695 found primarily in neurons and ubiquitously expressed APP751 and APP770 (Liu et al., Citation2021; Nguyen, Citation2019). The extracellular domain of APP exhibits 6 different subdomains with distinct molecular structure and discrete binding properties. Some of these domains facilitate interactions such as those with ECM proteins while others can bind metals or other regulatory factors thus indicating various roles played by APP within cells (Nguyen, Citation2019).
The external domain of the Amyloid Precursor Protein (APP) is composed of up to six unique subdomains, each featuring distinct structures and specific binding functions. This includes the ability to interact with proteins in the extracellular matrix, various metals, and proteins that play regulatory roles (Nguyen, Citation2019). Unique to APP within its protein family is the Aβ sequence, which is not conserved in its homologs, APLP1 and APLP2. This aspect is particularly significant in the context of APP's implication in AD through the formation of amyloid plaques (Liu et al., Citation2021; Nguyen, Citation2019; Zheng & Koo, Citation2011). Despite the unique Aβ sequence, the high sequence identity among the mammalian homologs of APP suggests they may have overlapping functions. The evolutionary conservation of these proteins, including in mammals highlights their significant role in physiological processes (Liu et al., Citation2021; Nguyen, Citation2019; Zheng & Koo, Citation2011).
After being produced in the endoplasmic reticulum, the Amyloid Precursor Protein (APP) which undertakes modifications following translation within the Golgi apparatus (O'Brien & Wong, Citation2011). Following these modifications, the APP is transported to the cell membrane, where it is acted upon by different membrane-associated proteolytic enzymes. This processing involves an initial cleavage by γ-secretase in the transmembrane domain, and then by α-secretase. These steps result in the production of several fragments, including the amyloid β (Ab) peptide, known for its association with neurotoxicity (Haass et al., Citation2012). Under normal physiological conditions, only a minor portion of APP is secreted, with α-secretase-mediated cleavage being more prevalent (Hick et al., Citation2015). The expression, trafficking, and processing of APP are tightly controlled under normal conditions. However, these processes experience substantial alterations during pathological states, including metabolic stress, ischaemia, brain injury, and inflammation (Guedes-Dias & Holzbaur, Citation2019; Liu et al., Citation2021; Nguyen, Citation2019). APP's interaction with different proteins, essential for preserving cell integrity under stress, indicates a dual nature in its outcomes post-cleavage, encompassing both protective and potentially harmful effects. This dual role of APP presents promising avenues for new therapeutic strategies in treating disorders such as stroke, Alzheimer’s Disease (AD), and traumatic brain injury (TBI) (Le Bras, Citation2021).
This study aims to investigate the doses effects of Tramadol exposure during the perinatal period on the development of the cerebral and cerebellar cortices in newborns and to understand its influence on the expression of amyloid precursor proteins. This research aims to deepen our understanding of the interaction between opioid analgesics and neurodevelopmental processes, particularly in the context of Alzheimer’s disease and other neurodegenerative conditions.
2. Materials and methods
2.1. Animal sample
This research received approval from the Animal Research Ethics, College of Medicine, Al-Nahrain University. Sixty adult female mice (Mus Musculus) were housed at the High Institute of Diagnosis and Assisted Reproductive Technology (HIDART) at Al-Nahrain University, Baghdad, Iraq. These mice were aged between 8-12 wk, weighed 20-30 g, and were kept in controlled environmental conditions with a 14:10 h light-dark cycle at a temperature of 22 ± 2 °C. They were housed in plastic cages, 2-3 animals per cage, with free access to a standard pellet diet and fresh tap water.
2.2. Animal mating and pregnancy documentation
For mating, each cage contained one male and two to three female mice. Mating typically occurred at night, with pregnancy confirmed by checking for the presence of a vaginal plug.
2.3. Sample Grouping
After fertilization, the pregnant mice were separated to indicate the commencement of pregnancy. These sixty pregnant mice were subsequently divided into four groups, each consisting of fifteen mice. The experimental groups, labelled A, B, and C, were administered Tramadol doses of 40, 60, and 80 mg/kg intraperitoneally, respectively. Meanwhile, the control group was given distilled water (D.W.) intraperitoneally.
The determination of the total animals and their allocations per group was guided by statistical and ethical considerations. The chosen sample size of 15 mice per group was designed to ensure statistical power sufficient to detect significant differences between the control and treated groups while minimizing the use of animal subjects in accordance with ethical research practices. This sample size reflects a balance between the need for reliable data and the imperative to adhere to the principles of the 3Rs (Replacement, Reduction, and Refinement) in animal research. By maintaining equal group sizes, the study aimed to provide a robust framework for assessing the effects of varying tramadol doses on pregnancy outcomes, ensuring that the findings are both scientifically valid and ethically sound.
2.4. Tramadol treatment
Tramadol® (HAVER© 100 mg/2 ml) was diluted in distilled water and administered daily intraperitoneally (IP). The doses were calculated based on animal weight, following the guidelines provided by Shamsi Meymandi & Keyhanfar, 2013, for optimal antinociceptive effects in mice (Shamsi Meymandi & Keyhanfar, Citation2013).
2.5. Animal dissection
Each female mouse delivered (2–6) newborns, two newborns per female mouse were selected, meaning that the total number of newborns were 120. Newborns were weighed post-delivery using a sensitive balance. Newborn mice were euthanized using chloroform chamber. Following death, their heads were dissected sagittal into two halves and prepared for histological analysis of the cerebral and cerebellar cortices.
