Abstract
Acanthospermum hispidum DC (Asteraceae), a traditional medicinal plant, plays a role as an alternative remedy for various diseases, such as bacterial and viral infections, jaundice, malaria, fever, gastrointestinal disorders, headache, convulsions, and snake bites. Flavonoids, alkaloids, saponins, tannins, terpenes, steroids, and cardioactive glycosides are the distinct classes of metabolites in the plant. Although A. hispidum was suggested as a promising antitumor phytomedicine, no studies identified its potential cytotoxic components. In this study, the cytotoxic compounds of A. hispidum were isolated using chromatographic techniques guided by in vitro MTT cytotoxicity assay against selected cancer cell lines; breast cancer (MCF7), colorectal adenocarcinoma (HT29), and hepatoblastoma (HepG2). The selective index (SI) was assessed on MRC5 (Normal human fetal lung fibroblast) cell line. The dichloromethane fraction (DCM) showed remarkable cytotoxic activity against MCF7 and HT29 (%Cell viability; 46.15 and 60.5, respectively). Hence, the main bioactive fraction from DCM was purified to afford two phytosterols; stigmasterol (1) and β-sitosterol (2), which were identified by 1-D and 2-D NMR spectroscopy. Cytotoxic evaluation of 1 and 2 revealed that β-sitosterol showed better selective cytotoxicity against MCF7 and HT29 (IC50 4.07 μg/mL, SI 2.63; IC50 4.52 μg/mL, SI 2.37) compared to stigmasterol (IC50 5.43 μg/mL, SI 1.38; IC50 4.21 μg/mL, SI 1.78), respectively. Bioinformatic assessments of drug-likeness and ADMET properties demonstrated that most criteria were obeyed by the investigated compounds except for their poor solubility, which recommended the preparation of special dosage forms, such as nanoformulation to enhance their oral bioavailability. Swiss Target prediction indicated that nuclear receptors represent the main target class (40%). Whereas caspase-3 stimulant activity (a key enzyme in apoptosis) was predicted by the PASS prediction tool as a potential anticancer mechanism. Our study suggests A. hispidum as a potential source of bioactive phytosterols and as a chemopreventive medicinal plant.
1. Introduction
Cancer is one of the most life-threatening diseases, which creates a major problem in both developing and developed countries. It is now the world’s second leading cause of death after cardiovascular disease. It can affect anyone at any age, and it is characterized by uncontrolled cell growth and proliferation (Cassileth & Deng, Citation2004; Gezici & Şekeroğlu, Citation2019; Chandrasekar et al., Citation2018; Sultana et al., Citation2019). Even though there are various types of cancer treatments, such as chemotherapy, immune, hormone, radiation, and targeted therapies, however, they all have side effects and are generally costly (Cassileth & Deng, Citation2004; Chandrasekar et al., Citation2018; Sultana et al., Citation2019). As an alternative therapy, several medicinal plants are administered to cancer patients to prevent and treat cancer. These plants also have less toxic anticancer, anti-tumor, and anti-proliferation agents than conventional allopathic therapeutics (Cassileth & Deng, Citation2004; Sultana et al., Citation2019). On the other hand, a lot of metabolites have been successfully developed into anticancer properties, such as berberine, evodiamine, piperine, matrine, sanguinarine, tetrandrine, camptothecin, and vinblastine (Lu et al., Citation2012). As a result, we may say natural products could be a key source of antitumor agents in modern anticancer therapy.
Sudan is rich in medicinal plants that possess diverse ethnobotanical applications, including anticancer activity, such as but not limited to Grewia villosa Willd., Annona senegalensis Pers., Cassia occidentalis L., Citrullus colocynthis (L.) Schrad., Combretum hartmannianum Schweinf., Erythrina abyssinica L., Croton zambesicus Mull-Arg., Fagonia cretica L., Leptadenia pyrotechnica (Forssk.) DC., Khaya senegalensis (Desr.) A. Juss., and Balanites aegyptiaca (L.) Del (Karar & Kuhnert, Citation2017). Acanthospermum hispidum DC., commonly known as “bristly starbur” or “goat’s head,” is a Sudanese plant that belongs to the Asteraceae family and is also a well-known medicinal plant in tropical America, Asia, and some parts of Africa (Adukpo et al., Citation2020; Araújo et al., Citation2008; Azeez & Banigo, Citation2018). Several attempts have been made to use A. hispidum as an alternative therapy for infectious diseases, malaria, viral illnesses, jaundice, fever, snake bite, headache, and convulsions. Additionally, A. hispidum leaves are used to treat various GIT problems, such as vomiting, stomach discomfort, and constipation (Chakraborty et al., Citation2012; Santos et al., Citation2022). Several in vitro and in vivo biological activities of the plant, including antioxidant, antimicrobial, antiparasitic, hepatoprotective, anticholinesterase, and hypoglycemic activities were reported by research groups (Adepiti et al., Citation2014; Arena et al., Citation2011; Edewor & Olajire, Citation2011; Koukouikila-Koussounda et al., Citation2013; N'Do J et al., Citation2018; Santos et al., Citation2022). The reported chemical composition of A. hispidum included terpenoids, flavonoids, alkaloids, steroids, hydrocarbons, anthracene derivatives, and tannins (Arena et al., Citation2011; Cartagena et al., Citation2000; Edewor & Olajire, Citation2011; Olawumi et al., Citation2014; Santos et al., Citation2022). Sesquiterpenic lactones, including guaianolides, germacranolides, and melampolides have been reported from the aerial parts of the Argentinian A. hispidum (Cartagena et al., Citation2000). Acanthospermal B was identified by another research group as the major sesquiterpene lactone of the Argentinian plant (Arena et al., Citation2011). The major constituents of the essential oil of A. hispidum were shown to be β-caryophyllene (35.2%), α-bisabolol (11.4%), and germacrene D (11.1%).
Encouraging antitumor activity was reported for several A. hispidum extracts, such as the ethanolic and methanolic extracts of the leaf, the ethyl acetate extract, and the diterpene-rich fraction of the whole plant against several cell lines, including HeLa cells, human rhabdomyosarcoma cells, and Dalton’s lymphoma cells in the ascitic form (Deepa & Rajendran, Citation2007; Koukouikila-Koussounda et al., Citation2013; Ogbole et al., Citation2017; Santos et al., Citation2022). However, no studies isolated the compounds responsible for the antitumoral activity of the plant.
