Abstract
Type I interferons (IFN-Is) play a significant role in systemic lupus erythematosus (SLE) pathogenesis. Double-filtration plasmapheresis (DFPP) is a treatment option for SLE; however, its effect on IFN-Is remains unclear. Therefore, we investigated the effects of DFPP on IFN-Is. Plasma from patients with SLE (n = 11) who regularly underwent DFPP was analysed using a cell-based reporter system to detect the bioavailability and inducing activity of IFN-I. The concentration of plasma dsDNA was measured, and western blotting analysis was used to assess the phosphorylation of the STING pathway. A higher IFN-I bioavailability and inducing activity were observed in patients compared to healthy controls, and both parameters decreased after DFPP. The reduction in IFN-I-inducing activity was particularly prominent in patients with high disease activity. Notably, this reduction was not observed in STING-knockout reporter cells. Additionally, plasma dsDNA levels decreased after DFPP treatment, suggesting that inhibition of the STING pathway was responsible for the observed decrease in activity. Western blotting analysis revealed suppression of STING pathway phosphorylation after DFPP. DFPP reduced IFN-I bioavailability and the inducing activity of plasma. This reduction is likely attributable to the inhibition of the STING pathway through the elimination of dsDNA.
1. Introduction
Systemic lupus erythematosus (SLE) is an autoimmune disease characterised by loss of immune tolerance and persistent production of autoantibodies [Citation1]. While various immune cells and cytokines are involved in the pathogenesis of SLE, type I interferon (IFN-I) is recognised for its vital role in SLE pathogenesis [Citation2–7]. Elevated levels of IFN-I in the blood of patients with SLE have been linked to disease activity, whereas the expression of IFN-I-stimulated genes (ISG) in peripheral blood mononuclear cells (PBMCs) is increased in SLE [Citation8–13]. In both animal models and humans, IFN-I overexpression or administration exacerbates the pathogenesis of SLE [Citation14–19]. Clinical trials have shown that therapies targeting IFN-I are more effective in patients with elevated IFN-I-stimulated gene expression [Citation20,Citation21].
The activation of pattern recognition receptors (PRRs) in innate immune cells is crucial for IFN-I production. The toll-like receptor (TLR) and cyclic GMP-AMP synthase (cGAS)–stimulator of IFN genes (STING) pathways are the primary nucleic acid recognition pathways [Citation22,Citation23]. TLR7/TLR9 recognises nucleic acids in endosomes and activates IFN-I production through MyD88 and interferon regulatory factor (IRF) 5 [Citation24]. Furthermore, upon binding to deoxyribonucleic acid (DNA), cGAS synthesises 2′-3′-cyclic GMP-AMP, which binds to STING, leading to TANK-binding kinase 1 and IRF3 activation through a phosphorylation-dependent mechanism [Citation25–27]. IFN-I production in patients with SLE is thought to be mainly induced by plasmacytoid dendritic cells (pDCs) via the TLR7/TLR9 signalling pathway [Citation28–32]. We previously reported that IFN-I production is enhanced in pDCs from patients with lupus and that the degree of activation correlates with disease activity and serum IFN-I levels [Citation33]. Similarly, we reported that IFN-I production via the STING pathway is increased in patients with SLE, particularly in monocytes, and that the frequency of IFN-I-producing monocytes is correlated with disease activity [Citation34]. In mouse models, cGAS or STING knockout reduced the development of lupus-like symptoms [Citation35–38].
Double-filtration plasmapheresis (DFPP) is a two-step filtration method that involves passing the plasma separated by a plasma separator through a plasma component separator [Citation39]. The plasma component separator concentrates and removes fractions containing pathogenic agents, such as immunoglobulins and immune complexes before returning the plasma enriched with albumin to the patient’s body [Citation40,Citation41]. The effectiveness of plasmapheresis in patients with SLE was initially reported in 1976 [Citation42], and a combination of cyclophosphamide and plasmapheresis was later found to be more effective and safer than monotherapy in inducing remission in patients with lupus nephritis [Citation43,Citation44]. Data on the efficacy of apheresis in treating glomerulonephritis and alveolar haemorrhage due to SLE are gradually accumulating [Citation44–47]. In Japan, patients with SLE are allowed to undergo DFPP up to four times a month, but we have been using DFPP at two-week or four-week intervals as maintenance therapy. We collected 5 years of clinical data from seven patients whose data had been registered in the electronic medical record system since the start of DFPP, and in all cases, data from laboratory tests showed a trend towards improvement and a reduction in glucocorticoid dose (Supplementary Figure 1). Notably, there were no major flare-ups. Therefore, DFPP may play a role in reducing disease activity and preventing relapse, despite the lack of clearly established evidence for maintenance therapy for SLE.
The therapeutic mechanism of DFPP is thought to involve the removal of circulating immune complexes, as it is more effective in patients with high levels of circulating immune complexes. Plasmapheresis reduces circulating immune complexes and anti-dsDNA antibody levels in the blood [Citation48,Citation49]. However, the detailed underlying mechanisms have yet to be elucidated.
Therefore, we conducted this study to investigate the effect of DFPP on the IFN-I bioavailability and inducing activity in the plasma of patients with SLE as part of our efforts to understand the therapeutic mechanism of DFPP.
2. Materials and methods
2.1. Clinical samples
The study included Japanese patients with SLE (n = 11) who regularly underwent DFPP at Juntendo University Hospital in May 2022. SLE was diagnosed based on the 1997 revised criteria for the classification of SLE by the American College of Rheumatology. Patients who underwent regular DFPP as maintenance therapy rather than for remission induction were included. Cases where DFPP was performed three times or less, or where blood products other than DFPP were administered within two weeks, were excluded. This exclusion was necessary because discussing the effectiveness of DFPP as a maintenance therapy under these conditions would be challenging.
Healthy control (HC) samples were obtained from five volunteer donors with no history of autoimmune diseases who had never received immunosuppressive therapy. This study complied with the Declaration of Helsinki, and the research protocol was approved by the ethics committee at Juntendo University. Written informed consent was obtained from all subjects before they were enrolled in the study. presents information on age, sex, symptoms, laboratory data, and patient treatment, whereas Supplementary Table 1 provides detailed patient profiles. Peripheral blood was collected from both patients and HCs; the blood from patients was collected before and at the end of treatment. Fresh plasma was obtained by centrifuging blood collected in sodium citrate-containing blood collection tubes at 3000 × g for 15 min and subsequently stored at −20 °C.
