Abstract
Antibiotics are widely prescribed for mothers in the peripartum period today. Approximately 40% of pregnant women at term are exposed to antibiotics. Antibiotics are useful against infectious conditions such as chorioamnionitis; however, they alter the maternal microbiome. The maternal microbiome, particularly the gut microbiome, is transmitted to their neonates and is one of the major sources that shape the child's gut microbiome. The gut microbiome early in life plays a crucial role in the development of the gut microbiome itself as well as the host health over the entire life. Microbes structure the commensal ecosystem in the host. Simultaneously, microbial components and metabolites influence the host organ functions including the immune system, and vice versa, the various factors of the host impact the microbiome. The alterations of the gut microbiome induced by antibiotics in mothers can lead to gut dysbiosis in children eventually resulting in chronic disease conditions including immune disorders. Knowledge of the lasting impacts of maternal peripartum exposure to antibiotics on the gut microbiome and health in offspring and reconsideration of the adequate use of antibiotics in clinical practice are needed. Avoiding and restoring neonatal dysbiosis following maternal antibiotics-induced dysbiosis could be a new preventive strategy for various diseases.
1. Introduction
The progress of genome sequencing technology, bioinformatics, and multi-omic technologies has extended our understanding of the human gut microbiome. These advances have revealed that the gut microbiome plays an important role in human health and its imbalance, dysbiosis, is associated with diseases [Citation1]. Interactions between the host and the gut microbiome are crucial for the healthy development of the host. The gut microbiome early in life plays critical roles in the development of host immune systems [Citation2,Citation3] as well as metabolism and other organ functions [Citation4,Citation5] and is thought to influence the host health over the entire life [Citation6]. Recent epidemiological studies have shown the association between the use of antibiotics early in life with the increased risk of various diseases such as obesity [Citation7–10], food allergy [Citation11], atopic disorders [Citation11], asthma [Citation12], inflammatory bowel disease [Citation13–15], celiac disease [Citation16], juvenile idiopathic arthritis [Citation17]. Given that antibiotics can alter the gut microbiome, these findings suggest that dysbiosis early in life may have causative effects in the development of the diseases later in life.
The human gut microbiome develops early in life. This development process is affected by various factors, such as maternal microbiome [Citation18], mode of delivery [Citation19–21], feeding practice/diet [Citation22,Citation23], diet supplements [Citation22,Citation24], probiotics [Citation25], antibiotics [Citation26], environmental factors (e.g. living area, hygiene, lifestyle, and so on) [Citation26,Citation27], and genetic background [Citation28,Citation29]. Peripartum maternal microbiome is one of the key factors that shape the gut microbiome in children. Antibiotics are widely used for women in the peripartum period, especially in developed countries [Citation30]. Peripartum antibiotics for mothers can influence the development of the child’s gut microbiome via antibiotics-induced alterations of the maternal vaginal and gut microbiome. This antibiotic-perturbed microbiome in a child, in turn, could have unfavorable health effects in the future.
Here we review the contribution of the maternal microbiome to the development of the child gut microbiome and the impact of maternal exposure to antibiotics on the microbiome development process of neonates and health later in life.
2. Maternal exposure to antibiotics during peripartum period in the current clinical setting
Antibiotics are needed for infectious diseases, particularly bacterial infections, including during pregnancy. Today, antibiotics are widely prescribed for mothers and neonates during the peripartum period, especially in the developed countries. There are data that approximately 40% of pregnant women at term and more than 30% of neonates are exposed to antibiotics [Citation31,Citation32]. During pregnancy, broad-spectrum antibiotics, such as cephalosporins, have become prescribed more frequently in the developed countries [Citation33,Citation34]. During pregnancy and labor, obstetrical antibiotic indications include chorioamnionitis, prevention of maternal infections after cesarean section, prevention of preterm birth, and prevention of Group B streptococcus (GBS) diseases in neonates [Citation30]. However, while maternal infections and inflammation lead to the increased risk of preterm birth, it has not been established whether antibiotic therapy can prevent it [Citation35]. A randomized trial showed that antibiotic treatment for the prevention of preterm birth might be even harmful leading to functional impairments and increased risk of [Citation36]. GBS is one of the leading causes of neonatal infections [Citation30]. Maternal intrapartum GBS colonization is the primary risk factor for neonatal GBS early-onset disease that occurs within the first week after birth. Intrapartum antibiotic prophylaxis (IAP) for GBS-colonized women decreases the risk of GBS transmission to their neonates and the risk of neonatal GBS infections [Citation37]. Meanwhile, it remains an important challenge to establish a risk-based strategy for screening subjects for IAP to avoid unnecessary prescriptions [Citation38,Citation39].