Thus, the aim of this study was to isolate the major cytotoxic compounds of A. hispidum through a bio-guided isolation technique and to test their cytotoxic activities against breast cancer (MCF7), hepatocellular carcinoma (HepG2), and colorectal carcinoma (HT29) cell lines. Additionally, to conduct a bioinformatic study of the isolated compound by various in silico prediction tools to establish their drug-likeness properties and to predict the possible underlying mechanism of their in vitro anticancer action.
2. Materials and methods
2.1. General
Petroleum-ether (Pet-ether), n-hexane (Hex), and chloroform (CHCl3), dichloromethane (DCM), ethyl acetate (EtOAc), ethanol (EtOH), methanol (MeOH), Dimethylsulfoxide (DMSO) and vanillin/conc.H2SO4 were purchased from Rovan chemical CO., Khartoum, Sudan and were used without purification. Silica gel (60–120 Mesh) and RP-C18 were used for column chromatography (Merck Millipore, Darmstadt, Germany). Thin layer chromatography (TLC) and preparative layer chromatography (PLC) (0.25 and 0.75 mm, respectively), were obtained from Merck Millipore, Darmstadt, Germany. The TLC chromatograms were visualized using long and short-wave ultraviolet light (UV) (366 nm and 254 nm, respectively), sulfuric acid spray reagent, phosphomolybdic acid stain, and Hanessian’s stain (cerium molybdate) with heat. Rotary evaporator R-215/Vacuum Controller V-850 (Buchi, Switzerland). NMR spectra were recorded on a Bruker UltraShield Plus 500 MHz spectrometer (NMR Unite, College of Pharmacy, Prince Sattam Bin Abdulaziz University) and the chemical shifts reported in δ (ppm) relative to deuterated solvent (Pyridine-d5 at 7.19, 7.56, and 8.71 ppm in 1H-NMR and 123.1, 135.1, and 149.4 ppm in 13C-NMR). The following abbreviations were used to explain the 1H-NMR data: chemical shift δ (ppm), multiplicity (s = singlet, d = doublet, t = triplet, q = quartet, m = multiplet, br = broad), and coupling constant (J, measured in hertz).
2.2. Plant material
Acanthospermum hispidum DC was collected from western Sudan in October of 2018. The plant was authenticated by the botanist Yahiya Suliman from the herbarium of the Department of Phytochemistry and Taxonomy, Medicinal and Aromatic Plants and Traditional Medicine Research Institute, National Center for Research, Khartoum, Sudan.
2.3. Extraction
The whole plant was carefully cleansed with tap water and dried at room temperature in the shade and ground using a pestle and mortar. The obtained coarsely powdered plant material (2366 g) was macerated using 96% of ethanol (2000 mL). The flask was tightly closed and kept overnight at room temperature for 72 h. The mixture was then carefully filtered with cotton tissue and concentrated to dryness using a rotary evaporator machine at 57 °C. The extraction, filtration, and evaporation were repeated three times with recovery ethanol until all the crude contents (175 g) were extracted.
2.4. Liquid–liquid fractionation
Precisely, 123 g of the total ethanol extract was completely dissolved in water, and fractionated with CHCl3 using a separating funnel, two phases were obtained, collected, concentrated to dryness, and labeled as chloroform and aqueous fractions. The chloroform fraction was dissolved in n-hexane and partitioned with a mixture of MeOH–H2O (90:10 v/v) and yielded two fractions; n-hexane fractions and MeOH–H2O (90:10 v/v). After that, the MeOH–H2O (90:10 v/v) fraction was diluted to (80:20 v/v) to give an 80% MeOH fraction. Finally, the diluted fraction was fractionated with CH2Cl2 to provide the dichloromethane (DCM) fraction. The quantities and percentage yields of obtained extracts and fractions are recorded in . About 2 g of the total extract was kept for the in vitro anticancer assays. Likewise, an amount of 50 mg from each fraction was required for the anticancer assay.
Table 1. The quantities and percentage yields of extracts and fractions obtained from the extraction and fractionation procedures of Acanthospermum hispidum.
2.5. Purification and isolation
A mixture of 16 g of DCM fraction and 16 g of SiO2 was subjected to column chromatography using 510 g of SiO2 packed on 5 × 60 (diameter × height) column with two gradients: Pet-ether/EtOAc (0 to 100% EtOAc) and EtOAc/MeOH (0 to 100% MeOH) this led to nine fractions, which have been all tested on MCF7 and HT29 cells. Among the most active fractions, AC4 (1047 g) was submitted to preparative TLC plates using 20% EtOAc in n-hexane to furnish 7 fractions (AC4-1 to AC4-7). Then, AC4-3 (18.8 g) was further purified on a medium-pressure liquid chromatography (MPLC) column using RP-C18, MeOH-H2O (gradient, from 20 to 100% MeOH), and an effluent volume of 10 mL. Fractions (F2: 70–73) and (F4: 77–81) eluted with MeOH (100%) afforded compounds 1 and 2, respectively (). The isolated compounds were analyzed by 1 and 2D NMR spectroscopy.
2.6. Cell culture
MRC5 (human normal fetal lung fibroblasts) and three cancer cell lines (MCF7, human breast adenocarcinoma, HT29, colorectal adenocarcinoma, and hepatoblastoma, HepG2) were obtained from the ATCC, USA. For a maximum of 5 to 10 passages, the examined cancer cells were subcultured in RPMI-1640 media (10% FBS), whereas MRC5 was kept in EMEM, 10% FBS. All of these conditions were maintained at 37 °C, 5% CO2, and 100% relative humidity (Abdalla et al., Citation2020).
