ABSTRACT
This study was conducted to evaluate the effects of different concentrations of two Polycyclic Aromatic Hydrocarbons (PAHs) on the profiles of soluble proteins and fatty acids and on LacsA (long-chain acyl-CoA synthesis) gene expression in Dunaliella tertiolecta and Dunaliella salina. The algae were treated with a control and three experimental concentrations (3000, 6000 and 9000 μg l‒1) of phenanthrene and anthracene. In D. salina treated with 6000 and 9000 μg l‒1 of anthracene, expression of LacsA and α-linolenic acid was higher than in the control treatment. Also, at 3000 and 6000 μg l‒1 of anthracene, there was a sharp increase in hexadecenoic acid and docosahexaenoic acid (omega-3). Dunaliella tertiolecta treated with 9000 μg l‒1of anthracene showed a stronger protein expression profile than other samples which was consistent with LacsA gene expression at the same dose of anthracene. In D. tertiolecta, α-linolenic acid and 11-octadecenoic acid increased significantly at 9000 µg l‒1 of anthracene compared to the control, and there were similar trends in proteins, LacsA gene expression and α-linolenic acid and 11-octadecenoic fatty acids content. Phenanthrene and anthracene have different molecular structures, and it seems that they also have different dose-dependent effects on synthesis/accumulation of fatty acids, SDS-PAGE profiles of proteins and LacsA gene expression in D. salina and D. tertiolecta. According to previous reports, the LacsA gene is mediated by α-linolenic acid, hexadecenoic acid and docosahexaenoic acid (omega-3) synthesis. Since PAHs tend to be accumulated in the cell membrane of the microalgae, microalgae produce various proteins and fatty acids to bioremediate these harmful substances. Our results indicated that Dunaliella spp. may be good candidates for biodegradation purposes as well as an adequate model as a biotechnological accumulator of fatty acids under exposure to PAHs.
Highlights
LacsA expression and α-linolenic acid increased in Dunaliella exposed to anthracene.
Hexadecenoic acid and omega-3 were produced in D. salina exposed to anthracene.
Phenanthrene and anthracene had dose- and species-dependent effects.
Introduction
Polycyclic Aromatic Hydrocarbons (PAHs) are common petroleum contaminants in the environment (Zhang et al., Citation2019). PAHs are derived from biogenic sources (e.g., forest and prairie fires, natural petroleum seeps) and anthropogenically from incomplete combustion of fossil fuels. Whatever their origin, PAHs have toxic, mutagenic, teratogenic and carcinogenic effects on organisms by inducing oxidative stress (Cerezo & Agusti, Citation2015).
PAHs are composed of carbon and hydrogen in the form of several fused benzene rings. Structurally, based on the numbers and arrangement of the benzene rings, low molecular weight PAHs (such as anthracene and phenanthrene in this study) have two or three rings and high molecular weight PAHs have four or more rings. Phenanthrene and anthracene do not pose a serious risk to humans but are toxic for fish and algae and are common PAHs in aquatic environments (Sutherland et al., Citation1990, Citation1992). Phenanthrene and anthracene are considered as markers for PAHs contamination and as a model to evaluate the effects of environmental factors on bioavailability and biodegradation of PAHs (Bouchez, Blanchet, & Vandecastelle, Citation1995; Kanaly & Harayama, Citation2000; Sutherland, Rafii, Khan, & Cerniglia, Citation1995).
Due to their harmful properties, many studies have been carried out to determine how to remove PAHs from different environments. Biodegradation has been of interest over the past 30 years. In this regard, various bacteria and fungi as well as unicellular algae such as Dunaliella have been identified which are able to biodegrade PAHs (Ji et al., Citation2022; Juhasz & Naidu, Citation2000; Touliabah, El-Sheekh, Ismail, & El-Kassas, Citation2022). Dunaliella (Chlorophyceae, Dunaliellales, Dunaliellaceae) is of particular interest as species such as D. tertiolecta are found in saline lakes and estuaries. Although 2–4% salinity is the optimum range for growth of D. tertiolecta (Arias, Bauza, Tobella, Vila, & Grifoll, Citation2008), it can live in 12% NaCl (Banciu, Enache, Rodriguez, Oren, & Ventosa, Citation2019; Borowitzka & Siva, Citation2007).