Table 1. Profiles of healthy control subjects and patients with SLE.
2.2. Plasmapheresis protocol
Two types of filters with different pore sizes were utilised for the DFPP: a plasma separator (Plasmaflo® OP-05D/08D, Asahi Kasei Medical Company Limited), which separates plasma from blood, and a plasma component separator (Cascadeflo® EC-20W, Asahi Kasei Medical Company Limited), which fractionates plasma based on molecular weight [Citation39]. We treated 40 mL/kg of plasma per session. High-molecular-weight components, such as autoantibodies and other pathogenic substances, were discarded, whereas low-molecular-weight components like albumin were returned to the patient. The plasma component separator had a low sieve factor, discarding 20% of the processed plasma volume; therefore, an equivalent volume of 12% albumin solution was used as replacement fluid. Nafamostat mesylate served as the anticoagulant.
2.3. Reporter cell lines and cell-based reporter assays
The cell reporter assay method was based on established methods [Citation50]. IFN-α/β reporter HEK 293 cells, THP1-Dual, and THP1-Dual-KO-STING were purchased from InvivoGen (San Diego, USA). To quantify IFN-I bioavailability, IFN-α/β reporter HEK 293 cells were incubated with 10% plasma for 24 h. Secreted embryonic alkaline phosphatase (SEAP) was quantified in the cell culture supernatant using QUANTI-Blue (InvivoGen) by measuring the optical density at 620 nm.
Before quantifying IFN-inducing activity, THP1-Dual and THP1-Dual-KO-STING cells were differentiated into macrophages by culturing in RPMI-1640 medium supplemented with 10% foetal calf serum (FCS) and 10 ng/mL phorbol 12-myristate 13-acetate (PMA) daily, and then rested in RPMI-1640 with 10% FCS. To quantify the IFN-inducing activity, the cells were incubated with 5% plasma for 18 h. Lucia luciferase in the supernatant was quantified using a QUANTI-Luc 4 Lucia/Gaussia (InvivoGen) by measuring the relative light units of chemiluminescence. To inhibit IFN-I, an IFN-I blocking cocktail was used in combination with 1 μg/mL anti-human IFN-α2 (InvivoGen), 1 μg/mL anti-human IFN-β (InvivoGen), and 1 μg/mL anti-IFN-α/β receptor chain 2 antibodies (Merck, Germany).
2.4. Measurement of deoxyribonucleic acid
Plasma dsDNA concentration was measured using the Quant-iT PicoGreen dsDNA assay kit (Invitrogen, Thermo Fisher Scientific, USA). Plasma single-stranded DNA (ssDNA) concentration was measured using the Quant-iT OliGreen ssDNA Assay Kit (Invitrogen, Thermo Fisher Scientific, USA), following the manufacturer’s instructions.
2.5. Western blotting analysis
Macrophages were lysed in a radioimmunoprecipitation assay buffer (Nacalai Tesque, Kyoto, Japan). The protein concentration was quantified using a bicinchoninic acid protein assay kit (Nacalai Tesque), following the manufacturer’s instructions. Subsequently, 10 µg of total protein per sample was loaded on 4–20% Mini-PROTEAN TGX precast gels from Bio-Rad (Hercules, CA, USA). The lysates were subjected to SDS-PAGE, and western blotting was conducted using anti-STING mouse mAb (Proteintech, IL, USA), anti-IRF3 mouse mAb (Proteintech), anti-phospho-STING rabbit mAb (Cell Signalling Technology, Danvers, MA, USA), anti-Phospho-IRF3 mouse mAb (Cell Signalling Technology), and anti-GAPDH mouse mAb (Proteintech). Blots were visualised using an enhanced chemiluminescence assay with Super Signal West Dura Extended Duration Substrate (Thermo Fisher Scientific), following the manufacturer’s instructions. Signals were detected using a LAS 4000 biomolecular imager (GE Healthcare, IL, USA) and quantified using ImageQuant TL.
2.6. Statistical analysis
Data were analysed using Prism 10 (GraphPad, Boston, MA, USA), and differences between groups were evaluated using the Wilcoxon signed rank test as well as the paired t-test. For comparisons between two independent groups, the Mann–Whitney U test was used. Associations between two variables, including clinical data, were assessed using Spearman’s correlation. Associations between two variables obtained from the light intensity measurements were assessed using Pearson’s correlation after confirming that the distribution was parametric via the Kolmogorov–Smirnov test. The significance level was set at p < .05. In cases where a significant difference was not expected due to the limited number of samples, the condition ‘tendency observed’ was defined for p-values <.1.
3. Results
3.1. Plasma from patients with SLE had high IFN-I bioavailability and IFN-I-inducing activity, which decreased after DFPP treatment
Initially, we assessed IFN-I bioavailability in the plasma of patients with SLE. To achieve this, IFN-α/β reporter HEK293 cells, a human foetal kidney cell carrying a reporter gene that releases SEAP in response to the transcription of an IFN-stimulated gene. The results indicated that IFN-I bioavailability in the plasma of patients with SLE was significantly higher than that in the plasma of HCs (). Moreover, DFPP reduced IFN-I bioavailability in the plasma.
Figure 1. DFPP treatment decreases IFN-I-inducing activity and bioavailability. (A) Type I interferon (IFN-I) bioavailability in plasma. IFN-I bioavailability in patients with SLE (n = 11) and healthy control (HC) subjects (n = 5) was quantified using IFN-α/β reporter HEK 293 cells and measuring the optical density (OD) at 620 nm. (B) IFN-I-inducing activity in plasma activity measured in relative light units (RLU). (C) Correlation between IFN-I-inducing activity in the plasma of patients with SLE and SLE disease activity before double filtration plasmapheresis (DFPP) treatment, assessed according to SLEDAI-2K. (D) Correlation between the IFN-I-inducing activity reduction ratio and SLE disease activity. The ratio of IFN-I-inducing activity reduction was calculated by dividing the value after DFPP by the value before DFPP treatment. (E) Correlation between IFN-I-inducing activity and bioavailability in the plasma of SLE patients before undergoing DFPP treatment. Each symbol represents an individual. Differences between the groups were evaluated using the Wilcoxon signed-rank test (A,B). Two asterisks (**) indicate p < .01. The associations between two variables were assessed using Spearman’s correlation (C,D) and Pearson’s correlation (E).