2.7. Cytotoxic activity assay
The cytotoxic activity of the tested extracts, pure compounds in addition to doxorubicin, were estimated by MTT cytotoxicity assay, according to the reported procedure (Abdalla et al., Citation2020). Separate cultures of each cell line were placed in 96-well (3 × 103/well) wells and was incubated with the test sample for three days at 37 °C overnight (DMSO 0.1%; n = 3 of three independent experiments) at final concentrations of 0–100 μg/mL and 0–50 μM, respectively. Each compound’s cytotoxicity was assessed using the MTT test following a 3-day incubation period. After adding 0.5 mg/mL of MTT in culture media to each well, the mixture was incubated for 3 h at 37 °C. Dimethyl sulfoxide (DMSO) was used to dissolve the formazan granules after the MTT solution was eliminated. A multi-plate reader (BIORAD, PR 4100, Hercules, CA, USA) was used to measure absorbance. The number of live cells is correlated with the optical density of the purple formazan at A550. GraphPad Prism was used to calculate the extract concentration that caused 50% inhibition (IC50) relative to 100% growth of control cells. The selectivity index (SI) for the twenty-three compounds was calculated according to a previous reference (Abdalla et al., Citation2021) by dividing the IC50 in normal cells (MRC-5 cells) and the IC50 of cancer cells (MCF7, HT29, and HepG2).
2.8. In silico drug-likeness prediction experiments
2.8.1. SwissADME prediction of ADMET and drug-likeness
The SwissADME online (http://www.sib.swiss) platform (Swiss Institute of Bioinformatics, Lausanne, Switzerland) was used to examine the physicochemical, pharmacokinetics, drug-likeness, and toxicity characteristics of compounds (Daina et al., Citation2017).
2.8.2. MolSoft drug-likeness
The online MolSoft toolkit was used to predict the drug-likeness of the isolated compounds (https://molsoft.com/mprop/) (Anwar et al., Citation2020; Gad et al., Citation2020).
2.8.3. OSIRIS prediction of toxicological properties
The OSIRIS Property Explorer (https://www.organic-chemistry.org/prog/peo/) predicts properties with high risks of undesired effects like mutagenicity or poor intestinal absorption are shown in red. Whereas a green color indicates drug-conform behavior (Zhang et al., Citation2022).
2.8.4. Swiss target prediction
Swiss Target Prediction is a ligand-based in silico target prediction online (http://www.swisstargetprediction.ch/) platform (Swiss Institute of Bioinformatics, Lausanne, Switzerland) (Daina et al., Citation2019). This prediction method is based on the SAR studies of data obtained in various types of assays, except biochemical assays. It involves direct interactions with 930 human protein targets. Confidence is the difference between the possibility that a chemical substance will interact and not interact with a specific target. The possibility that a good prediction will come true is higher when confidence levels are higher (Agamah et al., Citation2019; Celikler Ozer et al., Citation2021).
2.8.5. PASS prediction of biological activities
The PASS Online (http://www.way2drug.com/passonline/predict.php) platform was used to predict the biological activity of isolated compounds 1 and 2. It is a software created as a tool for assessing the overall biological potential of an organic drug-like organic molecule (Celikler Ozer et al., Citation2021; Filimonov et al., Citation1995).
3. Results
3.1. NMR spectral characterization of the isolated compounds
The 1H NMR data of compound 1 (Table S1) was characterized by the presence of upfield resonances at δH 0.68–2.08 (saturated aliphatic skeleton), 2.62 (2H, brs; H-4), 3.84 (1H, brs; H-3), 5.40 (1H, brs; H-6), 5.18 (1H, m; H-22), and 5.05, m (1H, overlapped, determined with HSQC; H-23). The 13C NMR spectrum of 1 displayed twenty-nine carbon signals, which were classified by DEPT135 spectrum into 11 methine, 9 methylene, and 6 methyl, in addition to three quaternary carbons. The most characteristic signals included these assigned for the two double bonds at C-5 (δC 141.8; C-5 & δC 121.0; C-6) and C-22 (δC 138.7; C-22 & δC 129.2; C-23). The assignment of all protons and carbons was performed with the aid of the HSQC spectrum and confirmed with HMBC and COSY spectra.
The 1H NMR data of compound 2 (Table S1) showed upfield resonances at δH 0.68–2.08 (saturated aliphatic skeleton), 2.64 (H-4), 3.85 (1H, brs; H-3), and 5.41 (1H, brs; H-6). The 13C NMR spectrum of 2 showed the presence of twenty-nine carbon signals with the presence of olefinic resonances at δC 141.7 (C-5) and 121.0 (C-6). DEPT135 spectrum classified these carbon signals into 9 methine, 11 methylene, and 6 methyl carbons, in addition to three quaternary carbons. It showed two downfield methines at δC 121.0 (C-6) and 71.1 (C-3). Interpretation of all protons was assigned and confirmed using 2D experiments, including HSQC, HMBC, and COSY.
3.2. Bio-guided isolation, cytotoxicity and selectivity studies
To start a bioguided fractionation study, the cytotoxic activity of the crude methanol extract of A. hispidum was investigated by in vitro MTT cytotoxicity assay against three human cancer cell lines; MCF7, HT29, and HepG2 and doxorubicin as a positive control. The selective cytotoxic activity was examined using the normal MRC5 cell line. The results are recorded in .
Table 2. Cytotoxic activity of the crude extract of Acanthospermum hispidum DC against three cell lines and one normal fibroblast cell line (MTT, 72 h, selectivity index (SI), IC50 μg/mL ± SD, n = 3).
Therefore, the crude ethanol extract was further fractionated successively to afford the n-hexane, dichloromethane, aqueous, and methanol 80% extracts according to the previously described procedure. Also, the cytotoxic activity of the obtained extracts was evaluated against the cancer cell lines: MCF7, and HT29. The results are recorded in .
Table 3. %Cell viability obtained by treatment of two cancer cell lines with the different fractions of Acanthospermum hispidum against (MTT, 72 h, ±SD, n = 3).
Thus, the DCM fraction was subjected to chromatographic column fractionation to yield nine groups of subfractions. The potential cytotoxic activity of the obtained subfractions (1–9) was examined on MCF7, and HT29 cell lines, and the results are recorded in .
Table 4. %Cell viability obtained by treatment of two cancer cell lines; MCF7 and HT29 with subfractions (1–9) of the DCM of Acanthospermum hispidum (MTT, 72 h, ±SD, n = 3).
Amongst the most active fractions (), fraction AC4 (1047 g) was purified by preparative TLC plates to provide 7 fractions (AC4-1 to AC4-7). Then, AC4-3 (18.8 g) was further purified to afford compounds 1 and 2. The cytotoxic activity of the isolated compounds (1 and 2) was investigated on MCF7, and HT29 cell lines, and the results are recorded in .