Features of the toxicity of PAHs in microalgae are decline in growth rate, biomass, photosynthetic activity and chlorophyll a content, and effects on membrane integrity, lipid content, antioxidant responses and gene expression (Othman, Pick, Hlaili, & Leboulanger, Citation2023). PAHs with hydrophobic characteristics accumulate in membranes and intracellular lipid bodies of microalgae and alter the membrane integrity and function via interaction with acyl chains of the membrane phospholipids (Aksmann & Tukaj, Citation2008; Barhoumi et al., Citation2000; Jimenez, Aranda, Teruel, & Ortiz, Citation2002; Shishlyannikov, Nikonova, Klimenkov, & Gorshkov, Citation2017; Subashchandrabose, Krishnan, Gratton, Megharaj, & Naidu, Citation2014).
Furthermore, PAHs in microalgae may affect lipid metabolism pathways which can result in production of many valuable fatty acids (Croxton, Wikfors, & Schulterbrandt-Gragg, Citation2015). In fatty acids synthesis, intermediates are covalently attached to the sulphhydryl groups of an acyl carrier protein (ACP). Fatty acid synthesis is prolonged by successive addition of two-carbon units derived from acetyl CoA. The activated donor of two-carbon units in the extended phase is a malonyl ACP in which the reaction proceeds with the release of CO2 (McNaught et al., Citation2023). Many enzymes are involved in this process, and the encoding genes such as long-chain acyl-CoA synthesis (LacsA, ID number 29,867) are affected by environmental stress.
LacsA gene expression can be regulated via the internal concentrations of fatty acids such as C18:3 (Lin, Shenm, & Lee, Citation2018). Fatty acid synthesis is dependent on the concentration of PAHs and increase in fatty acid synthesis due to the effects of PAHs on membrane integrity helps to restore membrane structure and function (Lin, Shenm, & Lee, Citation2018). For example, in Thalassiosira pseudonana treated with PAHs, LacsA gene expression was concentration-dependent (Bopp & Lettieri, Citation2007; Othman, Pick, Hlaili, & Leboulanger, Citation2023).
The present study determined the effects of different concentrations of two PAHs (phenanthrene and anthracene) on D. salina and D. tertiolecta. The purpose of this study was to evaluate changes in the profile of fatty acids, soluble proteins and the expression of genes related to the production of fatty acids under PAHs stress. By understanding this relationship, we can predict the possibility of removing these substances using microalgae as well as the use of these algae as a bio-indicator in the future.
Materials and methods
Algal cultures
Dunaliella tertiolecta and D. salina were obtained from the Artemia and Aquaculture Research Institute, Urmia University. First, we determined the optimum physicochemical conditions for algal growth. The optimum growth conditions provided in the growth chamber were 20–25°C temperature and continuous light at 400–500 µmol m‒2 s‒1. The culture medium was modified Walne’s nutrient solution (Walne, Citation1970) enriched with vitamins B12, B1 and Biotin (H) at pH 8–8.5 (Tan, Liew, Shaleh, & Lim, Citation2021). The nutrient solution was aerated by a central pump equipped with filtered pipettes. The growth medium solutions were prepared using Urmia lake water (located in Urmia, western Azerbaijan, Iran), which was filtered, autoclaved and allowed to cool. The optimum salinities for the stock culture of D. tertiolecta (30 g l‒1) and Dunaliella salina (100 g l‒1) were obtained by dissolving 30 g or 100 g of NaCl in 1 l of distiled water. 100 ml of the prepared stocks, 1 ml of modified Walne solution (Walne, Citation1970) and 0.1 ml of vitamin solution were mixed with 898.9 ml of sterile lake water to obtain 1 l of growth medium. The culture vessel was plugged by cotton and aerated using a filtered pipette until the optimum algal concentration was obtained, as described below.
Counting of algae
A Neubauer chamber was used to count the algae. 1 ml of the algal culture medium was transferred to a 50 ml flask, then the algae were fixed by adding a few drops of Lugol´s solution and made up to 50 ml by adding distiled water. One drop of the prepared sample in the chamber was used to count the algae.
Treatments
The algae were treated at the exponential phase of growth when the density of Dunaliella cells was 1.5 × 106 cells ml‒1. The anthracene and phenanthrene solutions were made up from their stock solutions (1 g l−1 in 30% acetone-water mixture) (Chen et al., Citation2018). The target concentrations of anthracene and phenanthrene for each treatment level (3000, 6000 and 9000 μg l‒1), which had been determined by pretreatment experiments, were prepared with sterile lake water.