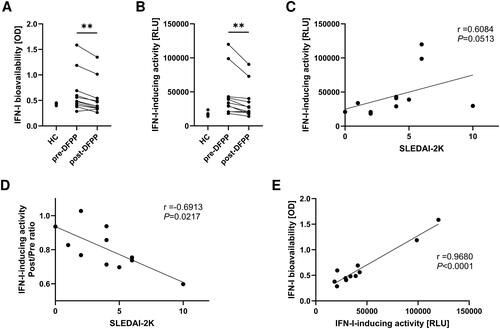
Next, we aimed to assess the IFN-I-inducing activity in plasma from patients with SLE. To improve the sensitivity of the measurement, THP-1 Dual cells, human monocytes carrying a reporter gene that releases luciferase in response to IFN-I production, were differentiated into human macrophages by using PMA before the experiment. The cells were then incubated for 18 h in a culture medium supplemented with 5% patient plasma, and IFN-I-inducing activity was assessed by measuring luminescence levels. The results demonstrated that the IFN-I-inducing activity in the plasma of patients with SLE was significantly higher than that in HCs (). Furthermore, DFPP treatment reduced the IFN-I-inducing activity, and this reduction was proportional to the SLEDAI-2K score, a measure of disease activity in SLE (). Notably, a higher level of disease activity was associated with a greater pre–post ratio of the therapeutic effects of DFPP (). We also observed a strong correlation between IFN-I bioavailability and IFN-I-inducing activity in the plasma of patients with SLE ().
3.2. IFN-I-inducing activity was reduced using the IFN-I blocking cocktail but still reduced by DFPP treatment
IFN-I induces IFN-I production through the JAK–STAT pathway. Therefore, it is imperative to investigate whether IFN-I in the plasma amplifies the production of IFN-I or whether other factors induce IFN-I. During the stimulation of human macrophages with plasma from patients with SLE to measure the IFN-I-inducing activity, we added a blocking cocktail targeting IFN-I and its receptor (see Materials and Methods for details). Although the addition of the blocking cocktail led to a reduction in IFN-I-inducing activity (), a significant difference was still observed before and after DFPP in the presence of IFN-I blocking (). This suggests the presence of a mechanism beyond IFN-I induction by itself.
Figure 2. IFN-I-inducing activity was reduced by the IFN-I blocking cocktail and further reduced by DFPP treatment. (A) Type I interferon (IFN-I)-inducing activity in plasma with or without an IFN blocking cocktail. IFN-I-inducing activity in patients with SLE (n = 11) and healthy control (HC) subjects (n = 5) was quantified by using THP-1 dual macrophages incubated with or without an IFN-I blocking cocktail and measuring relative light units (RLU) of chemiluminescence. Plasma samples from SLE patients were collected pre- and post-double-filtration plasmapheresis (DFPP) treatment. The IFN-I blocking cocktail contained 1 μg/mL each of anti-human IFN-α2, anti-human IFN-β antibodies, and anti-IFN-α/β receptor chain 2 antibodies. (B) IFN-I-inducing activity in plasma with an IFN-I blocking cocktail. Each symbol represents an individual. Differences between the groups were evaluated using the Wilcoxon signed-rank test (A,B). Two asterisks (**) indicate p < .01, and three asterisks (***) denote p < .001.
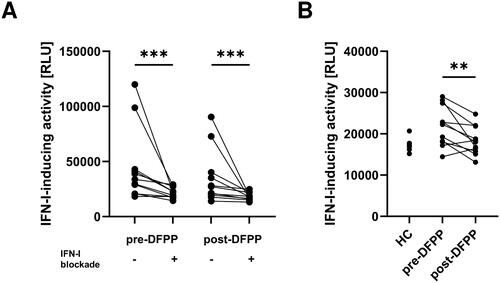
3.3. Reduction of IFN-I-inducing activity was not observed in STING-knockout reporter cells, and plasma dsDNA decreased following DFPP
In a previous study, we elucidated the involvement of the STING pathway in IFN-I production in patients with SLE. We hypothesised that this pathway may underlie the reduction in IFN-I-inducing activity following DFPP. Consequently, we assessed the IFN-I-inducing activity in human macrophages with STING knockout. The results revealed no discernible differences in IFN-I-inducing activity before and after DFPP treatment (). dsDNA activates the STING pathway by binding to cGAS. Subsequently, we analysed the plasma dsDNA concentration and found that it declined following DFPP treatment (). Given the apparent correlation between the dsDNA removal rate and the reduction of IFN-I-inducing activity in plasma (), we postulated that the STING pathway significantly contributes to the reduction of IFN-I-inducing activity due to DFPP therapy.
Figure 3. Reduction of IFN-I-inducing activity was not observed in STING-knockout reporter cells. (A) Type I interferon (IFN-I)-inducing activity in plasma of patients with SLE (n = 11) and HCs (n = 5), quantified using THP1-Dual-KO-STING cells differentiated into macrophages and measuring relative light units (RLU) of chemiluminescence. Plasma samples from SLE patients were collected pre- and post-double-filtration plasmapheresis (DFPP) treatment. (B) Concentration of plasma double-stranded DNA (dsDNA). (C) Correlation between the reduction in IFN-I-inducing activity and plasma dsDNA removal rate. Each symbol represents an individual. The overall differences between the groups were evaluated using the Wilcoxon signed-rank test (A,B). Three asterisks (***) indicate p < .001. Associations between the two variables were assessed using Pearson’s correlation coefficient (C).
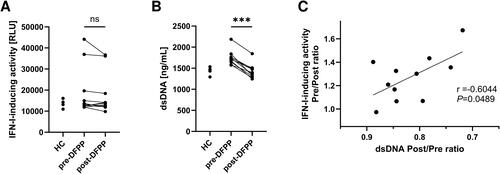
3.4. Western blotting analysis revealed reduced STING and IRF3 phosphorylation following DFPP
To investigate the role of the STING pathway in the reduction of IFN-I-inducing activity by DFPP treatment, we analysed STING phosphorylation in stimulated human macrophages using western blotting. These results revealed a reduction in STING phosphorylation following DFPP treatment, indicating that DFPP reduced STING pathway activation (). Similarly, IRF3 phosphorylation was reduced (), consistent with a reduced activation of the STING pathway.