Table 5. Cytotoxic activity of the isolated compounds 1 and 2, doxorubicin, and 5FU against two cancer cell lines, and normal fibroblast cell line (MTT, 72 h, selectivity index (SI), IC50 μM ± SD, n = 3).
3.3. Bioinformatic drug-likeness and target predictions
3.3.1. SwissADME prediction of ADMET and drug-likeness
The in silico physicochemical, pharmacokinetic and toxicity (ADMET), and drug-likeness properties of the isolated compounds; stigmasterol (1) and β-sitosterol (2) were evaluated by the SwissADME prediction tool and the results are recorded in (Daina et al., Citation2017).
Table 6. The SwissADME predicted physicochemical, ADMET properties, and drug-likeness of isolated compounds 1 and 2.
3.3.2. MolSoft drug-likeness
The drug-likeness properties of of stigmasterol (1) and β-sitosterol (2) were also investigated by MolSoft online tool, which is based on plotting a drug-likeness score (Anwar et al., Citation2020; Gad et al., Citation2020). The results are displayed in .
3.3.3. OSIRIS prediction of toxicological properties
The OSIRIS Property Explorer was used to predict the possible adverse and toxic effects of compounds 1 and 2 (Zhang et al., Citation2022).
3.3.4. PASS prediction of toxicological properties
PASS (Prediction of Activity Spectra of Substances) is an online tool used to predict the biological activity of compounds as well as adverse/toxic effects (Celikler Ozer et al., Citation2021; Filimonov et al., Citation1995). displays the top possible adverse and toxic effects (Pa>Pi) by 1 and 2.
Table 7. PASS predicted possible adverse and toxic effects of compounds 1 and 2.
3.3.5. In silico target prediction
The online Swiss Target Prediction tool was used to predict the possible targets of compounds 1 and 2. It is based on the concept of chemical structure similarity, which states that similar ligands or compounds would bind to similar targets with almost the same binding affinity and express similar biological responses (Daina et al., Citation2019). We investigated the possibility of binding 1 and 2 to the available human protein targets database and the results are displayed in and .
Table 8. Selected cancer-related targets predicted for compounds 1 and 2 by the Swiss Target Prediction online platform.
3.3.6. In silico prediction of biological activities
PASS was used to predict the biological activity of drug-like molecules (Filimonov et al., Citation1995). PASS results () were selected based on the anti-cancer-related biological activities.
Table 9. Probability data of selected relevant anti-cancer-related biological activity of compounds 1 and 2 using the PASS online platform.
4. Discussion
4.1. Isolation and identification of bioactive components from the DCM fraction
Phytosterols with a tetracyclic sterol nucleus, a side chain at C-17, and a double bond between C-5 and C-6 are very similar in their structures to cholesterol. β-Sitosterol, campesterol, and stigmasterol, respectively, are the most abundant plant sterols. They are recently used as hypocholesterolemic supplements and have been classified as functional foods by the European Union (Poli et al., Citation2021). Thus, their isolation and characterization procedures are of interest to researchers as well as pharmaceutical companies. Due to similar Rf values and TLC visualization color, several published research reported the separation of stigmasterol and β-sitosterol as a non-resolved mixture (Erwin et al., Citation2020; Pierre & Moses, Citation2015; Shwe et al., Citation2019). Therefore, the current study described a simple method for their isolation using reversed-phase RP-C18 medium-pressure column chromatography (MPLC) and MeOH (100%) as the eluant.
The 1H and 13C NMR data of the isolated phytosterol were carefully interpreted and confirmed by HSQC and HMBC experiments. The obtained data (Table S1) were consistent with that of stigmasterol (Erwin et al., 2020). Thus, the structure of compound 1 was confirmed as stigmasterol ().
The 1H NMR data of compound 2 (Table S1) was closely related to that of 1 except for the absence of the resonances at δH 5.18 and 5.05 corresponding to the protons C-22 and C-23 of the of Δ22 double bond. This was confirmed from the 13C NMR that showed twenty-nine carbon signals with the presence of only one double bond, as deduced from the signals at δC 141.7 (C-5) and 121.0 (C-6). However, the other two olefinic methine carbon signals were replaced by two aliphatic methylene carbon signals at δC 34.0 (C-22) and 26.1 (C-23) as deduced from the DEPT135 spectrum. By comparing the obtained data with those found in the literature, the structure of 2 was confirmed as β-sitosterol, (Erwin et al., 2020).
4.2. Cytotoxic and selectivity assay
The results of cytotoxic activity evaluation of the total ethanol extract of A. hispidum () showed a remarkable cytotoxic activity against the colon cancer (HT29) cell line (3.343 ± 0.22 μg/mL) with a great selectivity index (11.82). This was followed by the breast cancer, MCF7 (4.50 ± 1.28 μg/mL, SI 8.78). However, the hebatoblastoma, HepG2 cells showed lower cytotoxic activity and selectivity index (7.95 ± 1.61 μg/mL, SI 4. 97). Thus, it was further fractionated by liquid–liquid partitioning to obtain four fractions; the n-hexane, DCM, aqueous, and MeOH 80% fractions. As a continuation of the proposed bio-guided fractionation procedure, the cytotoxic activity of the obtained fractions was estimated against the most susceptible two cancer cell lines: MCF7, and HT29. The results () indicated that the DCM fraction was the most active among the tested fractions. Accordingly, the DCM fraction was further purified to produce nine subfractions. The cytotoxic activity of these subfractions was similarly evaluated using the same susceptible cancer cell lines (i.e. MCF7 and HT29). The results () showed that subfraction AC4 could be the major and bioactive fraction. Thus, further purification of AC4 was achieved to afford fractions from AC4-1 to AC4-7. Finally, fraction AC4 was purified to provide compounds 1 and 2.