Extraction and sample preparation
10 ml of the algal suspension from each flask was centrifuged at 3000 g for 5 min to obtain complete sedimentation. 10 ml of deionized water was added to the algal pellet and again mixed well by vortexing in order to break algal cells using deionized water. The resulting mixture was again centrifuged, twice, and the resulting fresh pellet was used for subsequent analyses.
Fatty acid profile
The fatty acid profile of the algae was determined using gas chromatography (GC). 5 ml of solution 1 including methanol and toluene (2:3 v/v) and 5 ml of solution 2 including methanol and acetyl chloride (1:20 v/v) were added with 0.5 g of the algal extracts into a 50 ml test tube. Then, the tubes were put in a hot water bath at 100°C for 1 hr, cooled and the contents were transferred into Falcon tubes. 5 ml of n-hexane and 5 ml of deionized water were added to each of the tubes. The samples were centrifuged for 10 min at 3000 rpm. The supernatant solution was poured into a separate Falcon tube. 3 ml of n-hexane was added to the residual sediment in each of the tubes, and it was again centrifuged and the supernatant was removed. This step was done twice. The samples were dehydrated using sodium sulphate and the solvent was removed with a rotary device at 35°C. Then, 300–500 µl of isooctane was added to each of the samples. The samples were kept in small vials at −30°C until they were used.
Gas chromatography was carried out as follows (Breuer et al., Citation2013). GC vials were filled with the hexane phase and were capped, completely. The wash solvents were hexane, and two blank vials (containing only hexane) were run on the GC before running the samples. Then, the samples were run on a GC-FID with a column (30 m × 0.53 mm × 1.0 μm), inlet temperature (250°C), pressure (81.7 kPa), split ratio (0.1:1), total flow rate (20 ml min‒1) and helium was used as carrier gas. FID detector conditions were set as 270°C, H2 flow rate of 40 ml min‒1, air flow rate of 400 ml min‒1 and He flow rate of 9.3 ml min‒1. 1 μl of each sample was injected for 45 min of total run time including 0.5 min at initial oven temperature (90°C), the time needed for raising the temperature (20°C min‒1) up to 200°C, and 39 min maintained oven temperature (200°C). In chromatograms, the peak area (µV s‒1) value represented fatty acids changes (Oliva & Llabres, Citation2019).
SDS-PAGE profile
Protein extraction and electrophoresis
SDS-PAGE profiles of proteins were determined as follows: the protein samples were mixed with half of the sample loading buffer (3×), boiled for 5 min and then vortexed. The samples were transferred to ice and then centrifuged at 2200 rpm for 1 min and stored in the refrigerator (Coustets, Al-Karablieh, Thomsen, & Teissié, Citation2013).
The resolving gels (10%, pH 8.8) consisted of 2.5 ml acrylamide: bisacrylamide; 3 ml Tris-Cl; 38 µl 20% SDS; 1.9 ml dH2O; 36 µl 10% APS; 5 µl TEMED. The stacking gels (6%, pH 6.8) were prepared using 1 ml acrylamide: bisacrylamide; 630 µl Tris-Cl; 25 µl 20% SDS; 3.6 ml dH2O; 25 µl 10%, APS; 5 µl TEMED. Two glass plates were cleaned with ethanol, placed in a special clamp, then positioned on the base. Resolving and stacking gel ingredients were mixed in separate tubes without adding 10% APS and TEMED. Finally, the last two materials were added to the resolving gel materials, mixed and poured slowly into the space between the two glass plates. Then, isopropanol solution was filled to about 0.5 cm above the gel, left for about 45 min for polymerization, and poured off.
After polymerization of the resolving gel, the stacking gel was prepared with a comb placed between the two glass plates. Then, the last two ingredients, APS (10%) and TEMED, were added to the other gel ingredients that had been prepared and mixed, gently. After gel polymerization (15–30 min), the comb was slowly pulled out. Two glass plates containing gel were separated from the base, fixed in a special clamp and placed inside the tank filled with running buffer. The wells were washed with running buffer using a syringe. Then, 25 µl of the pre-prepared protein samples and 2 µl of the protein marker (BenchMark™ protein ladder) were injected into each well. The lid of the container and the electrodes were fitted, and the gel run at 130 V for 15 min and 150 V for 80 min, until the colour strip was about 0.5 cm from the end of the gel, when the gel was taken out and stained with coomassie brilliant blue G-250 on a shaker.