Figure 4. DFPP treatment reduced STING and IRF3 phosphorylation. Extracts were prepared from THP-1 macrophages stimulated with either RPMI1640 alone (N), 1 μg/mL of 2′-3′-cyclic GMP-AMP (P), or plasma from patients with SLE (1–11) and subjected to western blotting analysis. Plasma samples from SLE patients were pre- and post-double-filtration plasmapheresis (DFPP) treatment. (A,B) Fold-change in the protein levels of stimulators of interferon genes (STING) and phosphorylated STING (pSTING) levels (A) and interferon regulatory factor (IRF3) and phosphorylated IRF3 (pIRF3) levels (B). Glyceraldehyde-3-phosphate dehydrogenase (GAPDH) was used as a loading control for protein normalisation. (C,D) pSTING (C) and pIRF3 (D) band intensity comparisons were conducted with normalisation against STING and IRF band intensities, respectively. Each symbol represents an individual. The overall differences between the groups were evaluated using the Wilcoxon signed-rank test (C,D). Two asterisks (**) indicate p < .01.
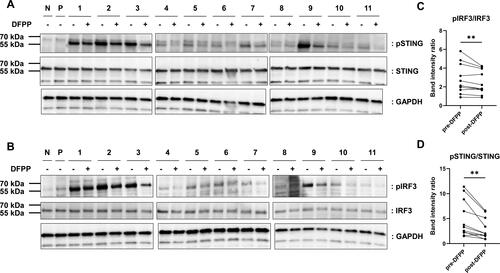
4. Discussion
We report that DFPP treatment effectively reduces IFN-I bioavailability and induces activity in the plasma of patients with SLE. We propose a possible mechanism involving the inhibition of the STING pathway via the removal of plasma dsDNA by DFPP.
Previous investigations have shown that blood IFN-I and plasma DNA levels correlate with disease activity in patients with SLE [Citation9,Citation51]. Extracellular dsDNA, when taken up by the immune cells, stimulates IFN-I production through the TLR9 and STING pathways [Citation22,Citation23]. Previous reports have indicated that an upregulation of cGAS expression in PBMCs of SLE patients, particularly in monocyte lineage cells, leads to enhanced IFNα production via the STING pathway and that the frequency of cGAS expression correlates with disease activity [Citation34]. A recent study demonstrated that dsDNA in the serum of patients with SLE promotes ISG expression via the cGAS–STING pathway, using a cell-based reporter system [Citation50]. This study further demonstrated that levels of IFN-I bioavailability and inducing activity are associated with disease activity. Similarly, our study showed elevated IFN-I bioavailability and induing activity in plasma from patients with SLE compared to that from HCs, and IFN-inducing activity tended to correlate with disease activity. The removal of extracellular dsDNA from the blood is expected to reduce IFN-I production through the STING pathway. In this study, we demonstrated that DFPP effectively removed plasma dsDNA, suppressed the activation of the STING pathway, and reduced IFN-I-inducing activity in the plasma. Based on previous studies, we proposed a causal relationship between these findings.
It is possible that extracellular dsDNA in plasma binds to anti-DNA antibodies and forms circulating immune complexes in the blood of patients with SLE. This is supported by previous research indicating a correlation between disease activity and anti-DNA antibody levels, as well as a link between anti-DNA antibody levels and IFN-I levels in the plasma [Citation9]. Previous in vitro studies have shown that immune complexes are taken up by monocytes via FcγRIIa and induce IFNα production [Citation52]. Additionally, anti-ds-DNA antibodies accelerate DNA-stimulated IFNα production by PBMCs [Citation53]. The therapeutic efficacy of apheresis in patients with SLE is believed to depend on the removal of autoantibodies and circulating immune complexes [Citation48,Citation49]. Considering that the widely accepted therapeutic efficacy of apheresis in patients with SLE is based on the removal of autoantibodies and circulating immune complexes, it is reasonable to assume that the dsDNA removed in this study may have formed immune complexes. Recent reports suggest that immune complexes induce the release of neutrophil extracellular traps, which can stimulate IFN-I production [Citation54]. This implies that the therapeutic effects of DFPP may extend beyond the proposed STING pathway.
As previously noted, in SLE, IFN-I is primarily produced through both the STING and TLR pathways. TLR9 recognises DNA but, unlike the STING pathway, activates downstream signals through unmethylated ssDNA with contiguous cytosine and guanine sequences. The THP-1 cell line used in this study exhibits low TLR9 expression; thus, this pathway has not been fully investigated. The concentration of ssDNA in plasma decreases with DFPP treatment, despite no observed increase in ssDNA concentration in the plasma of patients compared to HCs (Supplementary Figure 2). Additionally, the binding of autoantibodies to nucleic acid components is known to delay clearance by deoxyribonuclease [Citation55,Citation56]. The removal of autoantibodies by DFPP may indirectly contribute to the suppression of IFN-I production. Moreover, TLR7/8 recognises RNA and promotes IFN-I production, contributing to the pathogenesis of SLE [Citation33,Citation57]. TLR7, which recognises single-stranded RNA, is highly expressed in plasmacytoid dendritic cells and serves as a major source of IFN-I in SLE [Citation51]. The potential for controlling SLE disease activity through TLR7/8 inhibition is being explored, with clinical trials for new drugs underway. Although the dynamics of RNA in plasma were not measured in this study, it is possible that similar to DNA, RNA bound to autoantibodies is removed by DFPP. The potential for controlling SLE disease activity through TLR7/8 inhibition is being explored, and clinical trials with new drugs are in progress [Citation58].
In the present study, we observed a reduction in the bioavailability of IFN-I after DFPP treatment. The correlation between the reduction in IFN-I activity and IFN-I-inducing activity implied a strong connection between the two. This reduction may also stem from the direct removal of IFN-I and not only the reduction in IFN-I induction. We used a plasma component separator (Cascadeflo® EC-20W, Asahi Kasei Medical Company Limited) with a pore size of 0.03 μm that blocks 65% of albumin from passing through. Considering that albumin has a molecular weight of 66,500 Da, a certain percentage of IFN-I, which averages 20 kDa, may pass through the plasma component separator and should return to the patient. However, IFN-I bioavailability in patient plasma actually decreased after DFPP. This reduction may stem from the direct removal of IFN-I, as manufacturers report a blockage rate of just over 30% for small molecules like 20 kDa IFN-I.