Compounds 1 and 2 were characterized by NMR spectroscopy as stigmasterol and β-sitosterol as previously mentioned. Both compounds showed cytotoxicity against MCF7 and HT29 cells in the range of 4.07–4.43 μM compared with 0.30–3.83 μM caused by doxorubicin and 5FU, respectively (). However, β-sitosterol showed a better selectivity index (SI) compared to stigmasterol, as β-sitosterol showed an SI value of more than 2. While doxorubicin showed the best SI value ranging from 2.84 to 6.63 against the investigated cancer cell lines. 5FU was less selective as it showed an SI value of 2.15 against MCF7 and it was not selective against HT29 cells ().
It is worth mentioning that the anticancer effect and the underlying potential mechanisms of dietary phytosterols were early demonstrated in the literature (Awad et al., Citation2007; Awad & Fink, Citation2000; Awad et al., Citation1998). MCF7 is considered an estrogen receptor-positive cell line and is a suitable model for early-stage breast cancer. Fas antigen (Fas) is a protein that triggers apoptosis (Schattner & Friedman, Citation1996). Previous reports indicated that β-sitosterol promotes apoptosis in the MCF7 cell line via a specific effect on Fas expression (Awad et al., Citation2007). It also has been shown that after 24 h of β-sitosterol administration, MCF-7 cells expressed 30% more Fas, which was associated with an enhanced caspase-8 activity (1.9-fold) (Schattner & Friedman, Citation1996). Moreover, the anticancer molecular mechanisms of β-sitosterol were recently reviewed by Bao et al. (Citation2022) as displayed in .
Figure 5. Key molecular anti-cancer pathways mediated by β-sitosterol (Bao et al., Citation2022).
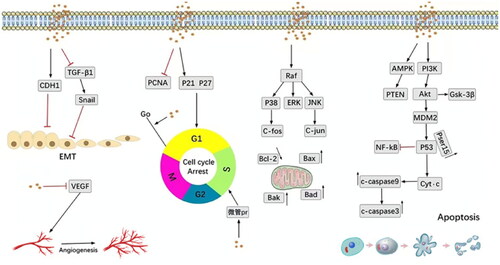
4.3. Drug-likeness, pharmacokinetics, and pharmacodynamics predictions
4.3.1. Prediction of ADMET and drug-likeness
The in silico physicochemical, pharmacokinetic and toxicity (ADMET), and drug-likeness properties of the isolated compounds were investigated by two methods; the SwissADME () (Daina et al., Citation2017) and the MolSoft methods () (Anwar et al., Citation2020; Gad et al., Citation2020).
The SwissADME method showed that the drug-likeness properties by Lipinski’s rule of five, which uses multiple filters, were obeyed by the investigated compounds except for their poor solubility. No PAINS (Pan-assay interference compounds) alerts were identified for the examined compounds. However, they showed expectedly low GIT absorption but a good bioavailability score of 0.55. Also, compounds 1 and 2 demonstrated no predictable inhibition of all CYP450 subtypes or BBB permeability, as well as P-gp protein binding by this prediction tool. The bioavailability radar plot () uses six descriptors for the prediction of oral bioavailability for a rapid judgment of drug-likeness. These include molecular size, polarity, lipophilicity, flexibility, saturation, and solubility. Drug-likeness by this model requires that the red boundaries assumed by the tested molecules be entirely located in the pink zone of the plot (Daina et al., Citation2017). The results showed that the poor solubility and lipophilicity are of special interest and indicated that the investigated compounds may require the preparation of special dosage forms, such as nanoformulation to enhance their oral bioavailability (Soleimanian et al., Citation2020).
The MolSoft method also agreed with those results obtained by the SwissADME method as displayed in , where both compounds (1 and 2) showed a positive value of 0.62 and 0.78, respectively, which suggested drug-likeness properties.
4.3.2. Prediction of toxicological properties
Two methods, viz., the OSIRIS Property Explorer (Zhang et al., Citation2022) and The PASS (Prediction of Activity Spectra of Substances) (Celikler Ozer et al., Citation2021; Filimonov et al., Citation1995) were used to predict the toxicity of the isolated compounds.
The OSIRIS method indicated that both compounds 1 and 2 showed no toxicity risks as indicated by the green color given for mutagenicity, tumorigenicity, irritability, and reproductive effectiveness. However, poor intestinal absorption was assumed for 1 and 2 from their poor solubility (−6.44 and −6.67), cLogP (7.6 and 7.86) and TPSA (20.23 and 20.23), respectively. Generally, stigmasterol (1) was given a better drug-likeness score compared to β-sitosterol (2).
However, the top predicted adverse effects of 1 and 2 obtained by the PASS method involved sleep disturbance, ocular toxicity, teratogenicity, and drowsiness ().
4.3.3. In silico target prediction
The possibility of 1 and 2 to bind the available human protein targets database by the online Swiss Target Prediction was investigated (Daina et al., Citation2019), and the results demonstrated that they are categorized into seven classes (). Nuclear receptors represent the main percentage (40%) of all predicted target classes. This was followed by cytochrome P450 indicating a possible alteration of liver metabolism (Zhao et al., Citation2021). Further data analysis revealed the existence of eight potential anti-cancer targets of stigmasterol (1) and β-sitosterol (2), (). High levels of confidence (0.912575154–0.272758582) were obtained for several nuclear receptors, including Androgen Receptor (AR), LXR-alpha (NR1H3), Nuclear receptor ROR-gamma (RORC), Estrogen receptor alpha (ESR1), and Estrogen receptor beta (ESR2), which have been reported to be incorporated in cancer development (Bilotta et al., Citation2020; Chen et al., Citation2022; Enmark et al., Citation1997; Fuentes & Silveyra, Citation2019; Michmerhuizen et al., Citation2020; Oh et al., Citation2016; Pontini & Marinozzi, Citation2021).
4.3.4. In silico prediction of biological activities
The biological activities of stigmasterol (1) and β-sitosterol (2) were predicted by the PASS online tool (Filimonov et al., Citation1995). The anti-cancer-related biological activities were selected and recorded in . The results indicated that both compounds showed high potential caspase-3 stimulant activity, which was demonstrated to be involved in the apoptotic anti-cancer mechanism of β-sitosterol () (Bao et al., Citation2022). Additionally, they showed potential chemopreventive activity and this result was previously verified on a colon cancer model (Baskar et al., Citation2010). They were also predicted to treat rectal polyps in patients with adenomatous polyposis. Moreover, stigmasterol showed potential inhibition of DNA polymerase I, which is involved in the hyperproliferation of cancer (Berdis, Citation2017). Whereas β-sitosterol may act as a phosphatase inhibitor. Phosphatases are involved in various cellular processes, including cell proliferation, differentiation, and carcinogenesis. Thus, using phosphatase inhibitors could be a beneficial strategy in cancer therapy (Turdo et al., Citation2021).