3. Transmission of the maternal microbiome to neonates and its impacts on the infant gut microbiome
The maternal microbiome is vertically transmitted from mothers to their children [Citation18]. With metagenomic shotgun sequencing and advanced bioinformatics technologies, now this process is confirmed at the strain level [Citation20,Citation40]. The maternal microbiome in the gut as well as other body sites influences the child microbiome. Ferretti et al. reported that the maternal microbiome of the gut, vagina, oral cavity, and skin contribute to structuring the infant gut microbiome accounting for 22.1%, 16.3%, 7.2%, and 5.0%, respectively [Citation41]. Although it remains controversial, several recent studies describe that the maternal microbiome starts to transmit to the fetus in the uterus [Citation42–45]. The difference in the mode of delivery impacts this vertical microbiome transmission process. The gut microbiome is different between vaginal-delivered neonates and those delivered by cesarean section [Citation19–21]. Meanwhile, when cesarean-born infants were exposed to maternal vaginal fluids, their microbiome became partially similar with vaginal-delivered infants [Citation46]. The transmitted maternal microbiome is thought to be dominant in neonates particularly during the first few days. Ferretti et al. reported that 50.7% of the bacterial species in the child gut microbiome on the day of birth were derived from their mothers [Citation41]. Bäckhed et al. showed that the vaginal-delivered infants shared up to 72% of species with their mothers within 2-5 days after birth [Citation47].
After birth, neonates are exposed to various environmental factors which may influence the microbiome [Citation26,Citation27]. Feeding practice is one of these factors that influence the gut microbiome development. It has been reported that the microbiome of exclusively breast-fed infants is different from those with non-exclusive breastfeeding [Citation48]. The infant gut microbe develops dynamically early in life while being influenced by internal (e.g. genetics) and external (e.g. growing environment) factors and is thought to be almost completely established by around 3 years old [Citation49,Citation50] (). The microbes derived from mothers are relatively stable in the infant's gut over months [Citation41]. Microbes in the gut share the niche and biological resources and the timing and order of colonization can be crucial for establishing the microbial community. In an animal model, it has been shown that the microbes colonize early in life contributes to determining the eventual gut microbiome structure [Citation51,Citation52]. These findings suggest that maternal antibiotics-induced dysbiosis can transmit to their children and maternal dysbiosis has lasting impacts on the development and establishment of the gut microbiome in children.
Figure 1. Development of the gut microbiome in children. The maternal microbiome is one of the significant factors that shape the neonatal gut microbiome. Various internal and external factors influence the development process of the microbial community. The gut microbiome reaches the adult-like structure at around three years old.