2.6. Histological tissue preparation
This process included multiple steps outlined below (Perry et al., Citation2016):
The preparation process for the specimens involved several meticulously optimized steps to ensure the integrity and quality of the samples for histological examination. Initially, each half of the dissected head was fixed in 10% neutral buffered formaldehyde for 36 h, a duration determined as optimal through preliminary trial assessments. Following fixation, the specimens were dehydrated through an ascending series of ethanol concentrations, starting with 70% ethanol for 24 h, followed by 90% ethanol for 6 h, and culminating with 100% ethanol for 3 h to ensure complete dehydration. Subsequently, the dehydrated specimens underwent clearing in xylene for 30 min, with the solution being changed twice to guarantee thorough clearing of all ethanol traces. After clearing, the specimens were infiltrated with melted paraffin wax at 58 °C in two stages, each lasting 2 h, to prepare them for embedding. The paraffin-infiltrated specimens were then carefully positioned within L-shaped metal moulds filled with melted paraffin, ensuring the mid-sagittal section was correctly oriented to face forward. Once set, the paraffin blocks were labelled and stored at 4 °C for preservation. Serial sections of 5 µm thickness, were cut from the sagittal side using an electrical Reichert-Jung 2030 Mot Biocut microtome for histopathological examination. These sections were then floated in a water bath before being mounted on glass slides for Haematoxylin and Eosin (H&E) staining and on positively charged slides for immunohistochemical staining, ensuring the samples were prepared for detailed microscopic examination.
2.7. Staining (Horobin, Citation2013)
Harris-Alum Haematoxylin Stain: The staining solution was prepared according to the procedure detailed by Survana & Bancroft, involving dissolving haematoxylin in absolute ethyl alcohol, adding potassium alum dissolved in warm distilled water, rapid boiling with mercuric oxide, cooling, and adding glacial acetic acid. The stain was stored and filtered before use (Bancroft & Layton, Citation2013).
Eosin: This counterstain to haematoxylin was prepared by dissolving eosin powder in 70% ethyl alcohol and adding glacial acetic acid.
Haematoxylin and Eosin Staining: The detailed procedure involves deparaffinization in xylene, rehydration in descending concentrations of ethanol, staining with Harris Alum Haematoxylin, counterstaining with eosin, dehydration, clearing in xylene, and mounting with Bukit mounting media.
Cresyl Violet Staining (Nissl stain): The slides were dewaxed, rehydrated, stained with cresyl violet, rinsed, dehydrated, and processed further as per the specified reference.
2.8. Immunohistochemistry for amyloid precursor protein antibody ab15272
In this study, a diverse range of APP-related antibodies was employed to investigate Amyloid Precursor Protein pathways, including rabbit monoclonal APP Y188 from Epitomics at 1:500, and mouse monoclonal APP A8967 from Sigma at dilutions of 1:100 to 1:1000. Additionally, rabbit polyclonal APP ab15272 from Abcam, and a laboratory-generated rabbit polyclonal antibody, APPc, were used within the same dilution range. Other key antibodies included mouse monoclonals such as 22C11, 4G8, and APP SIG-39184, all utilized at 1:100 to 1:1000 dilutions to ensure detailed and nuanced detection of APP and its variants. This comprehensive selection of primary antibodies, alongside secondary antibodies, and markers for neuronal identification, underscores the meticulous approach taken to elucidate APP's intricate roles in neural contexts (Guo et al., Citation2012; Richter et al., Citation2018).
For controls, the study incorporated both positive and negative benchmarks to validate the staining protocols. The positive control comprised spinal cord sections from the control group, which, when stained for amyloid precursor protein, exhibited positive staining with DAB, confirming the expected presence of APP. Conversely, the negative control involved sections from the treated groups, processed identically but without the application of the primary antibody, ensuring the specificity of staining and antibody binding.
2.9. Image analysis
2.9.1. Aperio Scope Image Analysis Software (version 9)
This software was used for quantifying specific stains in scanned slide images. The positive pixel count algorithm analyzed stained pixels in terms of hue, saturation, and intensity. Results included various metrics like the number of positive pixels and their intensity values.
2.10. Statistical analysis
Statistical analyses were performed using SPSS version 24. ANOVA single factor analysis was utilized for comparing groups, with a p-value of ≤0.05 considered statistically significant. Unpaired t-tests were employed for comparing groups when significant differences were detected.
2.11. Photography and scaling
Photographic documentation of the images was conducted using a Sony Cyber-shot camera at magnifications of 10X, 40X, and 100X. The ImageJ program was used for applying scale bars to the images.
3. Results
3.1. Macroscopic changes in newborn mice
There was a significant increase in the mean body weight for the mice in the three study groups (Group A: 1.65 ± 0.06, Group B: 1.45 ± 0.23, Group C: 1.07 ± 0.26, grams), when compared to the control group (0.98 ± 0.48, grams), (p ≤ 0.05). This was most notable in Group A and least in Group C.
3.2. Histological changes in cerebral and cerebellar cortices
In the cerebral cortex of the study groups, Haematoxylin and Eosin (H&E) staining revealed marked atrophy, characterized by a significant reduction in size and the loss of the gyri and sulci pattern (). This was accompanied by an expanded subarachnoid space (). Comparatively, the control group exhibited no atrophic changes and shallower sulci (). The three study groups displayed various features of apoptotic nuclear changes in the cerebral cortex, such as blebbing, cytoplasmic degeneration, (), nuclear fragmentation, (), cellular pyknosis, (, and macrophage activity to engulf apoptotic bodies ().
Figure 1. General histological changes of the cerebral and cerebellar cortices in the three study groups as compared to the control group (using Eosin and Haematoxylin stain). A: Study group a shows cerebral cortex atrophy: expanded subarachnoid space, smooth surface (10X). B: Control group: normal cerebral cortex morphology, shallow sulci (10X). C-E: Study group a displays apoptosis in the cerebral cortex: blebbing, cytoplasmic degeneration, pyknosis, nuclear fragmentation, macrophage infiltration, indicated by red (blebbing, fragmentation), black (pyknosis), and yellow arrows (macrophages) (40X, 100X). F: Study group B: cerebral cortex apoptosis with nuclear pyknosis (black arrow) (100X). G: Study group C: cerebral cortex apoptosis, nuclear pyknosis, cytoplasmic vacuolation, reduced cellularity (100X).