5. Conclusion
In this study, Acanthospermum hispidum DC (Asteraceae) was subjected to a bio-guided isolation procedure using various chromatographic and non-chromatographic techniques directed by the in vitro cytotoxic activity of the produced fractions. The dichloromethane fraction (DCM) showed the best cytotoxic activity against MCF7 and HT29. Therefore, it was further purified to afford stigmasterol (1) and β-sitosterol (2) as the major bioactive component. The selective index (SI) was detected using the normal human fetal lung fibroblasts (MRC5 cell line). β-sitosterol showed better selective cytotoxic activity compared to stigmasterol. Investigation of the drug-likeness, target prediction, and physicochemical properties demonstrated that both compounds obeyed Lipinski’s rule of five, but they showed poor solubility. We recommended nanoformulation for enhancing their oral bioavailability. Swiss Target Prediction and PASS prediction tools considered various potential drug targets for the isolated phytosterols. We selected the main targets that may be involved in cancer. These targets were mainly nuclear receptors and other proteins associated with carcinogenesis and cancer development. The results demonstrated the possible use of A. hispidum as a chemopreventive phytotherapeutic drug. However, further pharmacokinetics (PK) and toxicity studies are recommended to confirm the safety and bioavailability of the bioactive components.
Supplemental Material
Download MS Word (1 MB)Disclosure statement
No potential conflict of interest was reported by the authors.
Data availability statement
The dataset generated during and/or analysed during the current study are not publicly available but are available from the corresponding author on reasonable request.
Additional information
Funding
References
- Abdalla, A. N., Abdallah, M. E., Aslam, A., Bader, A., Vassallo, A., Tommasi, N. D., … El-Azab, A. S. (2020). Synergistic anti leukemia effect of a novel Hsp90 and a pan cyclin dependent kinase inhibitors. Molecules (Basel, Switzerland), 25(9), 2220. doi:10.3390/molecules25092220
- Abdalla, A. N., Malki, W. H., Qattan, A., Shahid, I., Hossain, M. A., & Ahmed, M. (2021). Chemosensitization of HT29 and HT29-5FU cell lines by a combination of a multi-tyrosine kinase inhibitor and 5FU downregulates ABCC1 and inhibits PIK3CA in light of their importance in saudi colorectal cancer. Molecules (Basel, Switzerland), 26(2), 334. doi:10.3390/molecules26020334
- Abdalla, A. N., Qattan, A., Malki, W. H., Shahid, I., Hossain, M. A., & Ahmed, M. (2020). Significance of targeting VEGFR-2 and cyclin D1 in luminal-A breast cancer. Molecules (Basel, Switzerland), 25(20), 4606. doi:10.3390/molecules25204606
- Adepiti, A., Adewunmi, C. O., & Agbedahunsi, J. (2014). Antitrichomonal activity of Acanthospermum hispidum D. C. (Asteraceae). African Journal of Biotechnology, 13(11), 1303–1307. doi:10.5897/AJB2013.13064
- Adukpo, S., Elewosi, D., Asmah, R., Nyarko, A., Ekpe, P., Adotei, D., & Ofori, M. (2020). Antiplasmodial and genotoxic study of selected Ghanaian medicinal plants. Evidence-Based Complementary and Alternative Medicine: ECAM, 2020, 1582724–1582710. doi:10.1155/2020/1582724
- Agamah, F. E., Mazandu, G. K., Hassan, R., Bope, C. D., Thomford, N. E., Ghansah, A., & ChIMusa, E. R. (2019). Computational/in silico methods in drug target and lead prediction. Briefings in Bioinformatics, 21(5), 1663–1675. %J Briefings in Bioinformatics doi:10.1093/bib/bbz103
- Anwar, M. M., Shalaby, M., Embaby, A. M., Saeed, H., Agwa, M. M., & Hussein, A. (2020). Prodigiosin/PU‑H71 as a novel potential combined therapy for triple negative breast cancer (TNBC): Preclinical insights. Scientific Reports, 10(1), 14706. doi:10.1038/s41598-020-71157-w
- Araújo, E., Randau, K., Filho, J., Pimentel, R., & Xavier, H. (2008). Acanthospermum hispidum DC (Asteraceae): Perspectives for a phytotherapeutic product. Revista Brasileira de Farmacognosia, 18, 777–784. doi:10.1590/S0102-695X2008000500024
- Arena, M. E., Cartagena, E., Gobbato, N., Baigori, M., Valdez, J. C., & Bardon, A. (2011). In vivo and in vitro antibacterial activity of acanthospermal B, a sesquiterpene lactone isolated from Acanthospermum hispidum. Phytotherapy Research: PTR, 25(4), 597–602. doi:10.1002/ptr.3300
- Awad, A. B., Chinnam, M., Fink, C. S., & Bradford, P. G. (2007). Beta-sitosterol activates Fas signaling in human breast cancer cells. Phytomedicine: International Journal of Phytotherapy and Phytopharmacology, 14(11), 747–754. doi:10.1016/j.phymed.2007.01.003
- Awad, A. B., & Fink, C. S. (2000). Phytosterols as anticancer dietary components: Evidence and mechanism of action. The Journal of Nutrition, 130(9), 2127–2130. doi:10.1093/jn/130.9.2127
- Awad, A. B., von Holtz, R. L., Cone, J. P., Fink, C. S., & Chen, Y. C. (1998). Beta-sitosterol inhibits growth of HT-29 human colon cancer cells by activating the sphingomyelin cycle. Anticancer Research, 18(1a), 471–473.
- Azeez, T., & Banigo, A. (2018). Phytochemical analysis of aqueous methanolic extract of Acanthospermum hispidium and its effect on biochemical and hematological indices in Plasmodium falciparum infected rats. African Journal of Biomedical Research, 21, 183–192.
