Abstract
The recognition by cytotoxic T cells (CTLs) is essential for the clearance of SARS-CoV-2 virus-infected cells. Several viral proteins have been described to be recognized by CTLs. Among them, the spike (S) protein is one of the immunogenic proteins. The S protein acts as a ligand for its receptors, and several mutants with different affinities for its cognate receptors have been reported, and certain mutations in the S protein, such as L452R and Y453F, have been found to inhibit the HLA-A24-restricted CTL response. In this study, we conducted a screening of candidate peptides derived from the S protein, specifically targeting those carrying the HLA-A24 binding motif. Among these peptides, we discovered that NF9 (NYNYLYRLF) represents an immunogenic epitope. CTL clones specific to the NF9 peptide were successfully established. These CTL clones exhibited the ability to recognize endogenously expressed NF9 peptide. Interestingly, the CTL clone demonstrated cross-reactivity with the Y453F peptide (NYNYLFRLF) but not with the L452R peptide (NYNYRYRLF). The CTL clone was able to identify the endogenously expressed Y453F mutant peptide. These findings imply that the NF9-specific CTL clone possesses the capability to recognize and respond to the Y453F mutant peptide.
1. Introduction
Severe acute respiratory syndrome coronavirus-2 (SARS-CoV-2) is a strain of coronavirus responsible for causing coronavirus disease-19 (COVID-19), a respiratory illness that has led to a global pandemic [Citation1]. Coronavirus families are also known to cause other severe respiratory diseases, including severe acute respiratory syndrome (SARS) and Middle East respiratory syndrome (MERS) [Citation2]. SARS-CoV-2 has given rise to several variants due to the accumulation of genetic mutations. These variants differ from the original virus in specific genetic sequences, and some of them have raised concerns due to their potential impact on transmissibility, severity, immunogenicity, and vaccine efficacy [Citation3]. SARS-CoV-2 is believed to be a zoonotic virus, likely originating from a coronavirus strain carried by bats [Citation4]. SARS-CoV-2 has the ability to transmit to various animal species, including hamsters, cats, dogs, mink, ferrets, tigers, lions, cynomolgus macaques, rhesus macaques, and treeshrews [Citation5].
The number of COVID-19 cases worldwide has exceeded 770 million, with approximately 7 million deaths and a mortality rate of around 1%. In Japan, however, there have been 33.8 million cases and about 75,000 deaths, resulting in a significantly lower mortality rate of only 0.2% [Citation6]. This variance in mortality rates may stem from factors such as hygiene practices and mask usage. However, we explored the potential influence of a unique Japanese genetic background: the human leukocyte antigen (HLA), a crucial genetic factor that shapes T-cell responses [Citation7]. Among Japanese HLAs, HLA-A24 is notably prevalent [Citation8]. We therefore decided to focus on HLA-A24 and to analyze HLA-A24-restricted immune responses. We investigated the immune response to the SARS-CoV-2 virus spike (S) protein, focusing on the HLA-A*24:02. We also examined its cross-reactivity with the SARS-CoV-2 delta variant (B.1.617.2) [Citation9] and the B.1.1.298 variant, which was associated with an outbreak among farmed minks in Denmark [Citation10].
2. Materials and methods
2.1. Design and synthesis of the peptides
Peptides encoded by the SARS-CoV-2 Spike (S) protein, carrying the Human Leukocyte Antigen (HLA-A24) binding motif, were screened using the NetMHCpan website (https://services.healthtech.dtu.dk/services/NetMHCpan-4.1/) [Citation11]. We used the amino acid sequence of the SARS-CoV-2 S protein (Wuhan-Hu-1, GenBank: MN908947.3). Candidate peptides with HLA-A24 binding potential were synthesized by GenScript (Tokyo, Japan). The peptides were dissolved in DMSO at a concentration of 20 mg/mL and stored at −80 °C.
2.2. Cell line and plasmids
T2-A24 cells were kindly provided by Dr. Kuzushima (Nagoya, Japan) and cultured in RPMI-1640 (SIGMA, St. Louis, MO) supplemented with 10% FBS and G418. K562 cells were purchased from American Type Culture Collection (ATCC) (Rockville, MD) and cultured in RPMI-1640 supplemented with 10% FBS. 293 T cells were also purchased from ATCC and cultured in DMEM supplemented with 10% FBS.