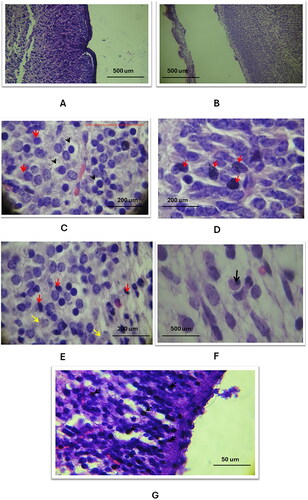
Additionally, the cerebral cortical blood vessels showed dilation and rupture. Apoptotic changes, including cytoplasmic degeneration, nuclear fragmentation, and the presence of macrophages, were detailed ().
Figure 2. The general histological changes of the cerebral blood vessels in the three study groups as compared to the control group (using Eosin and Haematoxylin stain). A: the control group exhibited normal histology of the capillary blood vessel (20X magnification), B: in study group A, there was a dilation of the capillary vessel in the cerebral cortex, as indicated by the arrow (20X magnification), C: in study group C, a ruptured blood vessel in the cerebral cortex was observed, with red blood cells (RBCs) escaping into the cortex (100X magnification).
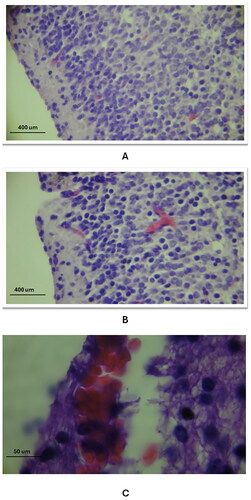
Further, in study group A, the molecular layer exhibited a reduced number of nuclei and vacuolation in the cortex parenchyma, alongside altered shapes of pyramidal cells in the cerebral cortex’s second layer (). Study group B showed distorted pyramidal cells with reduced cell volume and dense pyknosis of nuclei (). In study group C, the inner layer of the cortex had apoptotic nuclei with cytoplasmic degeneration and vacuolation ().
Figure 3. Histological changes of the cellular layers of the cerebral cortex among the three study groups as compared to the control group (using Eosin and Haematoxylin stain). A: Study group A: Abnormal cell shapes in the molecular layer (1) and external granular and pyramidal layer (2) (40X). B: Study group B: Pyramidal cell layer defects, reduced cell volume (red arrows), and apoptotic cells (black arrows) (100X). C: Study group C: Inner layer defects with numerous apoptotic cells and lost parenchymal structure (40X).
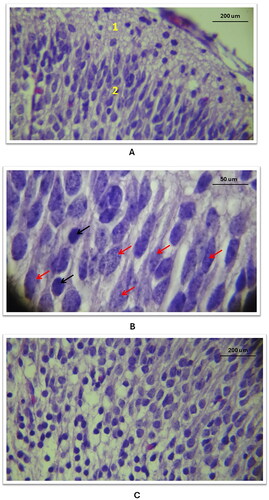
In the cerebellar cortex, similar histological changes were observed, such as atrophy with an expanded subarachnoid space, dilation of the 4th ventricle, absence of Purkinje cells, and localized haemorrhages ().
Figure 4. Histological changes of the cellular layers of the cerebellar cortex among the three study groups as compared to the control group (using Eosin and Haematoxylin stain). A: Control group: Normal folia and sulci, proper cerebellum and choroid plexus placement in the skull and 4th ventricle (10X). B: Study group A: Cerebellar atrophy with expanded subarachnoid space, shallow folia and sulci, dilated 4th ventricle, reduced choroid plexus (10X). C: Control group: All three cellular layers visible - A: molecular, B: Purkinje cells, C: granular (40X). D: Study group B: Missing Purkinje cells (yellow arrow), haemorrhage in granular layer (black arrow), apoptotic nuclei in molecular layer (40X). E: Study group C: Ventricular cavity with red blood cells (black arrow), decreased arborisation of choroid plexus villi (40X).
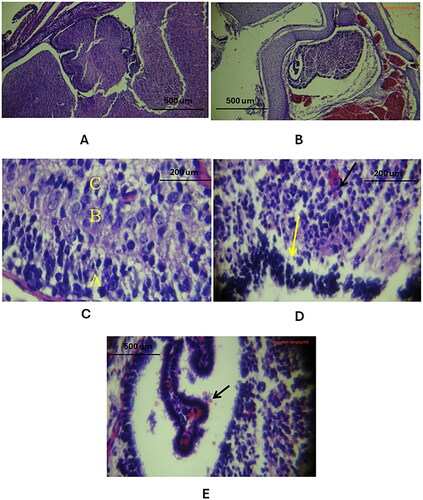
3.3. Cresyl violet staining to the brain tissues
3.3.1. Cerebral cortex changes
Tramadol injection induced the following changes in the layers of the cerebral cortex:
Layering Pattern Defects: The histology of the cerebral cortex in the control group showed a normal pentralaminar pattern (), while the three study groups showed a disrupted layering pattern with apparent separation between layers and atrophic changes (). These alterations were more pronounced with the higher tramadol doses.
Nuclear Pattern Alterations: In contrast to the control group’s normal vesicular nuclei with prominent nucleoli (), the three study groups exhibited dense, smaller-sized nuclei without prominent nucleoli. The extent of these changes was dose-dependent (.
Severe Changes with High-Dose Tramadol: In Group C, which received a high tramadol dose, there was marked nuclear pyknosis (.) This was coupled with a loss of the normal shape of neurons and cytoplasmic vacuolation, leading to a disrupted parenchymal structure of the cortex (). A phenomenon not observed in the control group, ().
Figure 5. General histological changes of the cerebral cortex in the three study groups as compared to the control group (using Cresyl Violet stain). A: in the control group, a histological section of the cerebral cortex displayed the penta-laminar pattern of cerebral cortex layers (10X magnification), B: in study group A, a section revealed a defect in the laminar structure and the presence of spaces between layers, indicated by red stars (10X magnification); C: Study group B exhibited a defect in the layering pattern, as shown by black arrows, and atrophic changes characterized by an enlarged subarachnoid space, marked with a star shape (10X magnification).