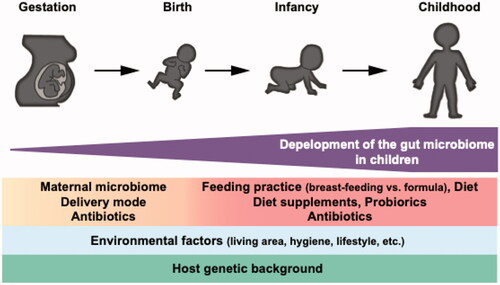
Several studies demonstrated that IAP affects the neonatal gut microbiome structure [Citation53–59]. Aloisio et al. compared the abundance of Lactobacillus spp., Bifidobacterium spp., Bacteroides fragilis group, Clostridiodis difficile, and Escherichia coli using real-time PCR between newborns at 6–7th days after birth from GBS-negative mothers and those from mothers treated with ampicillin as IAP. They showed that Bifidobacterium was significantly decreased [Citation53]. They performed another study employing 16S rRNA gene amplicon sequencing analysis and demonstrated a reduced bacterial diversity with a predominance of Enterobacteriaceae and a significant decrease of Bifidobacterium in infants from mothers with IAP for GBS compared to the healthy control [Citation54]. Corvaglia et al. employed real-time PCR and showed that Bifidobacterium significantly decreased in infants from mothers who received ampicillin as IAP for GBS on the 7th day after birth but there was no difference between the IAP group and the control at 30th day [Citation55]. In another Italian cohort, Mazzola et al. performed 16S rRNA gene amplicon sequencing and reported that infants from mothers with IAP of ampicillin for GBS presented a lower relative abundance of Bifidobacterium and higher relative abundance of Enterobacteriaceae compared to the control at 7th day after birth, while the Bifidobacterium recovered in the IAP group by 30th day. Nogacka et al. analyzed infants from mothers who received penicillin as IAP for GBS with 16S rRNA and demonstrated that the IAP group showed the different gut bacterial compositions from the control within the first weeks of life, with the lower relative abundance of Actinobacteria and Bacteroidetes and higher relative abundance of Proteobacteria and Firmicutes. The differences almost disappeared by 90 days of age. Interestingly, they also showed that IAP affected the levels of short-chain fatty acids (SCFA) in the infant feces [Citation57]. This finding suggests that IAP impacts on the gut microbiome structure as well as metabolic functions. Stearns et al. showed less bacterial diversity, a lower relative abundance of Bifidobacterium, and a higher abundance of Escherichia and Clostridium in infants from mothers with IAP of penicillin for GBS over the first 12 weeks, while the differences became less apparent by 12 weeks of age. Meanwhile, in the infants from mothers with IAP due to cesarean section, the alterations were evident persistently [Citation58]. The differences in the delivery mode, as well as the type of antibiotics, need to be taken into account when interpreting this result. In a large cohort study in Canada, Azad et al. analyzed the gut microbiome of 198 infants with 16S rRNA amplicon sequencing [Citation59]. The indications of IAP included GBS, the pre-labor rupture of membrane, and cesarean section in the cohort. They demonstrated that compositions of the gut microbiome in children with IAP were different from the control at 3 months after birth; less diversity, fewer Bacteroides and Parabacteroides, and more Enterococcus and Clostridium in the IAP group. The difference in the gut microbiome structure persisted up to 12 months, particularly in children with IAP for emergency cesarean section.
These studies support the notion that the influence of antibiotics administration in peripartum period on the neonatal microbiome needs to be recognized.
4. Maternal antibiotics exposure can increase the risk of diseases in children later in life
The gut microbiome early in life is now thought to have a crucial, lasting impact on human immune system health throughout life [Citation60,Citation61]. Various studies using animal models support this notion. For example, colonization of E. coli during pregnancy impacts on the intestinal mucosal innate immunity in offspring via aryl hydrocarbon receptor (AhR) leading to the increase of intestinal group 3 innate lymphoid (ILC3) cells and F4/80+ CD11c+ mononuclear cells in offspring [Citation62]. Glycosphingolipids of B. fragilis early in life play an important role in establishing the homeostasis of host invariant natural killer T (iNKT) cells [Citation63]. SCFA plays a role in regulating Tregs through the inhibition of histone deacetylases (HDAC) [Citation64,Citation65]. Thorburn et al. demonstrated that maternal consumption of a high-fiber diet alters the gut microbiome and promotes intestinal SCFA production, such as acetate, leading to an increased potential to induce regulatory T cells (Tregs) in the pups of a murine asthma model [Citation66]. A recent study by Al Nabhani et al. demonstrated the interactions between the host and gut microbiome in the weaning period are essential for the development of RORγt + Tregs and this process is crucial for host health in adulthood [Citation67]. Thus, the gut microbiome early in life plays an important role in the healthy development of both innate and adaptive immunity of the host. Missing the appropriate immunological education due to gut dysbiosis in the critical window period early in life could lead to aberrant immune development eventually resulting in immunological disorders later in life. Given maternal dysbiosis can be transmitted to children as mentioned above, maternal antibiotics-induced dysbiosis could lead to diseases in offspring.