In SLE, an increase in various cytokines within the blood has been documented, playing a crucial role in pathogenesis by mediating complex interactions between immune cells and target tissues [Citation59,Citation60]. Monocytes, macrophages, and dendritic cells produce inflammatory cytokines, such as tumour necrosis factor-alpha (TNF-α) and interleukin-6 (IL-6), not only IFN-I. It is known that the activation of TLR leads to the activation of nuclear factor-kappa B and mitogen-activated protein kinase pathways, which promote the gene transcription of these cytokines [Citation24]. Consequently, the removal of dsDNA from the blood may indirectly inhibit the production of TNF-α and IL-6 by suppressing TLR9 activation. Additionally, these cells produce B cell-activating factor (BAFF), which promotes the maturation and long-term survival of autoreactive B cells and plasma cells, contributing to the exacerbation and relapse of SLE [Citation61]. The inhibition of BAFF has been shown to improve disease activity, as evidenced by the efficacy of belimumab [Citation62,Citation63]. If DFPP directly removes IFN-I, it is also likely that IL-6 and BAFF, which are involved in the activation of the acquired immune system, are removed, suggesting an unexplored potential of DFPP.
One of the major findings of this study is the observation that the reduction in IFN-I-inducing activity was particularly significant in patients with higher levels of disease activity. As disease activity increased, IFN-I-inducing activity also tended to be higher. Cases with high IFN-I production are expected to have elevated disease activity [Citation64]. Consequently, it is suggested that DFPP, which removes nucleic acid substances and suppresses IFN-I production, may offer more significant benefits. This implies that DFPP could be particularly effective in the acute phase of treatment, where IFN-I substantially contributes to disease pathogenesis.
The relationship between concomitant medications and IFN-I dynamics in patients participating in this study was examined (Supplementary Figure 3). Hydroxychloroquine (HCQ) is known to inhibit IFN-I production by blocking TLR signalling [Citation65,Citation66]. Patients were divided into two groups: those receiving HCQ and those not. Disease activity, IFN-I bioavailability, and inducing activity, and dsDNA levels were compared. Although the small sample size precluded significant differences, a trend of lower IFN-I bioavailability was noted in the HCQ group compared to the non-HCQ group, despite similar dsDNA levels. Additionally, the correlation between glucocorticoid dosage and disease activity, IFN-I bioavailability and inducing activity, and dsDNA levels was also examined. A significant correlation was observed between glucocorticoid dosage and IFN-I bioavailability. It is reported that low-dose glucocorticoids do not affect IFN-I stimulation gene expression [Citation67–69]. Previous studies indicate that IFN-I is correlated with disease activity and symptoms, such as fatigue, fever, and arthritis [Citation64,Citation70,Citation71]. Furthermore, it has been reported that anifrolumab improves pain, fatigue, and mood [Citation72]. Elevated blood IFN-I levels may manifest symptoms, such as fatigue and joint pain, potentially impeding the reduction of glucocorticoid dosage. From this perspective, DFPP may have been effective in managing symptoms that conventional glucocorticoids and immunosuppressants could not control. Although anifrolumab [Citation73] may replace this role, it is noteworthy that DFPP does not cause excessive immunosuppression through IFN-I signature inhibition, making it safe with a low risk of infection, particularly herpes zoster. Therefore, during the glucocorticoid tapering process, if symptoms, such as fatigue and joint pain reemerge and disease activity increases, and if adding immunosuppressants, such as anifrolumab is challenging due to recurring viral infection [Citation74], DFPP remains an important therapeutic option in maintenance therapy.
To the best of our knowledge, this study represents the first report of DFPP reducing IFN-I bioavailability and IFN-I-inducing activity in the plasma of patients with SLE, shedding light on one aspect of the therapeutic mechanism. A recent report indicates that a subset of patients with SLE harbour neutralising anti-IFN-Abs that can modulate B cell responses and are associated with a better disease outcome [Citation75]. In recent years, anti-IFN-I blockade therapy has become a treatment option for SLE [Citation76]; therefore, the usefulness of reducing IFN-I in maintenance therapy is supported, and the effect of DFPP in reducing IFN-I-inducing activity is worth noting.
This study focuses on the efficacy of DFPP as a maintenance therapy. The role of IFN-I in the pathogenesis of SLE is significant, suggesting that DFPP may also play a major role in remission induction therapy for early onset and relapse. Therefore, our future work will encompass reassessing the effectiveness of DFPP in combination with current standard therapy in refractory and severe diseases.
A limitation of this study is that the evaluation of IFN-inducing activity was confined to THP-1 cell-derived macrophages. Due to the low TLR9 expression in these cells, we could not evaluate TLR9-mediated IFN-I production. Although our western blotting results clearly indicate the contribution of the STING pathway to the reduction in IFN-I-inducing activity, we must acknowledge that alternative pathways may play a role in this reduction. For instance, it is possible that certain other blood constituents that are removed by DFPP, in addition to dsDNA and IFN-I, could be involved. Moreover, the involvement of autoantibodies and circulating immune complexes remains unclear owing to the challenges in establishing evaluation methods.
Another limitation of our study is the relatively small sample size, which may reduce the statistical power and generalisability of the findings. The retrospective design is subject to various biases, particularly in data selection and recall. These factors could influence the generalisability of our results to broader populations. Despite these limitations, we believe our study provides valuable insights. However, further research with large, prospective cohorts is needed to confirm these findings. We believe that our study presents an avenue for future research on therapeutic approaches for patients with SLE.
Supplemental Material
Download Zip (4.2 MB)Acknowledgements
The authors are deeply grateful to Moeko Inoue for her invaluable contributions as a research assistant, which significantly enriched the quality and success of this study. The authors also thank the members of the Laboratory of Cell Biology and Laboratory of Molecular and Biochemical Research, Biomedical Research Core Facilities, Juntendo University Graduate School of Medicine for their technical assistance and Editage (www.editage.jp) for English language editing.
Disclosure statement
No potential conflict of interest was reported by the author(s).
Data availability statement
The data underlying this article will be shared, upon reasonable request, with the corresponding author.
Additional information
Funding
References
- Tsokos GC, Lo MS, Costa Reis P, et al. New insights into the immunopathogenesis of systemic lupus erythematosus. Nat Rev Rheumatol. 2016;12(12):716–730. doi: 10.1038/nrrheum.2016.186.