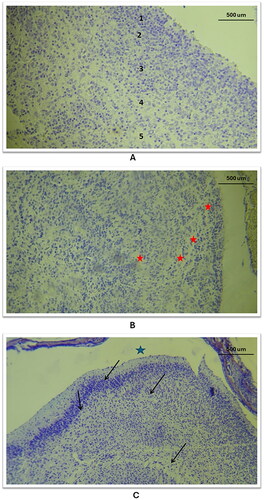
Figure 6. Histological changes of the outer and inner layers of the cerebral cortex in the three study groups as compared to the control group (using Cresyl Violet stain). A/B: Control group: Outer and inner layers show neurons with normal, vesicular nuclei and prominent nucleoli (40X). C: Study group A, outer layer: Neuronal nuclei shape altered and intensely stained (40X). D: Study group B, outer layer: Neuronal cell shape alterations in the 2nd layer (red curve) (40X). E: Study group C, outer layer: Nuclear size reduction, darkly stained nuclei without nucleoli, and atrophy (wide subarachnoid space marked with star) (10X). F: Study group C, inner layer: Significant nuclear and tissue pattern changes (10X).
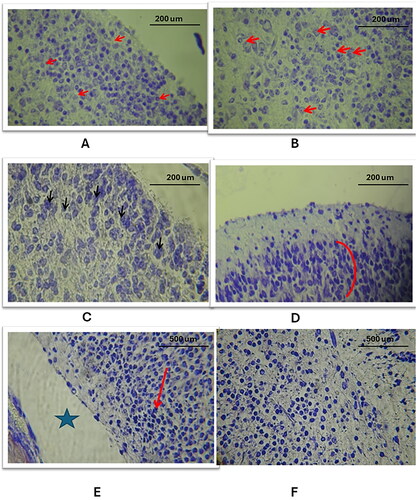
3.3.2. Cerebellar cortex changes
Cortical Atrophy: The cerebellar cortex in the study groups showed significant atrophy as elated to the control cohort, (, , respectively).
Purkinje Cells Layer Defects: There was a notable defect in the Purkinje cells layer in the study groups, with an absence of these cells (), compared to the control group which exhibited a normal trilaminar cortex ().
Nuclear Pattern Changes: The nuclei in the cerebellar cortex of the study groups appeared smaller and darker () than those in the control group ().
Parenchymal Tissue Pattern Alterations: The study groups exhibited a less compact cortical structure, indicating changes in the overall parenchymal tissue pattern (), as related to the control cohort ().
Figure 7. Histological changes of the outer and inner layers of the cerebellar cortex in the three study groups as compared to the control group (using Cresyl Violet stain). A. A/B: Control group: Normal cerebellar cortex, trilaminar structure visible with choroid plexus in 4th ventricle, layers: 1. Molecular, 2. Purkinje cells, 3. Granular (10X, 40X). C: Study group A: Cerebellar cortex atrophy, reduced size, expanded subarachnoid space (10X). D/E: Study group a and B: Loss of Purkinje cells layer, nuclear and parenchymal changes (red arrow) (40X). F/G: Study group C: Pronounced Purkinje cells absence, cortex parenchymal defects, significant nuclear and parenchymal alterations (black arrows) (10X, 40X).
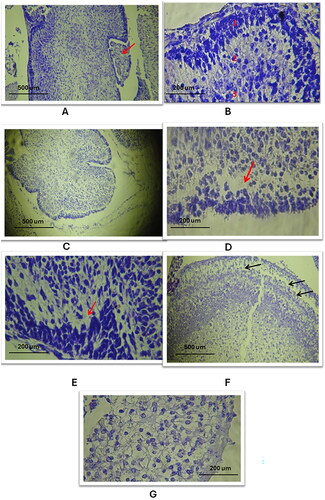
3.3.3. Immunohistochemistry for Amyloid Precursor Protein antibody ab15272:
The staining results using the Anti-Amyloid Precursor Protein antibody ab15272 revealed significant data in both the cerebrum and cerebellum (), showing distinct variations between the control cohort and tramadol treated cohorts, as detailed in (p < 0.01). Specifically, in the cerebrum, there was a statistically significant elevation in amyloid precursor protein expression correlated with increasing tramadol dosages, as indicated in (p < 0.001). A parallel pattern was observed in the cerebellum, where expression levels noticeably varied across the groups, again noted in (p < 0.001). These findings imply a dose-responsive effect of tramadol on amyloid precursor protein expression within these brain areas, highlighting the drug’s potential influence on neural biochemistry.
Figure 8. The levels of anti-amyloid precursor protein antibody ab15272 in the cerebrum and cerebellum across the four study groups., p < 0.001.
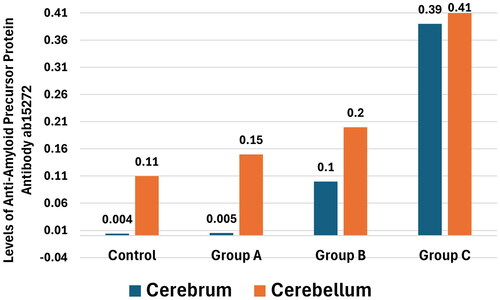
Table 1. Comparison of anti-Amyloid Precursor Protein antibody ab15272 in cerebrum and cerebellum, among the four groups of study.
4. Discussion
Opioid medications, including Tramadol, are commonly prescribed during pregnancy for a range of painful conditions such as low back and pelvic pain (Dave et al., Citation2019; Yazdy et al., Citation2015). However, the use of Tramadol in pregnancy is often avoided due to limited safety data and concerns about its potential effects on the foetus (Dave et al., Citation2019; Yazdy et al., Citation2015). This study explored these effects, specifically focusing on Tramadol’s impact on the development of the cerebral and cerebellar cortices in newborn mice.