Recent human epidemiological studies have demonstrated the association between maternal exposure to antibiotics in the peripartum period and the increased risk of diseases in children. A study in Denmark demonstrated that maternal exposure to antibiotics during pregnancy increased the risk of asthma in children [Citation68]. The analysis in a European birth cohort study showed that antibiotic exposure in utero was associated with atopic dermatitis and food allergy of children [Citation11]. A cohort study in Sweden reported the increased risk of inflammatory bowel diseases (IBD) in children with exposure to antibiotics during pregnancy [Citation15]. Clinical studies are limited in their ability to establish a causal link between maternal antibiotics-induced dysbiosis and diseases in children due to large differences in the inter-individual gut microbiome, challenges in controlling for confounding variables, and constraints of the observational clinical design. It is crucial to determine if maternal exposure to antibiotics during the peripartum period has a causative effect in the diseases via the child's gut dysbiosis for understanding the underlying pathophysiology and for developing preventive and therapeutic strategies. For this purpose, translational research using animal models can provide scientific merits. Alhasan et al. [Citation69] developed a mouse asthma model in which maternal antibiotic exposure during pregnancy increases the susceptibility to asthma in offspring. They showed that the antibiotic treatment caused gut dysbiosis and decreased levels of SCFA in offspring. These observations support the findings in human subjects [Citation70]. This animal model would be helpful to obtain further mechanistic insights on the changes in the immune profile of the offspring and the development of asthma caused by maternal exposure to antibiotics during pregnancy. Miyoshi et al. [Citation71] reported a mouse model that presents gut dysbiosis, the aberrant immune development, and the development of colitis in offspring with maternal peripartum antibiotic exposure. They demonstrated that the maternal antibiotic-induced dysbiosis was transmitted to their pups and showed a causal link between gut dysbiosis and the pro-inflammatory immune development in offspring. Schulfer et al. also reported that maternal perturbed microbiome by the antibiotic exposure promotes susceptibility to colitis in offspring [Citation72]. These models would be useful to investigate pathoetiology of IBD.
5. Conclusions
The maternal dysbiosis induced by peripartum exposure to antibiotics is transmitted to their children and has lasting impacts on the development of the child's gut microbiome. This process eventually can influence the health condition of children later in life. While antibiotics administration is crucial for some medical situations, recent evidence underscores the importance to carefully consider the use of antibiotics. It also suggests that the interventions approaching gut dysbiosis early in life can be future preventive strategies for various diseases.
Acknowledgment
The authors thank Dr. Mark W. Musch for his English proofreading.
Disclosure statement
The authors declare no conflict of interest.
References
- Carding S, Verbeke K, Vipond DT, et al. Dysbiosis of the gut microbiota in disease. Microb Ecol Health Dis. 2015;26:26191.
- Gensollen T, Iyer SS, Kasper DL, et al. How colonization by microbiota in early life shapes the immune system. Science. 2016;352(6285):539–544.
- Al Nabhani Z, Eberl G. Imprinting of the immune system by the microbiota early in life. Mucosal Immunol. 2020;13(2):183–189.
- Neuman H, Forsythe P, Uzan A, et al. Antibiotics in early life: dysbiosis and the damage done. FEMS Microbiol Rev. 2018;42(4):489–499.
- Robertson RC, Manges AR, Finlay BB, et al. The human microbiome and child Growth - First 1000 days and Beyond. Trends Microbiol. 2019;27(2):131–147.
- Ihekweazu FD, Versalovic J. Development of the pediatric gut microbiome: Impact on health and disease. Am J Med Sci. 2018;356(5):413–423.
- Trasande L, Blustein J, Liu M, et al. Infant antibiotic exposures and early-life body mass. Int J Obes. 2013;37(1):16–23.
- Bailey LC, Forrest CB, Zhang P, et al. Association of antibiotics in infancy with early childhood obesity. JAMA Pediatr. 2014;168(11):1063–1069.
- Poulsen MN, Pollak J, Bailey-Davis L, et al. Associations of prenatal and childhood antibiotic use with child body mass index at age 3 years. Obesity (Silver Spring. 2017;25(2):438–444.
- Cassidy-Bushrow AE, Burmeister C, Havstad S, et al. Prenatal antimicrobial use and early-childhood body mass index. Int J Obes (Lond)). 2018;42(1):1–7.