- Rönnblom L, Alm GV, Eloranta M-L. The type I interferon system in the development of lupus. Semin Immunol. 2011;23(2):113–121. doi: 10.1016/j.smim.2011.01.009.
- Crow MK. Type I interferon in the pathogenesis of lupus. J Immunol. 2014;192(12):5459–5468. doi: 10.4049/jimmunol.1002795.
- Crow MK, Olferiev M, Kirou KA. Targeting of type I interferon in systemic autoimmune diseases. Transl Res. 2015;165(2):296–305. doi: 10.1016/j.trsl.2014.10.005.
- Crow MK. Pathogenesis of systemic lupus erythematosus: risks, mechanisms and therapeutic targets. Ann Rheum Dis. 2023;82(8):999–1014. [cited 2024 Jun 5]. Available from: https://ard.bmj.com/content/early/2023/02/14/ard-2022-223741
- Tanaka Y, Kusuda M, Yamaguchi Y. Interferons and systemic lupus erythematosus: pathogenesis, clinical features, and treatments in interferon-driven disease. Mod Rheumatol. 2023;33(5):857–867. doi: 10.1093/mr/roac140.
- Gallucci S, Meka S, Gamero AM. Abnormalities of the type I interferon signaling pathway in lupus autoimmunity. Cytokine. 2021;146:155633. doi: 10.1016/j.cyto.2021.155633.
- Hooks JJ, Moutsopoulos HM, Geis SA, et al. Immune interferon in the circulation of patients with autoimmune disease. N Engl J Med. 1979;301(1):5–8. doi: 10.1056/NEJM197907053010102.
- Ytterberg SR, Schnitzer TJ. Serum interferon levels in patients with systemic lupus erythematosus. Arthritis Rheum. 1982;25(4):401–406. doi: 10.1002/art.1780250407.
- Preble OT, Black RJ, Friedman RM, et al. Systemic lupus erythematosus: presence in human serum of an unusual acid-labile leukocyte interferon. Science. 1982;216(4544):429–431. doi: 10.1126/science.6176024.
- Kirou KA, Lee C, George S, et al. Activation of the interferon-alpha pathway identifies a subgroup of systemic lupus erythematosus patients with distinct serologic features and active disease. Arthritis Rheum. 2005;52(5):1491–1503. doi: 10.1002/art.21031.
- Hua J, Kirou K, Lee C, et al. Functional assay of type I interferon in systemic lupus erythematosus plasma and association with anti-RNA binding protein autoantibodies. Arthritis Rheum. 2006;54(6):1906–1916. doi: 10.1002/art.21890.
- Niewold TB, Adler JE, Glenn SB, et al. Age- and sex-related patterns of serum interferon-alpha activity in lupus families. Arthritis Rheum. 2008;58(7):2113–2119. doi: 10.1002/art.23619.
- Mathian A, Weinberg A, Gallegos M, et al. IFN-alpha induces early lethal lupus in preautoimmune (New Zealand Black × New Zealand White) F1 but not in BALB/c mice. J Immunol. 2005;174(5):2499–2506. doi: 10.4049/jimmunol.174.5.2499.
- Santiago-Raber M-L, Baccala R, Haraldsson KM, et al. Type-I interferon receptor deficiency reduces lupus-like disease in NZB mice. J Exp Med. 2003;197(6):777–788. doi: 10.1084/jem.20021996.
- Braun D, Geraldes P, Demengeot J. Type I Interferon controls the onset and severity of autoimmune manifestations in lpr mice. J Autoimmun. 2003;20(1):15–25. doi: 10.1016/s0896-8411(02)00109-9.
- Kälkner KM, Rönnblom L, Karlsson Parra AK, et al. Antibodies against double-stranded DNA and development of polymyositis during treatment with interferon. Q J Med. 1998;91(6):393–399. doi: 10.1093/qjmed/91.6.393.
- Ehrenstein MR, McSweeney E, Swane M, et al. Appearance of anti-DNA antibodies in patients treated with interferon-alpha. Arthritis Rheum. 1993;36(2):279–280. doi: 10.1002/art.1780360224.
- Rönnblom LE, Alm GV, Oberg KE. Autoimmunity after alpha-interferon therapy for malignant carcinoid tumors. Ann Intern Med. 1991;115(3):178–183. doi: 10.7326/0003-4819-115-3-178.
- Khamashta M, Merrill JT, Werth VP, et al. Sifalimumab, an anti-interferon-α monoclonal antibody, in moderate to severe systemic lupus erythematosus: a randomised, double-blind, placebo-controlled study. Ann Rheum Dis. 2016;75(11):1909–1916. doi: 10.1136/annrheumdis-2015-208562.
- Furie R, Khamashta M, Merrill JT, et al. Anifrolumab, an anti-interferon-α receptor monoclonal antibody, in moderate-to-severe systemic lupus erythematosus. Arthritis Rheumatol. 2017;69:376–386. doi: 10.1002/art.39962.
- Takeuchi O, Akira S. Pattern recognition receptors and inflammation. Cell. 2010;140(6):805–820. doi: 10.1016/j.cell.2010.01.022.
- Chen Q, Sun L, Chen ZJ. Regulation and function of the cGAS–STING pathway of cytosolic DNA sensing. Nat Immunol. 2016;17(10):1142–1149. doi: 10.1038/ni.3558.
- Akira S, Takeda K. Toll-like receptor signalling. Nat Rev Immunol. 2004;4(7):499–511. doi: 10.1038/nri1391.
- Ishikawa H, Barber GN. STING is an endoplasmic reticulum adaptor that facilitates innate immune signalling. Nature. 2008;455(7213):674–678. doi: 10.1038/nature07317.
- Ablasser A, Goldeck M, Cavlar T, et al. cGAS produces a 2′-5′-linked cyclic dinucleotide second messenger that activates STING. Nature. 2013;498(7454):380–384. doi: 10.1038/nature12306.
- Zhang X, Shi H, Wu J, et al. Cyclic GMP-AMP containing mixed phosphodiester linkages is an endogenous high-affinity ligand for STING. Mol Cell. 2013;51(2):226–235. doi: 10.1016/j.molcel.2013.05.022.
- Siegal FP, Kadowaki N, Shodell M, et al. The nature of the principal type 1 interferon-producing cells in human blood. Science. 1999;284(5421):1835–1837. doi: 10.1126/science.284.5421.1835.