Our results demonstrated significant changes in the body weight of newborns exposed to Tramadol, with a marked increase in body weight observed. Interestingly, this increase was inversely related to the dosage of Tramadol administered, suggesting that higher doses might adversely affect foetal development. This could be attributed to Tramadol crossing the placental barrier, potentially impacting the metabolic pathways in the foetal liver where it is metabolised. Such effects might include alterations in organ development due to the concentrated presence of Tramadol in the foetal circulation, especially at higher doses (Dhesi et al., Citation2023).
Previous research on opioid use in pregnancy and its effects on birth weight has yielded mixed results. Some studies have linked opioid use with low birth weight or small for gestational age neonates (Yazdy et al., Citation2015), while others have reported no such associations or even found increased rates of large for gestational age births (Specialists, Citation2021). This inconsistency in findings highlights the complexity of assessing the impact of opioids on foetal development and underscores the need for further research in this area. In light of this, our study specifically focuses on the cerebral and cerebellar cortices as target structures for analysis, given their critical roles in cognitive and motor functions and their potential vulnerability to prenatal opioid exposure. This choice aims to shed light on specific neurodevelopmental outcomes that may be affected by Tramadol use during pregnancy, thus providing more targeted insights into its effects on foetal brain development, an area that has not been extensively studied. The findings suggest that while Tramadol use during pregnancy may impact on foetal growth, the exact nature and extent of these effects, particularly on the development of the cerebral and cerebellar cortices, warrant further investigation.
Moreover, in our study, tramadol was administered IP following the protocol outlined by Shamsi Meymandi & Keyhanfar, 2013, for achieving optimal antinociceptive effects in mice (Shamsi Meymandi & Keyhanfar, Citation2013). This route was chosen over the common oral or intravenous routes used clinically for its advantages in ensuring precise dosage, rapid absorption, and consistent systemic exposure. IP administration allows for direct delivery into the systemic circulation, bypassing the first-pass metabolism and facilitating accurate assessment of Tramadol’s pharmacological effects on foetal development.
4.1. Atrophic shifts in the cerebral cortex and cerebellar cortex
In our study, we observed pronounced atrophic changes in the cerebral and cerebellar cortices of neonates following Tramadol exposure, characterized by significant shrinkage and a noticeable widening of the subarachnoid space. These changes are indicative of cellular atrophy, often stemming from a loss of cytoplasmic proteins and neural connections (Ghoneim et al., Citation2014). This phenomenon in brain tissue is typically linked to neuronal loss, potentially triggered by apoptosis due to Tramadol treatment. Supporting this, Ghoneim et al., Citation2014, highlighted that Tramadol induces cerebral cortex histological abnormalities linked to oxidative stress, leading to an imbalance in the oxidant/antioxidant ratio and cellular apoptosis (Ghoneim et al., Citation2014). Further, the long-term use of opioids is known to cause significant alterations in brain morphology, connectivity, and function (Corbett et al., Citation2019; Els et al., Citation2017; Sullivan & Ballantyne, Citation2022). Specifically, our findings of a smoother brain surface and disrupted cerebellar cortex pattern in study groups suggest an impairment in cortical neurogenesis due to Tramadol’s neurotoxic effects (Corbett et al., Citation2019; Els et al., Citation2017; Sullivan & Ballantyne, Citation2022). Sowing a consistent with the concept of Lissencephaly ('smooth brain’) described by Mochida, et al. in 2013, where improper neuronal migration and cortical folding lead to a lack of normal brain surface features (Mochida et al., Citation2013). However, there is a paucity of studies linking Tramadol to these specific cerebellar cortex changes. Additionally, our study’s findings resonate with previous research which indicated a connection between opioid use, including codeine, during pregnancy and the occurrence of specific neural tube defects (NTDs) such as spina bifida (Bateman et al., Citation2021; Fishman et al., Citation2019).
4.2. Apoptotic changes in cerebral cortex
In the cerebral cortex of neonates exposed to Tramadol, a range of apoptotic changes where observed, including cellular blebbing, cytoplasmic vacuolation, nuclear fragmentation, and pyknosis. These findings are in line with previous research by Asahi et al. (Citation2017) and Tang et al. (Citation2019), who reported similar neuronal apoptosis and chromatolysis following Tramadol administration (Asahi et al., Citation2017; Tang et al., Citation2019). The mechanism underlying these changes can be linked to Tramadol’s ability to damage cellular structures, initiating apoptosis through both intrinsic and extrinsic pathways (Asahi et al., Citation2017; Tang et al., Citation2019). This finding emphasized the role of neurotoxins in triggering autophagy (Asahi et al., Citation2017; Tang et al., Citation2019). Furthermore, it is worth noting that Tramadol at high doses could disrupt the electron transfer chain in mitochondria, leading to oxidative stress and potential brain tissue damage. This is particularly concerning given the brain’s high oxygen consumption and vulnerability to oxidative damage (Corbett et al., Citation2019; Dave et al., Citation2019; Dhesi et al., Citation2023).
4.3. Changes in cellular layer pattern of cerebral and cerebellar cortices
The cerebral cortex of neonates exposed to Tramadol showed pronounced defects in the cellular layering pattern. This was evidenced by wide areas of separation between adjacent layers, disorganisation, and vacuolated foci indicating cellular loss. This observation echoes the findings of Couly et al. (Citation2022), who also reported disorganisation and neurocyte degeneration in the cortical layers upon long-term Tramadol treatment (Couly et al., Citation2022). The impact on cerebral cellular patterns could be linked to Tramadol’s influence on neuronal stem cells and progenitors during the prenatal period, as neuronal migration is crucial in forming cortical layers (Couly et al., Citation2022).
In the cerebellar cortex, the most notable change was the disappearance of the Purkinje cell layer. This alteration suggests a neurotoxic impact of Tramadol on the early-forming Purkinje cell precursors in the cerebellum (Bai et al., Citation2022). Similar cerebellar changes were reported by Bai et al. (Citation2022), who reported on morphine’s effects on Purkinje cells and indicated a developmental window where these cells are highly susceptible to opioid-induced damage, affecting differentiation and survival (Bai et al., Citation2022).