- Bao, X., Zhang, Y., Zhang, H., & Xia, L. (2022). Molecular mechanism of β-sitosterol and its derivatives in tumor progression. Frontiers in Oncology, 12, 926975. doi:10.3389/fonc.2022.926975
- Baskar, A. A., Ignacimuthu, S., Paulraj, G. M., & Al Numair, K. S. (2010). Chemopreventive potential of beta-sitosterol in experimental colon cancer model – an in vitro and in vivo study. BMC Complementary and Alternative Medicine, 10(1), 24. doi:10.1186/1472-6882-10-24
- Berdis, A. J. (2017). Inhibiting DNA polymerases as a therapeutic intervention against cancer. Frontiers in Molecular Biosciences, 4, 78. doi:10.3389/fmolb.2017.00078
- Bilotta, M. T., Petillo, S., Santoni, A., & Cippitelli, M. (2020). Liver X receptors: Regulators of cholesterol metabolism, inflammation, autoimmunity, and cancer. Frontiers in Immunology, 11, 584303. doi:10.3389/fimmu.2020.584303
- Cai, C., Zhang, Y., & Peng, X. (2021). Knocking down sterol regulatory element binding protein 2 (SREBF2) inhibits the serine protease 8 (PRSS8)/sodium channel epithelial 1alpha subunit (SCNN1A) axis to reduce the cell proliferation, migration and epithelial-mesenchymal transformation of ovarian cancer. Bioengineered, 12(2), 9390–9400. doi:10.1080/21655979.2021.1978615
- Cartagena, E., Bardón, A., Catalán, C. A., de Hernández, Z. N., Hernández, L. R., & Joseph-Nathan, P. (2000). Germacranolides and a new type of guaianolide from Acanthospermum hispidum. Journal of Natural Products, 63(10), 1323–1328. doi:10.1021/np9905057
- Cassileth, B. R., & Deng, G. (2004). Complementary and alternative therapies for cancer. The Oncologist, 9(1), 80–89. doi:10.1634/theoncologist.9-1-80
- Celikler Ozer, O., Orhan, I. E., Çalışkan, B., Senol Deniz, F. S., Gokbulut, A., Gur Maz, T., … Banoglu, E. (2021). Exploration of anti-tyrosinase effect of Geranium glaberrimum Boiss. & Heldr. with in silico approach and survey of 21 Geranium species. Journal of Herbal Medicine, 27, 100431. doi:10.1016/j.hermed.2021.100431
- Chakraborty, A. K., Gaikwad, A., & Singh, K. (2012). Phytopharmacological review on Acanthospermum hispidum. Journal of Applied Pharmaceutical Science, 2(1), 144–148.
- Chandrasekar, R., Sivagami, B., & Babu, M. N. (2018). A pharmacoeconomic focus on medicinal plants with anticancer activity. Research Journal of Pharmacognosy and Phytochemistry, 10(1), 91. doi:10.5958/0975-4385.2018.00015.8
- Chen, P., Li, B., & Ou-Yang, L. (2022). Role of estrogen receptors in health and disease. Frontiers in Endocrinology, 13, 839005. doi:10.3389/fendo.2022.839005
- Daina, A., Michielin, O., & Zoete, V. (2017). SwissADME: A free web tool to evaluate pharmacokinetics, drug-likeness and medicinal chemistry friendliness of small molecules. Scientific Reports, 7(1), 42717. doi:10.1038/srep42717
- Daina, A., Michielin, O., & Zoete, V. (2019). SwissTargetPrediction: Updated data and new features for efficient prediction of protein targets of small molecules. Nucleic Acids Research, 47(W1), W357–W364. doi:10.1093/nar/gkz382
- Deepa, N., & Rajendran, N. N. (2007). Anti-tumor activity of Acanthospermum hispidum DC on dalton ascites lymphoma in mice. Natural Product Sciences, 13, 234–240.
- Edewor, T., & Olajire, A. (2011). Two flavones from Acanthospermum hispidum DC and their antibacterial activity. Journal of Organic Chemistry, 01, 132–141. doi:10.4236/ijoc.2011.13020
- Enmark, E., Pelto-Huikko, M., Grandien, K., Lagercrantz, S., Lagercrantz, J., Fried, G., … Gustafsson, J. A. (1997). Human estrogen receptor beta-gene structure, chromosomal localization, and expression pattern. The Journal of Clinical Endocrinology and Metabolism, 82(12), 4258–4265. doi:10.1210/jcem.82.12.4470
- Erwin, Pusparohmana, W. R., Safitry, R. D., Marliana, E., Usman, & Kusuma, I. W. (2020). Isolation and characterization of stigmasterol and β-sitosterol from wood bark extract of Baccaurea macrocarpa Miq. Mull. Arg. Rasayan Journal of Chemistry. 13(04), 2552–2558. doi:10.31788/RJC.2020.1345652
- Filimonov, D. A., Poroĭkov, V. V., Karaicheva, E. I., Kazarian, R. K., Budunova, A. P., Mikhaĭlovskiĭ, E. M., … Burov, I. V. (1995). The computerized prediction of the spectrum of biological activity of chemical compounds by their structural formula: The PASS system. Prediction of activity spectra for substance. Eksperimental’naia i Klinicheskaia Farmakologiia, 58(2), 56–62.
- Fuentes, N., & Silveyra, P. (2019). Estrogen receptor signaling mechanisms. Advances in protein chemistry and structural biology, 116, 135–170. doi:10.1016/bs.apcsb.2019.01.001
- Gad, E., Nafie, M., Eltamany, E., Hammad, M., Barakat, A., & Boraei, A. (2020). Discovery of new apoptosis-inducing agents for breast cancer based on ethyl 2-amino-4,5,6,7-tetra hydrobenzo[b]thiophene-3-carboxylate: Synthesis, in vitro, and in vivo activity evaluation. Molecules (Basel, Switzerland), 25(11), 2523. doi:10.3390/molecules25112523
- Gezici, S., & Şekeroğlu, N. (2019). Current perspectives in the application of medicinal plants against cancer: Novel therapeutic agents. Anti-Cancer Agents in Medicinal Chemistry, 19(1), 101–111. doi:10.2174/1871520619666181224121004
- Karar, M. G. E., & Kuhnert, N. (2017). Herbal drugs from Sudan: Traditional uses and phytoconstituents. Pharmacognosy Reviews, 11(22), 83–103. doi:10.4103/phrev.phrev_15_15
- Koukouikila-Koussounda, F., Abena, A. A., Nzoungani, A., Mombouli, J. V., Ouamba, J. M., Kun, J., & Ntoumi, F. (2013). In vitro evaluation of antiplasmodial activity of extracts of Acanthospermum hispidum DC (Asteraceae) and Ficus thonningii Blume (Moraceae), two plants used in traditional medicine in the Republic of Congo. African Journal of Traditional, Complementary, and Alternative Medicines: AJTCAM, 10(2), 270–276.