LacsA gene expression
For RNA extraction from algal cells, 15 mg of D. salina and D. tertiolecta pelleted extract samples from each treatment were weighed. The algal samples were placed in 1.5 ml sterile micro tubes (3 replicates for each treatment), and then immersed in liquid nitrogen for 5 min and completely homogenized in a mortar on an ice container at 4°C. RNA was extracted using the Trizol LS method and manufacturer’s protocol (Invitrogen), then stored at −70°C. RNA was quantified using a biophotometer. Three replicates of each experimental treatment were diluted in a ratio of 4 µl in 400 µl and absorbance recorded at 260 nm (Othman, Pick, Hlaili, & Leboulanger, Citation2023). cDNA was synthesized using a kit protocol (Invitrogen), and PCR was performed. The PCR programme for LacsA was as follows: 2 min at 94°C, 25 s at 94°C, 36 cycles of 25 s at 56°C, 50 s at 72°C), with a final 3 min at 72°C. The primers were as follows: Forward: 5 ́GGCATGTCGTGTGTGGTTT 3 ́, Reverse: 5 ́AGTGGATGCTTGAGTCTC 3 ́ (Bopp & Lettieri, Citation2007). After PCR, 10 µl of the samples were electrophoresed on an agarose gel, photographed and PCR products were analysed.
Statistical analysis
For the experiments, a completely random design with three replicates was conducted. The data were analysed using ANOVA in SPSS 21 Software. Duncan’s multiple range test at 5% significance level was performed to compare mean values.
Results
Fatty acid profile
GC analysis showed a total of 20 different types of fatty acids in D. salina and D. tertiolecta extracts (). The fatty acids profiles of D. salina and D. tertiolecta changed under different concentrations of anthracene and phenanthrene ().
Figure 1. Changes in fatty acids profile under treatment by anthracene (3000, 6000 and 9000 µg l‒1) and control conditions (0 μg l‒1 of anthracene) in Dunaliella tertiolecta (p ≤ 0.05).
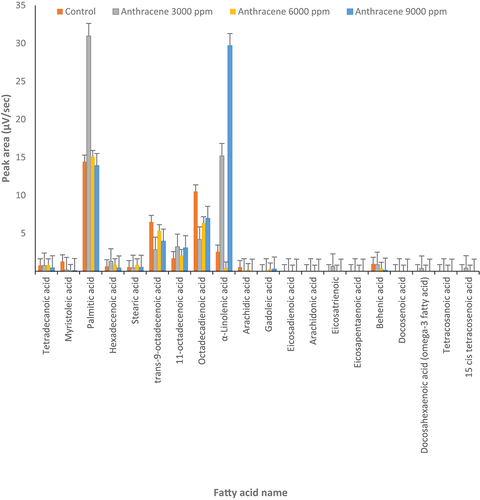
Figure 2. Changes in fatty acids profile under treatment but phenanthrene (3000, 6000 and 9000 µg l‒1) and control conditions (0 μg l‒1 of anthracene) in Dunaliella salina (p ≤ 0.05).
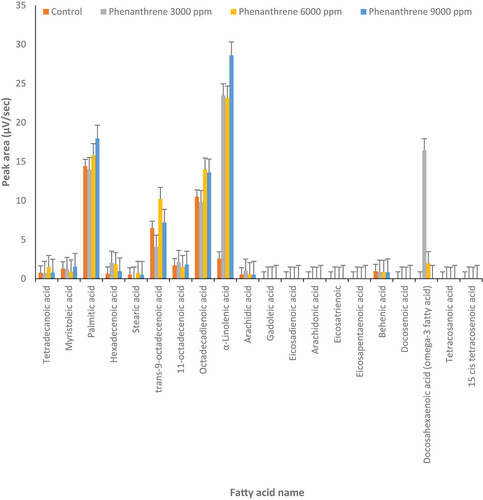
Table 1. Fatty acids found in Dunaliella salina and/or D. tertiolecta (naming based on Lobb & Chow, Citation2015).