Minigene plasmids were constructed using following oligos; 5′-ggatccgccaccatgaatta taattacctgtatagat tgttttagctcgag-3′ for NF9-mini, 5′-ggatccgccaccatgtc taacaatcttgattctaa ggttggtggtaattataat tacctgtatagattgtttaggaa gtctaatctcaaaccttt tgagagatagctcgag-3′ for NF9-long, 5′-ggatccgccaccatgaa ttataattacctgtttagatt gttttagctcgag-3′ for Y453F-mini, 5′-ggatccgccaccatgtcta acaatcttgattctaag gttggtggtaattataa ttacctgtttagattgttta ggaagtctaatctcaaac cttttgagagatagctcgag-3′ for Y453F-long. Each oligos were cloned into pcDNA3.1(+) plasmid (Thermo Fisher Scientific, Waltham, MA) and confirmed the sequence.
2.3. Isolation of PBMCs and Induction of CTL clone
In this study, we used material derived from healthy donors. The experimental protocol was approved by the institutional-review board of Sapporo Medical University (approval no. 29-2-69), and written informed consent was obtained from the donors. The isolation of PBMCs, CTL induction, and establishment of CTL clones were performed as previously described with some modifications [Citation12]. Peripheral blood mononuclear cells were isolated from HLA-A24-positive donors using Lymphoprep (COSMO BIO Inc., Tokyo, Japan). PBMCs were seeded in 24-well plates (CORNING Inc., Corning, NY) at 2 × 10^6/well in 1 mL of AIM-V medium (Thermo Fisher Scientific) supplemented with 10% HS. The candidate peptides were divided into 3 pools (pool #1, #2, and #3), each containing 5 peptides. Three pools of peptides were added to the PBMCs at a final peptide concentration of 1 μg/mL. IL-2 was added to each well at a final concentration of 100 U/mL. Fresh AIM-V medium supplemented with 10% HS and 100 U/mL IL-2 was added to each well every 3 days.
Bulk CTL cultures were stained with HLA-A24-peptide complex tetramers (MBL Inc.) for 30 min at 4 °C and then analyzed using FACS Aria II. Unstimulated PBMCs were used as a negative control, and the gate for tetramer positivity was defined as lower than 0.1% in the negative control sample. Positively stained cells were single-cell sorted into 96-well round-bottom plates and cultured with 100 Gy-irradiated PBMCs at 5 × 10^4/well in AIM-V supplemented with 10% HS, IL-2 (100 U/mL), IL-15 (10 ng/mL), and PHA (10 μg/mL). Two weeks later, the growing wells were tested by IFNγ ELISPOT assay and tetramer staining.
2.4. IFNγ ELISpot assay
Each cultured well was tested for reactivity by an interferon-γ (IFNγ) ELISPOT assay as described previously [Citation13]. Peptide-pulsed T2-T24 cells were used as positive targets. Peptide-unpulsed T2-T24 cells and K562 cells were used as negative controls.
2.5. Statistical analysis
The data were subjected to statistical analysis using one-way analysis of variance and Tukey’s honestly significant difference test. P-values less than 0.05 were considered indicative of a significant difference.
3. Results
3.1. Identification of immune-Dominant antigenic peptide derived from SARS-CoV-2 S protein
We referred to the amino acid sequence of the SARS-CoV-2 S protein (Wuhan-Hu-1) and screened for peptides carrying the HLA-A24 anchor motif. Fifteen peptides were identified with a positive HLA-A24 binding motif; consequently, these 15 peptides were synthesized (), and the S7 peptide was previously described as the NF9 peptide [Citation14]. We divided the peptides into 3 pools, each containing 5 peptides, respectively (). To assess the immunogenicity of the candidate peptides, we stimulated PBMCs from HLA-A24-positive donors using the 3 peptide pools for 2 weeks (). Subsequently, the reactivity of PBMCs was evaluated using target cells pulsed with each peptide.