These findings underscore the potential neurotoxic effects of Tramadol on brain development, particularly impacting critical stages of neurogenesis and cellular organisation in the brain tissues.
4.4. Vascular changes in cerebral and cerebellar cortices
vascular changes observed in the cerebral and cerebellar cortices after Tramadol treatment included vessel dilation, rupture, and cortical haemorrhage. This pattern suggests a connection with increased APP expression, potentially leading to amyloid angiopathy, a condition marked by amyloid deposition in blood vessels (Chwalisz, Citation2021; Szidonya & Nickerson, Citation2023). Cerebral amyloid angiopathy (CAA), as explained by Chwalisz (Citation2021), can cause various haemorrhagic and ischaemic brain lesions (Chwalisz, Citation2021). The correlation between increased APP expression and vascular changes is also evident in Down’s syndrome, and Alzheimer’s disease, where elevated APP levels contribute to vascular anomalies. This raises important considerations about the neurovascular impact of Tramadol, especially regarding its potential to induce changes resembling those seen in neurodegenerative disorders (Hamina et al., Citation2018; Salarinasab et al., Citation2020; Taipale et al., Citation2018).
4.5. Effect of APP expression in cerebral and cerebellar cortices
Significant changes in APP expression were observed in our study, particularly the dose-dependent increase in the cerebral cortex following Tramadol treatment, align with findings from previous research (Arai et al., Citation2019; Brendel et al., Citation2014). Moreover, it is worth to highlight the APP's role in neuroprotection, particularly under acute cellular stress conditions such as hypoxia, ischaemia, or traumatic brain injury, suggesting its upregulation is a defensive response to cellular insult (Dar & Glazner, Citation2020; Peng et al., Citation2021). Similarly, the over-expression of APP following mechanical insults in various animal models, indicating its acute neuroprotective effects (Dar & Glazner, Citation2020; Peng et al., Citation2021). Additionally, other studies reported that intra-ventricular administration of APP post-traumatic brain injury could reduce axonal injury and apoptosis, improving motor and cognitive outcomes in rats (Dar & Glazner, Citation2020). These studies collectively suggest that the increase in APP expression in response to Tramadol may be part of a neuroprotective response to the drug’s neurotoxic effects.
Additionally, APP plays a role in the regulation of synaptic vesicle exocytosis, modulates glutamatergic, GABAergic, and cholinergic synaptic transmission, and contributes to synapse formation (Cai et al., Citation2023). Intriguingly, it has been found to prevent neurodegeneration by interacting with a wide range of survival-related signalling pathways and mediating various protective effects to neurons (Bai et al., Citation2022; Cai et al., Citation2023; Liu et al., Citation2021).
In the cerebellar cortex, APP expression also exhibited a proportional increase with the dose of treatment. Nonetheless, there was a noticeable difference between the groups, especially concerning group C of the study group, which received the highest dose of Tramadol treatment. This indicates that the expression of APP in the cerebellum might exhibit its neuroprotective effect later compared to its manifestation in the cerebral cortex.
The brain tissue injury induced by Tramadol could be attributed to factors such as hypoxia and haemorrhage, as the drug affects cerebral cortical vessels, as demonstrated in our results. This leads to the overexpression of APP. The recognised trophic effects of APP on viability of nerves might also influence the recovery from mechanical trauma traumatic and metabolic injury, as well as to the counteraction of degeneration (Bai et al., Citation2022; Cai et al., Citation2023; Liu et al., Citation2021).
Taken together, these findings regarding changes in cerebral and cerebellar cellular structures, apoptotic alterations, and the overexpression of APP by neurons collectively underscore the potential harmful effects of Tramadol use during pregnancy, particularly when initiated early in pregnancy, used over extended periods, and in high doses.
Future research should consider expanding the range of neurotoxicity biomarkers and employing advanced analytical techniques to further investigate tramadol’s effects on foetal development. Additionally, exploring complementary histological staining methods could enhance our understanding of the structural changes induced by tramadol. These approaches will enrich our insights into tramadol-induced neurotoxicity and potentially guide the development of mitigation strategies.
5. Conclusion
In summary, our study highlights the toxic effects of Tramadol on neurons in the developing foetal brain, particularly in the cerebral and cerebellar cortices. We observed significant changes in cellular structures, apoptosis, and an increase in amyloid precursor protein (APP) expression, indicating potential risks associated with Tramadol use during pregnancy. APP's neuroprotective role and its upregulation in response to Tramadol-induced cellular insult suggest the need for caution when considering this drug during pregnancy. These findings emphasise the importance of further research to better understand the mechanisms underlying these effects and to explore potential strategies for mitigating the neurotoxic impact of Tramadol on foetal brain development.
Informed consent statement
N/A.
Author contributions
The authors worked jointly and equally on this manuscript. All the authors approved the final version of the manuscript.
Table 1.docx
Download MS Word (14.6 KB)Acknowledgments
The authors express their gratitude to all the staff at the High Institute of Diagnosis and Assisted Reproductive Technology (HIDART) at Al-Nahrain University, Baghdad, Iraq for providing the technical support necessary for the successful completion of this work.
Disclosure statement
No potential conflict of interest was reported by the author(s).
Data availability statement
The datasets are available from the corresponding author upon request.