In D. tertiolecta, palmitic acid significantly increased twofold with 3000 µg l‒1 of anthracene compared to the control (p ≤0.05) () but not at the higher concentration of anthracene. Also, 11-octadecenoic acid increased at 3000 and 9000 µg l‒1 of anthracene compared to control. The α-linolenic acid increased about sixfold and 12-fold at 3000 and 9000 µg l‒1 of anthracene, respectively. In contrast, under different concentrations of anthracene, trans-9-octadecenoic acid and octadecadienoic acid decreased compared to the control. Docosahexaenoic acid (omega-3 fatty acid) was produced at 3000 µg l‒1 of anthracene but not under control conditions or other concentrations of anthracene. The concentration of other fatty acids did not significantly change at different concentrations of anthracene ().
In D. salina, in the presence of anthracene, α-linolenic acid increased about 10-fold at 6000 and 9000 μg l‒1 compared to the control (). Also, at 3000 and 6000 µg l‒1 of anthracene, hexadecenoic acid increased about fivefold in D. salina. As in D. tertiolecta (), docosahexaenoic acid (omega-3) was produced at 6000 µg l‒1 of anthracene while it was not produced in D. salina under control conditions or other concentrations of anthracene ().
Figure 3. Changes in fatty acids profile under treatment by anthracene (3000, 6000 and 9000 µg l‒1) and control conditions (0 μg l‒1 of anthracene) in Dunaliella salina (p ≤ 0.05).
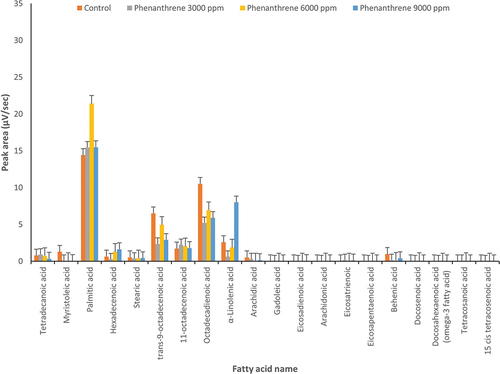
In D. tertiolecta, palmitic acid was increased almost 1.5-fold by 6000 µg l‒1 of phenanthrene as compared to the control (p ≤ 0.05) () while other concentrations of phenanthrene did not affect palmitic acid. Also, α-linolenic acid increased about threefold in the presence of 9000 µg l‒1 of phenanthrene. Conversely, under other concentrations of phenanthrene, trans-9-octadecenoic acid and octadecadienoic acid decreased compared to the control ().
Figure 4. Changes in fatty acids profile under treatment by phenanthrene (3000, 6000 and 9000 µg l‒1) and control conditions (0 μg l‒1 of anthracene) in Dunaliella tertiolecta (p ≤ 0.05).
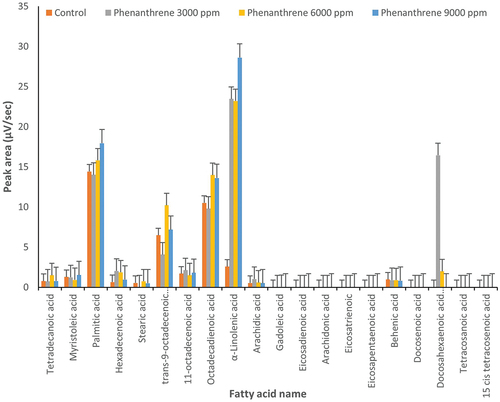
In D. salina, at 6000 μg l‒1 of phenanthrene, palmitic acid and trans-9-octadecenoic acid showed 50% and 60% increases compared to the control, respectively (p ≤ 0.05) (). Octadecadienoic acid increased by 30% at 6000 and 9000 µg l‒1 of phenanthrene. Different concentrations of phenanthrene can cause a significant (8–10 fold) increase of α-linolenic acid content in D. salina. At 3000 and 6000 µg l‒1 of phenanthrene, docosahexaenoic acid (omega-3) rose compared to the control (). Hexadecenoic acid doubled when phenanthrene was applied at 3000 and 6000 µg l‒1 ().
Phenanthrene and anthracene caused changes in SDS-PAGE of Dunaliella salina and D. tertiolecta
Weaker bands were observed in the 3000 μg l‒1 of phenanthrene treatment of D. salina (, samples 5 and 6) which might be related to the low amount of soluble protein. The SDS-PAGE bands were stronger at higher levels of phenanthrene (9000 μg l‒1).