- Metzler S, Frei R, Schmaußer-Hechfellner E, PASTURE/EFRAIM study group, et al. Association between antibiotic treatment during pregnancy and infancy and the development of allergic diseases. Pediatr Allergy Immunol. 2019;30(4):423–433.
- Zou Z, Liu W, Huang C, et al. First-Year antibiotics exposure in relation to childhood asthma, allergies, and airway illnesses. Int J Environ Res Public Health. 2020;17:5700.
- Kronman MP, Zaoutis TE, Haynes K, et al. Antibiotic exposure and IBD development among children: a population-based cohort study. Pediatrics. 2012;130(4):e794–e803.
- Ungaro R, Bernstein CN, Gearry R, et al. Antibiotics associated with increased risk of new-onset crohn's disease but not ulcerative colitis: a meta-analysis. Am J Gastroenterol. 2014;109(11):1728–1738.
- Ortqvist AK, Lundholm C, Halfvarson J, et al. Fetal and early life antibiotics exposure and very early onset inflammatory bowel disease: a population-based study. Gut. 2019;68(2):218–225.
- Marild K, Ye W, Lebwohl B, et al. Antibiotic exposure and the development of coeliac disease: a nationwide case-control study. BMC Gastroenterol. 2013;13:109.
- Horton DB, Scott FI, Haynes K, et al. Antibiotic exposure and juvenile idiopathic arthritis: a Case-Control study. Pediatrics. 2015;136(2):e333–e343.
- Wang S, Ryan CA, Boyaval P, et al. Maternal vertical transmission affecting early-life microbiota Development. Trends Microbiol. 2020;28(1):28–45.
- Chu DM, Ma J, Prince AL, et al. Maturation of the infant microbiome community structure and function across multiple body sites and in relation to mode of delivery. Nat Med. 2017;23(3):314–326.
- Wampach L, Heintz-Buschart A, Fritz JV, et al. Birth mode is associated with earliest strain-conferred gut microbiome functions and immunostimulatory potential. Nat Commun. 2018;9(1):5091.
- Shao Y, Forster SC, Tsaliki E, et al. Stunted microbiota and opportunistic pathogen colonization in caesarean-section birth. Nature. 2019;574(7776):117–121.
- Nielsen S, Nielsen DS, Lauritzen L, et al. Impact of diet on the intestinal microbiota in 10-month-old infants. J Pediatr Gastroenterol Nutr. 2007;44(5):613–618.
- Krebs NF, Sherlock LG, Westcott J, et al. Effects of different complementary feeding regimens on iron status and enteric microbiota in breastfed infants. J Pediatr. 2013;163(2):416–423.
- Andersen AD, Molbak L, Michaelsen KF, et al. Molecular fingerprints of the human fecal microbiota from 9 to 18 months old and the effect of fish oil supplementation. J Pediatr Gastroenterol Nutr. 2011;53(3):303–309.
- Navarro-Tapia E, Sebastiani G, Sailer S, et al. Probiotic supplementation during the perinatal and infant period: Effects on gut dysbiosis and disease. Nutrients. 2020;12:2243.
- Tamburini S, Shen N, Wu HC, et al. The microbiome in early life: implications for health outcomes. Nat Med. 2016;22(7):713–722.
- Gupta VK, Paul S, Dutta C. Geography, ethnicity or Subsistence-Specific variations in human microbiome composition and diversity. Front Microbiol. 2017;8:1162.
- Goodrich JK, Waters JL, Poole AC, et al. Human genetics shape the gut microbiome. Cell. 2014;159(4):789–799.
- Blekhman R, Goodrich JK, Huang K, et al. Host genetic variation impacts microbiome composition across human body sites. Genome Biol. 2015;16:191.
- Martinez de Tejada B. Antibiotic use and misuse during pregnancy and delivery: benefits and risks. Int J Environ Res Public Health. 2014;11(8):7993–8009.
- Stokholm J, Schjorring S, Pedersen L, et al. Prevalence and predictors of antibiotic administration during pregnancy and birth. PLoS One. 2013;8(12):e82932.
- Broe A, Pottegard A, Lamont RF, et al. Increasing use of antibiotics in pregnancy during the period 2000-2010: prevalence, timing, category, and demographics. BJOG. 2014;121(8):988–996.