Additional information
Funding
References
- Ahmadimanesh, M., Naeini, M. B., Rouini, M. R., Shadnia, S., & Ghazi-Khansari, M. (2020). Assessment of tramadol pharmacokinetics in correlation with CYP2D6 and clinical symptoms. Drug Metabolism and Personalized Therapy, 35(2), 1–10. doi:10.1515/dmpt-2019-0021
- Arai, Y., Iwasaki, Y., Suzuki, T., Ide, S., & Kaga, M. (2019). Elimination of amyloid precursor protein in senile plaques in the brain of a patient with Alzheimer-type dementia and down syndrome. Brain & Development, 41(1), 106–110. doi:10.1016/j.braindev.2018.07.017
- Asahi, H., Inoue, S.-I., Niikura, M., Kunigo, K., Suzuki, Y., Kobayashi, F., & Sendo, F. (2017). Profiling molecular factors associated with pyknosis and developmental arrest induced by an opioid receptor antagonist and dihydroartemisinin in Plasmodium falciparum. PLOS One, 12(9), e0184874. doi:10.1371/journal.pone.0184874
- Bai, J., Ye, T., Wei, Y. B., Yang, Y., Yang, H. M., & Lan, Y. (2022). Opioid receptors modulate parallel fiber-Purkinje cell synaptic transmission in mouse cerebellum. Neuroscience Letters, 770, 136356. doi:10.1016/j.neulet.2021.136356
- Bancroft, J. D., & Layton, C. (2013). 10 - The hematoxylins and eosin. In S. K. Suvarna, C. Layton, & J. D. Bancroft (Eds.), Bancroft’s Theory and Practice of Histological Techniques (Seventh Edition) (pp. 173–186). Oxford: Churchill Livingstone.
- Brendel, M., Delker, A., Rötzer, C., Böning, G., Carlsen, J., Cyran, C., … Rominger, A. (2014). Impact of partial volume effect correction on cerebral β-amyloid imaging in APP-Swe mice using [(18)F]-florbetaben PET. NeuroImage, 84, 843–853. doi:10.1016/j.neuroimage.2013.09.017
- Bateman, B. T., Hernandez-Diaz, S., Straub, L., Zhu, Y., Gray, K. J., Desai, R. J., … Huybrechts, K. F. (2021). Association of first trimester prescription opioid use with congenital malformations in the offspring: Population based cohort study. BMJ, 372, n102. doi:10.1136/bmj.n102
- Cai, W., Li, L., Sang, S., Pan, X., & Zhong, C. (2023). Physiological roles of β-amyloid in regulating synaptic function: Implications for AD pathophysiology. Neuroscience Bulletin, 39(8), 1289–1308. doi:10.1007/s12264-022-00985-9
- Chwalisz, B. K. (2021). Cerebral amyloid angiopathy and related inflammatory disorders. Journal of the Neurological Sciences, 424, 117425. doi:10.1016/j.jns.2021.117425
- Corbett, K., Dugan, A., Vitale, C., & Gravel, T. (2019). Long-term effects of opioids on the cardiovascular system: Examine the evidence. Nursing, 49(4), 47–49. doi:10.1097/01.Nurse.0000554247.12754.28
- Couly, S., Goguadze, N., Yasui, Y., Kimura, Y., Wang, S.-M., Sharikadze, N., … Su, T.-P. (2022). Knocking out sigma-1 receptors reveals diverse health problems. Cellular and Molecular Neurobiology, 42(3), 597–620. doi:10.1007/s10571-020-00983-3
- Dar, N. J., & Glazner, G. W. (2020). Deciphering the neuroprotective and neurogenic potential of soluble amyloid precursor protein alpha (sAPPα). Cellular and Molecular Life Sciences, 77(12), 2315–2330. doi:10.1007/s00018-019-03404-x
- Dave, C. V., Goodin, A., Zhu, Y., Winterstein, A., Wang, X., Alrwisan, A., & Hartzema, A. (2019). Prevalence of maternal-risk factors related to neonatal abstinence syndrome in a commercial claims database: 2011-2015. Pharmacotherapy, 39(10), 1005–1011. doi:10.1002/phar.2315
- Dhesi, M., & Maldonado, K. A, CV, M. (2023). & Tramadol: Stat Pearls Publishing, FL, USA.
- Edinoff, A. N., Kaplan, L. A., Khan, S., Petersen, M., Sauce, E., Causey, C. D., … Kaye, A. D. (2021). Full opioid agonists and tramadol: Pharmacological and clinical considerations. Anesthesiology and Pain Medicine, 11(4), e119156. doi:10.5812/aapm.119156
- Els, C., Jackson, T. D., Kunyk, D., Lappi, V. G., Sonnenberg, B., Hagtvedt, R., … Straube, S. (2017). Adverse events associated with medium- and long-term use of opioids for chronic non-cancer pain: An overview of cochrane reviews. The Cochrane Database of Systematic Reviews, 10(10), Cd012509. doi:10.1002/14651858.CD012509.pub2
- Fishman, B., Daniel, S., Koren, G., Lunenfeld, E., & Levy, A. (2019). Pregnancy outcome following opioid exposure: A cohort study. PLOS One, 14(7), e0219061. doi:10.1371/journal.pone.0219061
- Ghoneim, F. M., Khalaf, H. A., Elsamanoudy, A. Z., & Helaly, A. N. (2014). Effect of chronic usage of tramadol on motor cerebral cortex and testicular tissues of adult male albino rats and the effect of its withdrawal: Histological, immunohistochemical and biochemical study. International Journal of Clinical and Experimental Pathology, 7(11), 7323–7341.
- Grond, S., & Sablotzki, A. (2004). Clinical pharmacology of tramadol. Clinical Pharmacokinetics, 43(13), 879–923. doi:10.2165/00003088-200443130-00004
- Guedes-Dias, P., & Holzbaur, E. L. F. (2019). Axonal transport: Driving synaptic function. Science, 366(6462), 1–35. doi:10.1126/science.aaw9997
- Guo, Q., Li, H., Gaddam, S. S., Justice, N. J., Robertson, C. S., & Zheng, H. (2012). Amyloid precursor protein revisited: Neuron-specific expression and highly stable nature of soluble derivatives. The Journal of Biological Chemistry, 287(4), 2437–2445. doi:10.1074/jbc.M111.315051
- Haass, C., Kaether, C., Thinakaran, G., & Sisodia, S. (2012). Trafficking and proteolytic processing of APP. Cold Spring Harbor Perspectives in Medicine, 2(5), a006270–a006270. doi:10.1101/cshperspect.a006270
- Hamina, A., Lavikainen, P., Tanskanen, A., Tolppanen, A. M., Tiihonen, J., Hartikainen, S., & Taipale, H. (2018). Impact of opioid initiation on antipsychotic and benzodiazepine and related drug use among persons with Alzheimer’s disease. International Psychogeriatrics, 30(7), 947–956. doi:10.1017/s1041610217002897
- Hick, M., Herrmann, U., Weyer, S. W., Mallm, J.-P., Tschäpe, J.-A., Borgers, M., … Müller, U. C. (2015). Acute function of secreted amyloid precursor protein fragment APPsα in synaptic plasticity. Acta Neuropathologica, 129(1), 21–37. doi:10.1007/s00401-014-1368-x
- Horobin, R. W. (2013). 9 - How histological stains work. In S. K. Suvarna, C. Layton, & J. D. Bancroft (Eds.), Bancroft’s Theory and Practice of Histological Techniques (Seventh Edition) (pp. 157–171). Oxford: Churchill Livingstone.