Figure 1. Induction of T cell response by HLA-A24 restricted SARS-CoV-2 Spike protein. (A) List of candidate peptides carrying the HLA-A24 binding motif. Fifteen peptides carrying the HLA-A24 binding motif were designed, with each peptide pool containing 5 peptides. (B) PBMC stimulation schema. PBMCs were stimulated using each peptide pool on day 0 and day 7, and the specificity of PBMCs was evaluated using each peptide-pulsed target cells. (C) IFNγ ELISpot assay. PBMCs were evaluated for peptide specificity by an IFNγ ELISpot assay. Fifty thousand PBMCs were co-cultured with each peptide-pulsed target cells, and IFNγ production was assessed. Peptide-unpulsed targets (-) were used as a negative control.
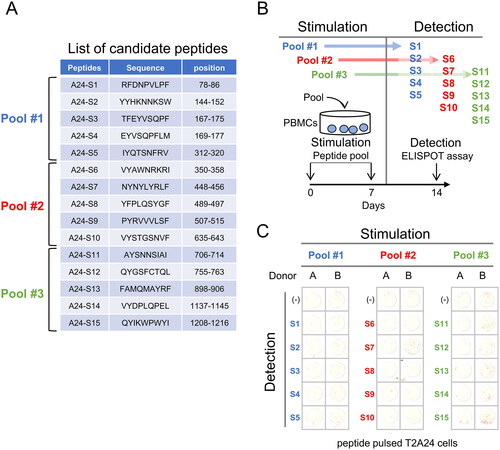
From PBMCs stimulated with peptide pool #2, spots were observed when using S7 peptide-pulsed target cells. Similarly, PBMCs stimulated with peptide pool #3 showed spots with S15 peptide-pulsed target cells (). Therefore, both S7 peptide and S15 peptide are candidates for HLA-A24 binding peptides.
3.2. Establishment of HLA-A24 restricted CTL clone specific for NF9 peptide
To analyze the functions of CTLs, we isolated S7 peptide and S15 peptide-specific cells using HLA-A24-S7 tetramer and HLA-A24-S15 tetramer. The PBMCs specific for S7 (NF9) peptide () and the PBMCs specific for S15 peptide (supplemental Figure) were stained with HLA-A24-S7 tetramer and HLA-A24-S15 tetramer, respectively, and analyzed by flow cytometry. The positive rates for the tetramer were 3.17% in CD8+ cells (). The positive rates for HLA-A24-S15 tetramer were 2.14% (supplemental Figure). Thus, the positivity for S7 peptide was higher than that in S15 peptide.
Figure 2. Establishment of NF9-specific CTL clone. (A) IFNγ ELISpot assay. PBMCs were evaluated for peptide specificity by an IFNγ ELISpot assay using NF9-peptide-pulsed T2-A24 cells as targets. Peptide-unpulsed T2-A24 cells served as a negative control. (B) FACS analysis using NF9 tetramer. PBMCs were stained with the HLA-A24 NF9 complex tetramer and analyzed by flow cytometry. The HLA-A24 HIV peptide complex tetramer was used as a negative control. NF9 tetramer-positive cells were sorted individually. (C) IFNγ ELISpot assay. Tetramer-positive clone cells were evaluated by an IFNγ ELISpot assay using NF9-peptide-pulsed T2-A24 cells as targets. Peptide-unpulsed T2-A24 cells served as a negative control.
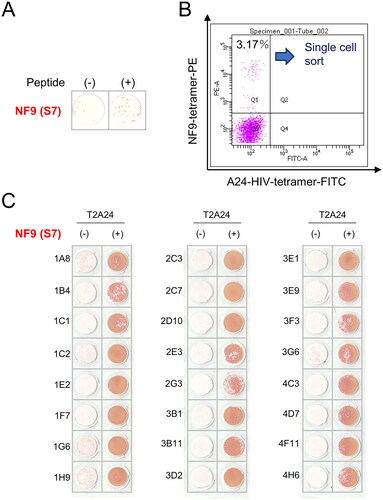
HLA-A24-S7 tetramer-positive cells and HLA-A24-S15 tetramer-positive cells were then single-cell sorted and cultured to establish CTL clones. A total of 16 wells showed growth in HLA-A24-S7 tetramer-sorted cells, and a total of 2 wells showed growth in HLA-A24-S15 tetramer-sorted cells. To evaluate the specificity of the growing wells, an IFNγ ELISPOT assay was performed. All wells in HLA-A24-S7 tetramer-sorted cells showed specific reactivity for S7 (NF9) peptide-pulsed target cells compared with negative control cells (), whereas all wells in HLA-A24-S15 tetramer-sorted cells showed no reactivity to S15 peptide-pulsed target cells (supplemental Figure). Therefore, we further analyzed S7 (NF9) peptide-specific CTL clones.