Figure 5. 10% SDS-PAGE gel of soluble proteins of Dunaliella salina (1 and 2: 9000 μg l‒1, 3 and 4: 6000 μg l‒1, 5 and 6: 3000 μg l‒1 phenanthrene).
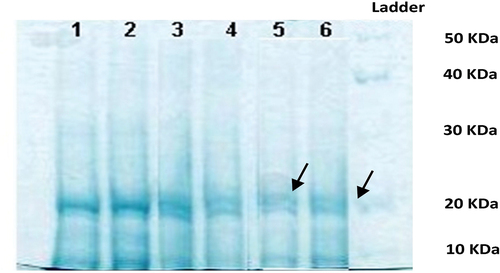
In D. salina, the soluble profile of proteins showed a higher expression of proteins in samples 3 and 4 treated with 6000 μg l‒1 of anthracene (). In this experiment, a decrease in the expression of proteins less than 30 kDa and of 40 kDa proteins was observed.
Figure 6. 10% SDS-PAGE gel of soluble proteins of Dunaliella salina (1 and 2: 9000 μg l‒1, 3 and 4: 6000 μg l‒1, 5 and 6: 3000 μg l‒1 of anthracene).
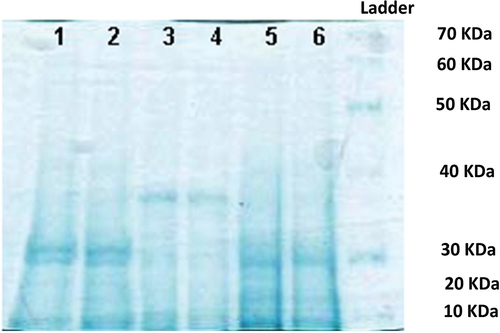
In D. tertiolecta 3000 μg l‒1 of phenanthrene resulted in a big reduction in the amount of the soluble proteins (, lanes 5–6). At 9000 μg l‒1 of phenanthrene (samples number 1 and 2), heavier proteins were observed which appeared to be better resolved than the others ().
Figure 7. 10% SDS-PAGE gel of soluble proteins of Dunaliella tertiolecta (1 and 2: 9000 μg l‒1, 3 and 4: 6000 μg l‒1, 5 and 6: 3000 μg l‒1 of phenanthrene).
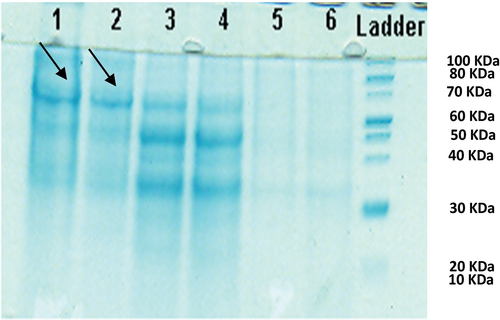
Soluble proteins in D. tertiolecta treated with different concentrations of anthracene showed that at 6000 μg l‒1, there was a high colour intensity compared to the other samples, related to a 30 kDa protein ().
LacsA gene expression under PAHs stress in Dunaliella salina and D. tertiolecta
In order to evaluate LacsA gene expression, new primer combinations were designed. Subsequently, the annealing temperature was checked and the temperature gradient was optimized for this reaction. Based on the melting temperature of the primers, the best temperature for PCR was around 52°C (data not shown).
In this experiment, samples 1–3 were D. tertiolecta treated with phenanthrene at concentrations of 3000, 6000, and 9000 μg l‒1. These three concentrations did not differ from each other but showed lower expression compared to the control (number 4) (). Samples number 5–7 showed LacsA gene expression in D. tertiolecta under anthracene treatment. LacsA gene expression increased greatly at 9000 μg l‒1 () which can be related to the structural difference between anthracene and phenanthrene.
Figure 9. RT-PCR products on electrophoresis gel from Dunaliella salina and D. tertiolecta samples. Samples numbers 1–3: D. tertiolecta treated with phenanthrene at concentrations of 3000, 6000, and 9000 μg l‒1; 5–7: D. tertiolecta treated with 3000, 6000, and 9000 μg l‒1 anthracene; 4: control; 8–10: D. salina treated with 3000, 6000, and 9000 μg l‒1 of phenanthrene; 14: control; 11–13: D. salina treated with 3000, 6000, and 9000 μg l‒1 anthracene, respectively.