- Lu, J. J., Bao, J. L., Chen, X. P., Huang, M., & Wang, Y. T. (2012). Alkaloids isolated from natural herbs as the anticancer agents. Evidence-Based Complementary and Alternative Medicine: ECAM, 2012, 485042–485012. doi:10.1155/2012/485042
- Michmerhuizen, A. R., Spratt, D. E., Pierce, L. J., & Speers, C. W. (2020). Are we there yet? Understanding androgen receptor signaling in breast cancer. NPJ Breast Cancer, 6(1), 47. doi:10.1038/s41523-020-00190-9
- N'Do, J. Y., Hilou, A., Ouedraogo, N., Sombie, E. N., & Traore, T. K. (2018). Phytochemistry, antioxidant, and hepatoprotective potential of Acanthospermum hispidum DC extracts against diethylnitrosamine-induced hepatotoxicity in rats. Medicines (Basel, Switzerland), 5(2), 42. doi:10.3390/medicines5020042
- Ogbole, O. O., Segun, P. A., & Adeniji, A. J. (2017). In vitro cytotoxic activity of medicinal plants from Nigeria ethnomedicine on Rhabdomyosarcoma cancer cell line and HPLC analysis of active extracts. BMC Complementary and Alternative Medicine, 17(1), 494. doi:10.1186/s12906-017-2005-8
- Oh, T. G., Wang, S.-C. M., Acharya, B. R., Goode, J. M., Graham, J. D., Clarke, C. L., … Muscat, G. E. O. (2016). The nuclear receptor, RORγ, regulates pathways necessary for breast cancer metastasis. EBioMedicine, 6, 59–72. doi:10.1016/j.ebiom.2016.02.028
- Olawumi, O., Oladosu, I., & Christianah, F. (2014). Tetrahydroanthracene derivative: Anti-microbial isolate from Acanthospermum hispidum DC. International Journal of Advanced Chemistry, 2(2), 182–184. doi:10.14419/ijac.v2i2.3519
- Pierre, L. L., & Moses, M. N. (2015). Isolation and characterisation of stigmasterol and B-sitosterol from Odontonema Strictum (Acanthaceae). JIPBS, 2(1), 88–96.
- Poli, A., Marangoni, F., Corsini, A., Manzato, E., Marrocco, W., Martini, Da. n. iela., … Visioli, F. (2021). Phytosterols, cholesterol control, and cardiovascular disease. Nutrients, 13(8), 2810. doi:10.3390/nu13082810
- Pontini, L., & Marinozzi, M. (2021). Shedding light on the roles of liver X receptors in cancer by using chemical probes. British Journal of Pharmacology, 178(16), 3261–3276. doi:10.1111/bph.15200
- Santos, E., Machado, J., Ferreira, M., & Soares, L. (2022). Acanthospermum hispidum DC: An updated review on phytochemistry and biological activities. Mini Reviews in Medicinal Chemistry, 22(5), 684–700. doi:10.2174/1389557521666210913115651
- Schattner, E., & Friedman, S. M. (1996). Fas expression and apoptosis in human B cells. Immunologic Research, 15(3), 246–257. doi:10.1007/BF02918252
- Shwe, H. H., Thein, W. W., Win, S. S., Pe, N. N., & Win, T. (2019). Structural characterization of stigmasterol and β-sitosterol from the roots of Premna herbacea Roxb. IEEE-SEM, 7(8), 195–201.
- Soleimanian, Y., Goli, S. A. H., Varshosaz, J., Di Cesare Mannelli, L., Ghelardini, C., Cirri, M., & Maestrelli, F. (2020). β-sitosterol loaded nanostructured lipid carrier: Physical and oxidative stability, in vitro simulated digestion and hypocholesterolemic activity. Pharmaceutics, 12(4), 386. doi:10.3390/pharmaceutics12040386
- Sultana, S., Chishti, A., Muhammad, S., Ali Shah, D. S., Akram, M., & Nisar, J. (2019). Complementary and alternative approach to heal cancer: A review. Pakistan Journal of Biological Science. 2, 32–41.
- Turdo, A., D'Accardo, C., Glaviano, A., Porcelli, G., Colarossi, C., COLarossi, L., … Stassi, G. (2021). Targeting phosphatases and kinases: How to checkmate cancer. Frontiers in Cell and Developmental Biology, 9, 690306. doi:10.3389/fcell.2021.690306
- Yarmolinsky, J., Bull, C. J., Vincent, E. E., Robinson, J., Walther, A., Smith, G. D., … Martin, R. M. (2020). Association between genetically proxied inhibition of HMG-CoA reductase and epithelial ovarian cancer. JAMA, 323(7), 646–655. doi:10.1001/jama.2020.0150
- Zhang, M., Wu, S., Wang, L., Xia, Z., Kuang, K., Xu, Q., … Zhou, N. (2022). Visible-light-induced cascade cyclization of N-propargyl aromatic amines and acyl oxime esters: Rapid access to 3-acylated quinolines. The Journal of Organic Chemistry, 87(15), 10277–10284. doi:10.1021/acs.joc.2c01277
- Zhang, R., Zeng, J., Liu, W., Meng, J., Wang, C., Shi, L., … Xing, D. (2022). The role of NPC1L1 in cancer. Frontiers in Pharmacology, 13, 956619. doi:10.3389/fphar.2022.956619
- Zhao, M., Ma, J., Li, M., Zhang, Y., Jiang, B., Zhao, X., … Qin, S. (2021). Cytochrome P450 enzymes and drug metabolism in humans. International Journal of Molecular Sciences, 22(23), 12808. doi:10.3390/ijms222312808