- Cella M, Jarrossay D, Facchetti F, et al. Plasmacytoid monocytes migrate to inflamed lymph nodes and produce large amounts of type I interferon. Nat Med. 1999;5(8):919–923. doi: 10.1038/11360.
- Colonna M, Trinchieri G, Liu Y-J. Plasmacytoid dendritic cells in immunity. Nat Immunol. 2004;5(12):1219–1226. doi: 10.1038/ni1141.
- Kwok S-K, Lee J-Y, Park S-H, et al. Dysfunctional interferon-alpha production by peripheral plasmacytoid dendritic cells upon Toll-like receptor-9 stimulation in patients with systemic lupus erythematosus. Arthritis Res Ther. 2008;10(2):R29. doi: 10.1186/ar2382.
- Kawai T, Akira S. The role of pattern-recognition receptors in innate immunity: update on Toll-like receptors. Nat Immunol. 2010;11(5):373–384. doi: 10.1038/ni.1863.
- Murayama G, Furusawa N, Chiba A, et al. Enhanced IFN-α production is associated with increased TLR7 retention in the lysosomes of palasmacytoid dendritic cells in systemic lupus erythematosus. Arthritis Res Ther. 2017;19(1):234. doi: 10.1186/s13075-017-1441-7.
- Murayama G, Chiba A, Kuga T, et al. Inhibition of mTOR suppresses IFNα production and the STING pathway in monocytes from systemic lupus erythematosus patients. Rheumatology. 2020;59(10):2992–3002. doi: 10.1093/rheumatology/keaa060.
- Gall A, Treuting P, Elkon KB, et al. Autoimmunity initiates in nonhematopoietic cells and progresses via lymphocytes in an interferon-dependent autoimmune disease. Immunity. 2012;36(1):120–131. doi: 10.1016/j.immuni.2011.11.018.
- Ahn J, Gutman D, Saijo S, et al. STING manifests self DNA-dependent inflammatory disease. Proc Natl Acad Sci USA. 2012;109(47):19386–19391. doi: 10.1073/pnas.1215006109.
- Liu Y, Jesus AA, Marrero B, et al. Activated STING in a vascular and pulmonary syndrome. N Engl J Med. 2014;371(6):507–518. doi: 10.1056/NEJMoa1312625.
- Baum R, Sharma S, Carpenter S, et al. Cutting edge: AIM2 and endosomal TLRs differentially regulate arthritis and autoantibody production in DNase II-deficient mice. J Immunol. 2015;194(3):873–877. doi: 10.4049/jimmunol.1402573.
- Hirano R, Namazuda K, Hirata N. Double filtration plasmapheresis: review of current clinical applications. Ther Apher Dial. 2021;25(2):145–151. doi: 10.1111/1744-9987.13548.
- Mineshima M. Double filtration plasmapheresis: determination of the optimal albumin concentration in the supplementation fluid. Transfus Apher Sci. 2017;56(5):654–656. doi: 10.1016/j.transci.2017.08.009.
- Connelly-Smith L, Alquist CR, Aqui NA, et al. Guidelines on the use of therapeutic apheresis in clinical practice – evidence-based approach from the Writing Committee of the American Society for Apheresis: the ninth special issue. J Clin Apher. 2023;38(2):77–278. doi: 10.1002/jca.22043.
- Jones JV, Cumming RH, Bucknall RC, et al. Plasmapheresis in the management of acute systemic lupus erythematosus? Lancet. 1976;1(7962):709–711. doi: 10.1016/s0140-6736(76)93088-9.
- Yamaji K, Kim Y-J, Tsuda H, et al. Long-term clinical outcomes of synchronized therapy with plasmapheresis and intravenous cyclophosphamide pulse therapy in the treatment of steroid-resistant lupus nephritis. Ther Apher Dial. 2008;12(4):298–305. doi: 10.1111/j.1744-9987.2008.00591.x.
- Li M, Wang Y, Qiu Q, et al. Therapeutic effect of double-filtration plasmapheresis combined with methylprednisolone to treat diffuse proliferative lupus nephritis: therapeutic effect of DFPP in diffuse proliferative LN. J Clin Apher. 2016;31(4):375–380. doi: 10.1002/jca.21408.
- Al-Adhoubi NK, Bystrom J. Systemic lupus erythematosus and diffuse alveolar hemorrhage, etiology and novel treatment strategies. Lupus. 2020;29(4):355–363. doi: 10.1177/0961203320903798.
- Furuichi K, Wada T. Apheresis for kidney disease. Contrib Nephrol. 2018;196:188–193. doi: 10.1159/000485721.
- Kronbichler A, Brezina B, Quintana LF, et al. Efficacy of plasma exchange and immunoadsorption in systemic lupus erythematosus and antiphospholipid syndrome: a systematic review. Autoimmun Rev. 2016;15(1):38–49. doi: 10.1016/j.autrev.2015.08.010.
- Jones JV, Cumming RH, Bacon PA, et al. Evidence for a therapeutic effect of plasmapheresis in patients with systemic lupus erythematosus. Q J Med. 1979;48(192):555–576.
- Krapf F, Manger B, Koch B, et al. The estimation of circulating immune complexes, C3d, and anti-ds-DNA-antibody serum levels in the monitoring of therapeutic plasmapheresis in a patient with systemic lupus erythematosus. A case report. Clin Exp Rheumatol. 1985;3:159–162.
- Kato Y, Park J, Takamatsu H, et al. Apoptosis-derived membrane vesicles drive the cGAS–STING pathway and enhance type I IFN production in systemic lupus erythematosus. Ann Rheum Dis. 2018;77(10):1507–1515. doi: 10.1136/annrheumdis-2018-212988.
- Tug S, Helmig S, Menke J, et al. Correlation between cell free DNA levels and medical evaluation of disease progression in systemic lupus erythematosus patients. Cell Immunol. 2014;292(1–2):32–39. doi: 10.1016/j.cellimm.2014.08.002.
- Båve U, Magnusson M, Eloranta ML, et al. FcγRIIa is expressed on natural IFN-α-producing cells (plasmacytoid dendritic cells) and is required for the IFN-α production induced by apoptotic cells combined with lupus IgG 1. J Immunol. 2003;171(6):3296–3302. doi: 10.4049/jimmunol.171.6.3296.