3.3. NF9-specific CTL clone cross-reacts with Y453F mutant but not L452R mutant
Since the NF9 peptide is encoded in the receptor binding motif (RBM) of the S protein, which is responsible for binding to the cognate receptor ACE2, and some mutants have been reported, we addressed whether our CTL clone can also react to those mutants [Citation14]. The NF9 peptide-specific CTL clone recognized NF9 peptide-pulsed target cells. Interestingly, the CTL clone also recognized the Y453F peptide but not the L452R peptide (). To assess the sensitivity to the Y453F peptide, the CTL reactivity for serially diluted peptide-pulsed targets was examined. The CTL clone recognized the NF9 peptide at 10^−12 M and the Y453F peptide at 10^−10 M (). These data suggest that the NF9 peptide-specific CTL clone can cross-react with the Y453F peptide at a lower affinity.
Figure 3. NF9 peptide-specific CTL clone cross-reacts with the Y453F peptide. (A) IFNγ ELISpot assay. The NF9 peptide-specific CTL clone was evaluated using NF9 peptide, L452R peptide, and Y453F peptide. Each peptide was pulsed onto T2-A24 cells, and the reactivity of the CTL clone was examined by an IFNγ ELISpot assay. Peptide-unpulsed T2-A24 cells were used as a negative control. (B) Peptide titration. Serially diluted NF9 peptide and Y453F peptide were pulsed onto T2-A24 cells and used for an IFNγ ELISpot assay. The NF9 peptide-specific CTL clone was used as effector cells.
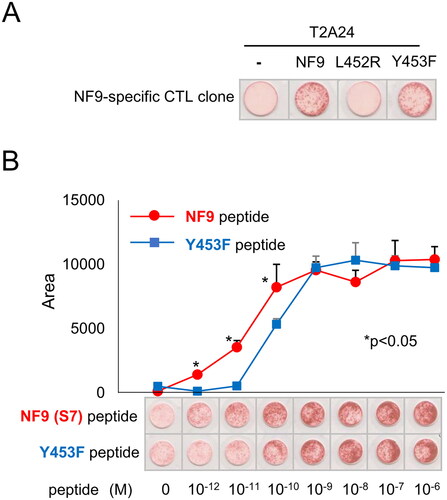
3.4. NF9-specific CTL clone can recognize endogenously expressed Y453F mutant peptide
Since the affinity of the NF9-specific CTL clone for the Y453F mutant peptide was lower than that for the NF9 peptide, we investigated whether the CTL clone could recognize endogenously expressed Y453F peptide. We designed minigenes encoding the minimal epitopes of the NF9 and Y453F mutant peptides (NF9-mini, Y453F-mini) and minigenes with 5′- and 3′-flanking sequences (NF9-long, Y453F-long), as minimal epitope minigenes are not sufficient to evaluate endogenous expression (). The NF9 peptide-specific CTL clone recognized both NF9-mini and NF9-long transfected 293 T cells. Interestingly, the CTL clone recognized Y453F-mini and Y453F-long at lower levels compared to those of NF9-mini and NF9-long (). These findings suggest that the NF9-specific CTL clone can cross-react with endogenously expressed Y453F peptide at lower levels.
Figure 4. NF9 peptide-specific CTL clone recognizes endogenously expressed Y453F peptide. The design of minigenes (upper panel). NF9-mini encodes the minimal epitope, NF9-long encodes the epitope with 5’- and 3’-flanking sequences, Y453F-mini encodes the minimal epitope of the Y453F mutant, and Y453F-long encodes the Y453F epitope with 5’- and 3’-flanking sequences. IFNγ ELISpot assay (lower panel). Minigenes were co-transfected with HLA-a*24:02 cDNA into 293 T cells and used for an IFNγ ELISpot assay. The NF9 peptide-specific CTL clone was used as an effector. Minigene-negative 293 T cells were used as a negative control. NF9 peptide and Y453F peptide-pulsed T2-A24 cells were used as positive controls.