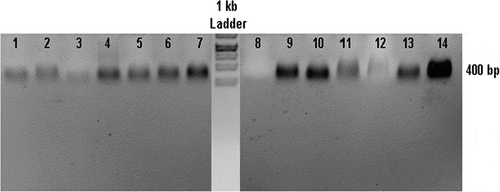
Samples 8–10 correspond to D. salina treated with phenanthrene. LacsA gene expression is much greater at 9000 and 6000 μg l‒1 than at 3000 μg l‒1. Also, D. salina treated with phenanthrene had lower expression than the control sample (number 14) (). In sample number 11, D. salina treated with 3000 μg l‒1 of anthracene, LacsA gene expression was lower than in the control and 9000 μg l‒1.
Discussion
In the present study, phenanthrene and anthracene caused changes in the profiles of soluble proteins and fatty acids. Due to their lipophilic properties, it seems PAHs accumulate in hydrophobic environments such as membranes and induce oxidative stress (Shishlyannikov, Nikonova, Klimenkov, & Gorshkov, Citation2017; Subashchandrabose, Krishnan, Gratton, Megharaj, & Naidu, Citation2014). Therefore, it is anticipated that the PAHs could increase the content of lipids because the increase of lipids causes a better reduction of free PAH in the cell and prevents the binding of PAH to DNA and the formation of a bridge between the two strands (Kottuparambil & Park, Citation2019).
Here should be emphasized that in microalgae, synthesis, degradation and storage of lipids are different from higher plants due to regulatory mechanisms to control fatty acid flux between the different intracellular compartments such as cytosol and plastid (Cagliari et al., Citation2011; Hu et al., Citation2008), whilst fatty acid synthesis, in microalgae, occurs in the plastid and cytosol dependent on the fatty acid type. On the other hand, fundamental changes in LacsA gene expression were observed in the algae under PAHs. According to previous studies, fatty acids are the substrates for the enzymes and proteins (such as LacsA) which provide acyl-ACP in the plastid and acyl-CoA in the cytosol (Fulda, Shockey, Werber, Wolter, & Heinz, Citation2002). The acyl-CoA synthesis (ACSs) enzymes activate free fatty acids into acyl-CoA and are involved in fatty acid transport into different pathways in different cellular compartments resulting in fatty acid elongation (Andrews & Keegstra, Citation1983; Pei et al., Citation2017). There are different groups of ACSs enzymes based on the length of the carbon chain of substrate including medium chain ACSs for C6:0 - C10:0 substrates; long chain ACSs (LacsAs) for C12:0–C20:0 fatty acids and very long-chain ACSs for fatty acids with more than C22:0 (Mashek, Li, & Coleman, Citation2007; Steinberg, Morgenthaler, Heinzer, Smith, & Watkins, Citation2000). Among the ACSs groups, LacsA enzymes are critical for controlling fatty acid metabolism under different environmental conditions. Many enzymes are involved in fatty acid synthesis in the cytosol, with genes affected by environmental stress such as LacsA gene. It has been observed that in the diatom of Thallassiosira pseudonana treated with PAHs, LacsA gene expression was concentration-dependent (Leung, Yi, Ip, Mak, & Leung, Citation2017).
Among diatoms, the hydrophobic interaction of PAHs with acyl chains of the membrane phospholipids could change hydrophobic regions of the membrane and eventually cause loss of integrity and precise function due to membrane expansion and swelling. A cellular pathway to explain the diatom response to Benzo(a)pyrene (BaP) treatment at sub-lethal concentrations has been proposed. In this study lipid metabolism was evaluated along with the genes and enzymes involved in this pathway. It was observed that the PAHs molecule (BaP) reacted with the membrane and caused to its expansion and swelling. BaP can affect cholesterol and fatty acid content in the affected regions of the membrane. Among the 14 genes mediating lipid metabolism, 10 genes were up-regulated and 4 down-regulated by BaP exposure. The genes involved in long-chain phospholipids biosynthesis were up-regulated but the genes involved in lipid degradation down-regulated. These changes may result in fatty acid increase and localization into the membrane to compensate the missing arrangement in the affected regions by PAHs. One of the most important genes is LacsA which is proposed as the biomarker for BaP. Our similar findings in this study suggest LacsA gene as the biomarker of anthracene in D. salina.