- Vallin H, Perers A, Alm GV, et al. Anti-double-stranded DNA antibodies and immunostimulatory plasmid DNA in combination mimic the endogenous IFN-α inducer in systemic lupus erythematosus. J Immunol. 1999;163(11):6306–6313. doi: 10.4049/jimmunol.163.11.6306.
- Lood C, Blanco LP, Purmalek MM, et al. Neutrophil extracellular traps enriched in oxidized mitochondrial DNA are interferogenic and contribute to lupus-like disease. Nat Med. 2016;22(2):146–153. doi: 10.1038/nm.4027.
- Emlen W, Ansari R, Burdick G. DNA-anti-DNA immune complexes. Antibody protection of a discrete DNA fragment from DNase digestion in vitro. J Clin Invest. 1984;74(1):185–190. doi: 10.1172/JCI111400.
- Hartl J, Serpas L, Wang Y, et al. Autoantibody-mediated impairment of DNASE1L3 activity in sporadic systemic lupus erythematosus. J Exp Med. 2021;218(5):e20201138. doi: 10.1084/jem.20201138.
- Heil F, Hemmi H, Hochrein H, et al. Species-specific recognition of single-stranded RNA via toll-like receptor 7 and 8. Science. 2004;303(5663):1526–1529. doi: 10.1126/science.1093620.
- Tanaka Y, Tago F, Yamakawa N, et al. A new therapeutic target for systemic lupus erythematosus: the current landscape for drug development of a toll-like receptor 7/8 antagonist through academia-industry-government collaboration. Immunol Med. 2024;47(1):24–29. doi: 10.1080/25785826.2023.2264023.
- Idborg H, Oke V. Cytokines as biomarkers in systemic lupus erythematosus: value for diagnosis and drug therapy. Int J Mol Sci. 2021;22(21):11327. doi: 10.3390/ijms222111327.
- Caielli S, Wan Z, Pascual V. Systemic lupus erythematosus pathogenesis: interferon and beyond. Annu Rev Immunol. 2023;41(1):533–560. doi: 10.1146/annurev-immunol-101921-042422.
- Möckel T, Basta F, Weinmann-Menke J, et al. B cell activating factor (BAFF): structure, functions, autoimmunity and clinical implications in systemic lupus erythematosus (SLE). Autoimmun Rev. 2021;20(2):102736. doi: 10.1016/j.autrev.2020.102736.
- Navarra SV, Guzmán RM, Gallacher AE, et al. Efficacy and safety of belimumab in patients with active systemic lupus erythematosus: a randomised, placebo-controlled, phase 3 trial. Lancet. 2011;377(9767):721–731. doi: 10.1016/s0140-6736(10)61354-2.
- Zhang F, Bae S-C, Bass D, et al. A pivotal phase III, randomised, placebo-controlled study of belimumab in patients with systemic lupus erythematosus located in China, Japan and South Korea. Ann Rheum Dis. 2018;77(3):355–363. doi: 10.1136/annrheumdis-2017-211631.
- Oke V, Gunnarsson I, Dorschner J, et al. High levels of circulating interferons type I, type II and type III associate with distinct clinical features of active systemic lupus erythematosus. Arthritis Res Ther. 2019;21(1):107. doi: 10.1186/s13075-019-1878-y.
- Gies V, Bekaddour N, Dieudonné Y, et al. Beyond anti-viral effects of chloroquine/hydroxychloroquine. Front Immunol. 2020;11:1409. doi: 10.3389/fimmu.2020.01409.
- Fujio K. Functional genome analysis for immune cells provides clues for stratification of systemic lupus erythematosus. Biomolecules. 2023;13(4):591. doi: 10.3390/biom13040591.
- Northcott M, Gearing LJ, Nim HT, et al. Glucocorticoid gene signatures in systemic lupus erythematosus and the effects of type I interferon: a cross-sectional and in-vitro study. Lancet Rheumatol. 2021;3(5):e357–e370. doi: 10.1016/S2665-9913(21)00006-0.
- Northcott M, Jones S, Koelmeyer R, et al. Type 1 interferon status in systemic lupus erythematosus: a longitudinal analysis. Lupus Sci Med. 2022;9(1):e000625. doi: 10.1136/lupus-2021-000625.
- Casey KA, Guo X, Smith MA, et al. Type I interferon receptor blockade with anifrolumab corrects innate and adaptive immune perturbations of SLE. Lupus Sci Med. 2018;5(1):e000286. doi: 10.1136/lupus-2018-000286.
- Dall’era MC, Cardarelli PM, Preston BT, et al. Type I interferon correlates with serological and clinical manifestations of SLE. Ann Rheum Dis. 2005;64(12):1692–1697. doi: 10.1136/ard.2004.033753.
- Lin SL, Aw E, Zhang Y, et al. Interferon-alpha plays an important role in glial-mediated symptoms of neuropsychiatric systemic lupus erythematosus. J Immunol. 2023;210(1_Supplement):63.19–63.19. doi: 10.4049/jimmunol.210.Supp.63.19.
- Stull D, O’Quinn S, Williams B, et al. Causal cascade of direct and indirect effects of anifrolumab on patient-reported outcomes: structural equation modelling of two phase 3 trials. Rheumatology. 2022;61(12):4731–4740. doi: 10.1093/rheumatology/keac138.
- Morand EF, Furie R, Tanaka Y, et al. Trial of anifrolumab in active systemic lupus erythematosus. N Engl J Med. 2020;382(3):211–221. doi: 10.1056/nejmoa1912196.
- Vital EM, Merrill JT, Morand EF, et al. Anifrolumab efficacy and safety by type I interferon gene signature and clinical subgroups in patients with SLE: post hoc analysis of pooled data from two phase III trials. Ann Rheum Dis. 2022;81(7):951–961. doi: 10.1136/annrheumdis-2021-221425.
- Bradford HF, Haljasmägi L, Menon M, et al. Inactive disease in patients with lupus is linked to autoantibodies to type I interferons that normalize blood IFNα and B cell subsets. Cell Rep Med. 2023;4(1):100894. doi: 10.1016/j.xcrm.2022.100894.
- Tanaka Y. Viewpoint on anifrolumab in patients with systemic lupus erythematosus and a high unmet need in clinical practice. RMD Open. 2023;9(3):e003270. doi: 10.1136/rmdopen-2023-003270.