- Le Bras, A. (2021). New insights into the APP family. Lab Animal, 50(7), 166. doi:10.1038/s41684-021-00803-1
- Liu, L., Lauro, B. M., Wolfe, M. S., & Selkoe, D. J. (2021). Hydrophilic loop 1 of Presenilin-1 and the APP GxxxG transmembrane motif regulate γ-secretase function in generating Alzheimer-causing Aβ peptides. The Journal of Biological Chemistry, 296, 100393. doi:10.1016/j.jbc.2021.100393
- Mochida, G. H., Poduri, A., & Walsh, C. A. (2013). Chapter 115 - genetic disorders of cerebral cortical development. In D. Rimoin, R. Pyeritz, & B. Korf (Eds.), Emery and Rimoin’s Principles and Practice of Medical Genetics (Sixth Edition) (pp. 1–26). Oxford: Academic Press.
- Nguyen, K. V. (2019). β-Amyloid precursor protein (APP) and the human diseases. AIMS Neuroscience, 6(4), 273–281. doi:10.3934/Neuroscience.2019.4.273
- O'Brien, R. J., & Wong, P. C. (2011). Amyloid precursor protein processing and Alzheimer’s disease. Annual Review of Neuroscience, 34(1), 185–204. doi:10.1146/annurev-neuro-061010-113613
- Peng, Z., Bedi, S., Mann, V., Sundaresan, A., Homma, K., Gaskey, G., … Doursout, M.-F. (2021). Neuroprotective effects of asparagus officinalis stem extract in transgenic mice overexpressing amyloid precursor protein. Journal of Immunology Research, 2021, 8121407–8121410. doi:10.1155/2021/8121407
- Perry, C., Chung, J.-Y., Ylaya, K., Choi, C. H., Simpson, A., Matsumoto, K. T., … Hewitt, S. M. (2016). A buffered alcohol-based fixative for histomorphologic and molecular applications. The Journal of Histochemistry and Cytochemistry: Official Journal of the Histochemistry Society, 64(7), 425–440. doi:10.1369/0022155416649579
- Richter, M. C., Ludewig, S., Winschel, A., Abel, T., Bold, C., Salzburger, L. R., … Müller, U. C. (2018). Distinct in vivo roles of secreted APP ectodomain variants APPsα and APPsβ in regulation of spine density, synaptic plasticity, and cognition. The EMBO Journal, 37(11), 1–23. doi:10.15252/embj.201798335
- Salarinasab, S., Salimi, L., Alidadiani, N., Shokrollahi, E., Arzhanga, P., Karbasforush, S., … Nikanfar, M. (2020). Interaction of opioid with insulin/IGFs signaling in Alzheimer’s disease. Journal of Molecular Neuroscience, 70(6), 819–834. doi:10.1007/s12031-020-01478-y
- Shamsi Meymandi, M., & Keyhanfar, F. (2013). Assessment of the antinociceptive effects of pregabalin alone or in combination with morphine during acetic acid-induced writhing in mice. Pharmacology, Biochemistry, and Behavior, 110, 249–254. doi:10.1016/j.pbb.2013.07.021
- Specialists, O. o T. I. (2021). Mother To Baby: Fact Sheets: Organization of Teratology Information Specialists (OTIS).
- Sullivan, M. D., & Ballantyne, J. C. (2022). Understanding the risks of long-term opioid therapy for chronic pain. The American Journal of Psychiatry, 179(10), 696–698. doi:10.1176/appi.ajp.20220592
- Szidonya, L., & Nickerson, J. P. (2023). Cerebral amyloid angiopathy. Radiologic Clinics of North America, 61(3), 551–562. doi:10.1016/j.rcl.2023.01.009
- Taipale, H., Hamina, A., Lampela, P., Tanskanen, A., Tiihonen, J., Karttunen, N., … Hartikainen, S. (2018). Is Alzheimer’s disease associated with previous opioid use? Pain Medicine (19(11), 2115–2121. doi:10.1093/pm/pnx210
- Tang, D., Kang, R., Berghe, T. V., Vandenabeele, P., & Kroemer, G. (2019). The molecular machinery of regulated cell death. Cell Research, 29(5), 347–364. doi:10.1038/s41422-019-0164-5
- Yazdy, M. M., Desai, R. J., & Brogly, S. B. (2015). Prescription opioids in pregnancy and birth outcomes: A review of the literature. Journal of Pediatric Genetics, 4(2), 56–70. doi:10.1055/s-0035-1556740
- Zebala, J. A., Searle, S. L., Webster, L. R., Johnson, M. S., Schuler, A. D., Maeda, D. Y., & Kahn, S. J. (2019). Desmetramadol has the safety and analgesic profile of tramadol without its metabolic liabilities: consecutive randomized, double-blind, placebo- and active comparator-controlled trials. The Journal of Pain, 20(10), 1218–1235. doi:10.1016/j.jpain.2019.04.005
- Zheng, H., & Koo, E. H. (2011). Biology and pathophysiology of the amyloid precursor protein. Molecular Neurodegeneration, 6(1), 27. doi:10.1186/1750-1326-6-27