- Petersen I, Gilbert R, Evans S, et al. Oral antibiotic prescribing during pregnancy in primary care: UK population-based study. J Antimicrob Chemother. 2010;65(10):2238–2246.
- Palmsten K, Hernandez-Diaz S, Chambers CD, et al. The most commonly dispensed prescription medications among pregnant women enrolled in the U.S. Medicaid program. Obstet Gynecol. 2015;126(3):465–473.
- Kenyon SL, Taylor DJ, Tarnow-Mordi W. Broad-spectrum antibiotics for spontaneous preterm labour: the ORACLE II randomised trial. ORACLE collaborative group. Lancet. 2001;357(9261):989–994.
- Kenyon S, Pike K, Jones DR, et al. Childhood outcomes after prescription of antibiotics to pregnant women with spontaneous preterm labour: 7-year follow-up of the ORACLE II trial. Lancet. 2008;372(9646):1319–1327.
- Baltimore RS, Huie SM, Meek JI, et al. Early-onset neonatal sepsis in the era of group B streptococcal prevention. Pediatrics. 2001;108(5):1094–1098.
- Lyytikainen O, Nuorti JP, Halmesmaki E, et al. Invasive group B streptococcal infections in Finland: a population-based study. Emerg Infect Dis. 2003;9(4):469–473.
- Heath PT, Balfour G, Weisner AM, et al. Group B streptococcal disease in UK and irish infants younger than 90 days. Lancet. 2004;363(9405):292–294.
- Yassour M, Jason E, Hogstrom LJ, et al. Strain-Level analysis of mother-to-Child bacterial transmission during the first few months of life. Cell Host Microbe. 2018;24(1):146–154 e4.
- Ferretti P, Pasolli E, Tett A, et al. Mother-to-Infant microbial transmission from different body sites shapes the developing infant gut microbiome. Cell Host Microbe. 2018;24(1):133–145. e5.
- Aagaard K, Ma J, Antony KM, et al. The placenta harbors a unique microbiome. Sci Transl Med. 2014;6(237):237ra65–237ra65.
- Dong XD, Li XR, Luan JJ, et al. Bacterial communities in neonatal feces are similar to mothers' placentae. Can J Infect Dis Med Microbiol. 2015;26(2):90–94.
- Hansen R, Scott KP, Khan S, et al. First-Pass meconium samples from healthy term Vaginally-Delivered neonates: an analysis of the microbiota. PLoS One. 2015;10(7):e0133320.
- Collado MC, Rautava S, Aakko J, et al. Human gut colonisation may be initiated in utero by distinct microbial communities in the placenta and amniotic fluid. Sci Rep. 2016;6:23129.
- Dominguez-Bello MG, De Jesus-Laboy KM, Shen N, et al. Partial restoration of the microbiota of cesarean-born infants via vaginal microbial transfer. Nat Med. 2016;22(3):250–253.
- Backhed F, Roswall J, Peng Y, et al. Dynamics and stabilization of the human gut microbiome during the first year of life. Cell Host Microbe. 2015;17(6):852.
- Stewart CJ, Ajami NJ, O'Brien JL, et al. Temporal development of the gut microbiome in early childhood from the TEDDY study. Nature. 2018;562(7728):583–588.
- Yatsunenko T, Rey FE, Manary MJ, et al. Human gut microbiome viewed across age and geography. Nature. 2012;486(7402):222–227.
- Rodriguez JM, Murphy K, Stanton C, et al. The composition of the gut microbiota throughout life, with an emphasis on early life. Microb Ecol Health Dis. 2015;26:26050.
- Martinez I, Maldonado-Gomez MX, Gomes-Neto JC, et al. Experimental evaluation of the importance of colonization history in early-life gut microbiota assembly. Elife. 2018;7:e36521.
- Sprockett D, Fukami T, Relman DA. Role of priority effects in the early-life assembly of the gut microbiota. Nat Rev Gastroenterol Hepatol. 2018;15(4):197–205.
- Aloisio I, Mazzola G, Corvaglia LT, et al. Influence of intrapartum antibiotic prophylaxis against group B Streptococcus on the early newborn gut composition and evaluation of the anti-Streptococcus activity of bifidobacterium strains. Appl Microbiol Biotechnol. 2014;98(13):6051–6060.