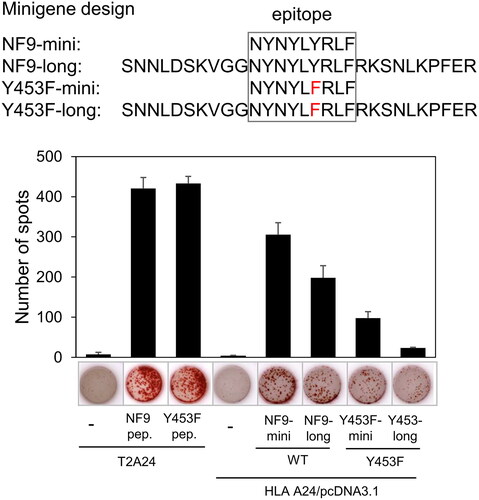
4. Discussion
In this study, we conducted a screening of HLA-A24 binding peptides derived from the S protein and identified the NF9 peptide as the most immunogenic among them. A prior study established the S protein as the primary target for the immune response among the proteins encoded by the SARS-CoV-2 gene [Citation15]. Therefore, CTL response to NF9 peptide might be important for HLA-A24-positive individuals. We successfully generated a novel CTL clone specific to the NF9 peptide. Remarkably, this CTL clone exhibited a remarkable affinity for the NF9 peptide, recognizing it even at a concentration as low as 10^-12 M. This high affinity suggests the potential effectiveness of T cell receptors (TCRs) for further therapeutic approaches, such as TCR-transduced T cell (TCR-T) therapy, targeting SARS-CoV-2.
The Y453F mutant is encoded by a SARS-CoV-2 variant, B.1.1.298, linked to an outbreak among farmed minks in Denmark [Citation10]. The S protein carrying the Y453F mutant showed a strong binding affinity to the mink ACE2 protein [Citation16]. Interestingly, the Y453F mutant did not enhance virus entry into human cells but did reduce neutralization by antibodies [Citation17]. In terms of the CTL response, Motozono et al. reported that NF9-specific CTLs did not cross-react with the Y453F peptide [Citation14]. However, in our study, the CTL clone specific to the NF9 peptide recognized the endogenously expressed Y453F mutant peptide. This discrepancy may be attributed to differences in assay sensitivity, as we utilized CTL clones while Motozono et al. employed bulk lymphocytes.
The mechanisms by which the NF9-specific TCR cross-reacts with the Y453F peptide but not with L452R remain elusive. However, we hypothesize that one possible explanation for this phenomenon is the similarity in amino acid sequence. The distinction between tyrosine (Y) and phenylalanine (F) is only one hydroxy group, whereas leucine (L) is a hydrophobic amino acid, and lysine (R) is a basic amino acid with different charge characteristics. These differences may be relevant to the cross-reactivity of the TCR.
It is essential to note that the SARS-CoV-2 virus can infect not only minks but also bats and various other animal species, potentially leading to the emergence of variants carrying mutations within the receptor-binding motif (RBM) in the future. As a result, the findings of this study hold significant importance.
Intriguingly, the CTL clone used in this study did not exhibit cross-reactivity with the L452R mutant peptide. Despite our efforts to generate CTLs specific to the L452R peptide by stimulating peripheral blood mononuclear cells (PBMCs) with the L452R peptide, we could not detect an L452R response (data not shown). Consequently, the L452R mutant, encoded by the B.1.617.2 variant (also known as the delta variant) [Citation9], may not represent an immunogenic epitope concerning HLA-A24.
In summary, our study focused on screening HLA-A24 binding peptides encoded by the SARS-CoV-2 virus S protein, identifying the NF9 peptide as an immune-dominant epitope. We successfully established a novel CTL clone specific to the NF9 peptide, which exhibited cross-reactivity with the Y453F mutant peptide. These findings suggest that NF9 peptide-specific CTLs may play a role in clearing the Y453F mutant virus.