- Aloisio I, Quagliariello A, De Fanti S, et al. Evaluation of the effects of intrapartum antibiotic prophylaxis on newborn intestinal microbiota using a sequencing approach targeted to multi hypervariable 16S rDNA regions. Appl Microbiol Biotechnol. 2016;100(12):5537–5546.
- Corvaglia L, Tonti G, Martini S, et al. Influence of intrapartum antibiotic prophylaxis for group B Streptococcus on gut microbiota in the first month of life. J Pediatr Gastroenterol Nutr. 2016;62(2):304–308.
- Mazzola G, Murphy K, Ross RP, et al. Early gut microbiota perturbations following intrapartum antibiotic prophylaxis to prevent group B streptococcal disease. PLoS One. 2016;11(6):e0157527.
- Nogacka A, Salazar N, Suarez M, et al. Impact of intrapartum antimicrobial prophylaxis upon the intestinal microbiota and the prevalence of antibiotic resistance genes in vaginally delivered full-term neonates. Microbiome. 2017;5(1):93.
- Stearns JC, Simioni J, Gunn E, et al. Intrapartum antibiotics for GBS prophylaxis alter colonization patterns in the early infant gut microbiome of low risk infants. Sci Rep. 2017;7(1):16527.
- Azad MB, Konya T, Persaud RR, CHILD Study Investigators, et al. Impact of maternal intrapartum antibiotics, method of birth and breastfeeding on gut microbiota during the first year of life: a prospective cohort study. BJOG. 2016;123(6):983–993.
- Vangay P, Ward T, Gerber JS, et al. Antibiotics, pediatric dysbiosis, and disease. Cell Host Microbe. 2015;17(5):553–564.
- Renz H, Adkins BD, Bartfeld S, et al. The neonatal window of opportunity-early priming for life. J Allergy Clin Immunol. 2018;141(4):1212–1214.
- Gomez de Aguero M, Ganal-Vonarburg SC, Fuhrer T, et al. The maternal microbiota drives early postnatal innate immune development. Science. 2016;351(6279):1296–1302.
- An D, Oh SF, Olszak T, et al. Sphingolipids from a symbiotic microbe regulate homeostasis of host intestinal natural killer T cells. Cell. 2014;156(1-2):123–133.
- Furusawa Y, Obata Y, Fukuda S, et al. Commensal microbe-derived butyrate induces the differentiation of colonic regulatory T cells. Nature. 2013;504(7480):446–450.
- Smith PM, Howitt MR, Panikov N, et al. The microbial metabolites, short-chain fatty acids, regulate colonic treg cell homeostasis. Science. 2013;341(6145):569–573.
- Thorburn AN, McKenzie CI, Shen S, et al. Evidence that asthma is a developmental origin disease influenced by maternal diet and bacterial metabolites. Nat Commun. 2015;6:7320.
- Al Nabhani Z, Dulauroy S, Marques R, et al. A weaning reaction to microbiota is required for resistance to immunopathologies in the adult. Immunity. 2019;50(5):1276–1288 e5.
- Stensballe LG, Simonsen J, Jensen SM, et al. Use of antibiotics during pregnancy increases the risk of asthma in early childhood. J Pediatr. 2013;162(4):832–838 e3.
- Alhasan MM, Cait AM, Heimesaat MM, et al. Antibiotic use during pregnancy increases offspring asthma severity in a dose-dependent manner. Allergy. 2020;75(8):1979–1990.
- Arrieta MC, Stiemsma LT, Dimitriu PA, CHILD Study Investigators, et al. Early infancy microbial and metabolic alterations affect risk of childhood asthma. Sci Transl Med. 2015;7(307):307ra152.
- Miyoshi J, Bobe AM, Miyoshi S, et al. Peripartum antibiotics promote gut dysbiosis, loss of immune tolerance, and inflammatory bowel disease in genetically prone offspring. Cell Rep. 2017;20(2):491–504.
- Schulfer AF, Battaglia T, Alvarez Y, et al. Intergenerational transfer of antibiotic-perturbed microbiota enhances colitis in susceptible mice. Nat Microbiol. 2018;3(2):234–242.