Suppl_Figure .tif
Download TIFF Image (482.5 KB)Disclosure statement
No potential conflict of interest was reported by the author(s).
Additional information
Funding
References
- Zhou P, Yang X-L, Wang X-G, et al. A pneumonia outbreak associated with a new coronavirus of probable bat origin. Nature. 2020;579(7798):270–273. doi:10.1038/s41586-020-2012-7.
- Mann R, Perisetti A, Gajendran M, et al. Clinical characteristics, diagnosis, and treatment of major coronavirus outbreaks. Front Med (Lausanne). 2020;7:581521. doi:10.3389/fmed.2020.581521.
- Carabelli AM, Peacock TP, Thorne LG, et al. SARS-CoV-2 variant biology: immune escape, transmission and fitness. Nat Rev Microbiol. 2023;21(3):162–177. doi:10.1038/s41579-022-00841-7.
- Temmam S, Vongphayloth K, Baquero E, et al. Bat coronaviruses related to SARS-CoV-2 and infectious for human cells. Nature. 2022;604(7905):330–336. doi:10.1038/s41586-022-04532-4.
- Nielsen SS, Alvarez J, Bicout DJ, et al. SARS-CoV-2 in animals: susceptibility of animal species, risk for animal and public health, monitoring, prevention and control. EFSA J. 2023;21:e07822.
- World Health Organization. (who.int). 2022.
- Murphy KM, Weaver C, Berg LJ. Immunobiology. 2022; 10th edition.
- Nakaoka H, Inoue I. Distribution of HLA haplotypes across Japanese Archipelago: similarity, difference and admixture. J Hum Genet. 2015;60(11):683–690. doi:10.1038/jhg.2015.90.
- Mlcochova P, Kemp SA, Dhar MS, et al. SARS-CoV-2 B.1.617.2 Delta variant replication and immune evasion. Nature. 2021;599(7883):114–119. doi:10.1038/s41586-021-03944-y.
- Oude Munnink BB, Sikkema RS, Nieuwenhuijse DF, et al. Transmission of SARS-CoV-2 on mink farms between humans and mink and back to humans. Science. 2021;371(6525):172–177. doi:10.1126/science.abe5901.
- Reynisson B, Alvarez B, Paul S, et al. NetMHCpan-4.1 and NetMHCIIpan-4.0: improved predictions of MHC antigen presentation by concurrent motif deconvolution and integration of MS MHC eluted ligand data. Nucleic Acids Res. 2020;48(W1):W449–W454. doi:10.1093/nar/gkaa379.
- Morita R, Hirohashi Y, Nakatsugawa M, et al. Production of multiple CTL epitopes from multiple tumor-associated antigens. Methods Mol Biol. 2014;1139:345–355. doi:10.1007/978-1-4939-0345-0_28.
- Yamada S, Miyata H, Isono M, et al. Cisplatin resistance driver claspin is a target for immunotherapy in urothelial carcinoma. Cancer Immunol Immunother. 2023;72(7):2057–2065. doi:10.1007/s00262-023-03388-5.
- Motozono C, Toyoda M, Zahradnik J, et al. SARS-CoV-2 spike L452R variant evades cellular immunity and increases infectivity. Cell Host Microbe. 2021;29(7):1124–1136.e11. doi:10.1016/j.chom.2021.06.006.
- Grifoni A, Weiskopf D, Ramirez SI, et al. Targets of T cell responses to SARS-CoV-2 coronavirus in humans with COVID-19 disease and unexposed individuals. Cell. 2020;181(7):1489–1501.e15. doi:10.1016/j.cell.2020.05.015.
- Ren W, Lan J, Ju X, et al. Mutation Y453F in the spike protein of SARS-CoV-2 enhances interaction with the mink ACE2 receptor for host adaption. PLoS Pathog. 2021;17(11):e1010053. doi:10.1371/journal.ppat.1010053.
- Hoffmann M, Zhang L, Krüger N, et al. SARS-CoV-2 mutations acquired in mink reduce antibody-mediated neutralization. Cell Rep. 2021;35(3):109017. doi:10.1016/j.celrep.2021.109017.