Therefore, it can be seen that there is an important correlation between LacsA gene expression and changes in the profile of fatty acids in microalgae, which together with small protein increases act as a defence barrier against PAHs stress. The present study showed that in D. salina, low amounts of soluble protein were observed at 3000 μg l‒1 of phenanthrene and high amounts of protein at 9000 μg l‒1. On the other hand, D. salina treated with phenanthrene had lower LacsA gene expression than the control but the expression at 9000 and 6000 μg l‒1 was more than 3000 μg l‒1. These changes were in contrast to fatty acid profile changes when in D. salina at 6000 μg l‒1 of phenanthrene, octadecadienoic acid (C18:2n6 cis), palmitic acid (C16:0) and trans-9-octadecenoic acid (C18:1n7) were significantly more than the control. All concentration of phenanthrene caused increase in fatty acid α-linolenic acid (C18:3n3) content in D. salina, significantly. Hexadecenoic acid (C16:1n7) was increased and docosahexaenoic acid (C22:6n3; omega-3) showed increase due to 3000 and 6000 μg l‒1 of phenanthrene as compared to control. In total when D. salina was exposed to different doses of phenanthrene, it showed a significant reduction in the amount of LacsA gene expression while at the same time C18 fatty acids especially α-linolenic acid (C18:3n3) showed an increase.
In D. salina, at 6000 μg l‒1 of anthracene, lower expression of 30 kDa and 40 kDa proteins was observed compared to the control. In contrast, in D. salina treated with 6000 and 9000 μg l‒1 of anthracene, LacsA gene expression and α-linolenic acid (C18:3n3) were significantly higher than in the control. Also, at 3000 and 6000 μg l‒1 of anthracene, hexadecenoic acid (C16:1n7) increased and docosahexaenoic acid (omega-3) was produced. It seems that there was a relationship between anthracene dose, LacsA gene upregulation and α-linolenic acid (C18:3n3), hexadecenoic acid (C16:1n7) and docosahexaenoic acid (C22:6n3; omega-3) content in D. salina. It should be noted that this result is in contrast to phenanthrene. In this research, the different effects of anthracene and phenanthrene on D. salina may be related to their structural difference because both molecules have the same molecular weight (178.2) with 3 benzene rings. The linear structure of anthracene and the angular one in phenanthrene may cause different physical and chemical properties such as different solubility and bioavailability. The linear structures such as anthracene are more stable and less soluble (water solubility 0.64 mg l‒1) than the angular ones such as phenanthrene (water solubility 1.24 mg l‒1) due to higher availability of the angular structure to the degradable enzymes in the bay regions between the benzene rings (Okere & Semple, Citation2012).
In contrast, D. tertiolecta at 3000 μg l‒1 of phenanthrene revealed a reduction in protein transcription compared to 9000 μg l‒1 at which heavier proteins with sharper bands were observed. Under different concentration of phenanthrene, trans-9-octadecenoic acid (C18:1n7) and octadecadienoic acid (C18:2n6 cis) depicted reduction as compared to control. We found that in D. tertiolecta treated with phenanthrene, LacsA gene expression did not change. Similarly, palmitic acid (C16:0) did not change at 3000 and 9000 μg l‒1 of phenanthrene as compared to control. Inversely, α-linolenic acid (C18:3n3) revealed an increase at 9000 μg l‒1 of phenanthrene.
At 9000 μg l‒1 of anthracene in D. tertiolecta there was a stronger pattern of proteins compared to other samples. Similarly, LacsA gene expression greatly increased at 9000 μg l‒1 of anthracene. Also, α-linolenic acid (C18:3n3) and 11-octadecenoic acid (C18:1n9) revealed upregulation at 9000 μg l‒1 of anthracene as compared to control. The similar trend in protein pattern, LacsA gene expression and also α-linolenic acid and 11-octadecenoic acid fatty acids content at 9000 μg l‒1 of anthracene, may represent interactions between different fatty acid biosynthesis pathways in the cells. Also, in both species of Dunaliella, similar variation in LacsA gene expression and α-linolenic acid content was observed at the highest dose of anthracene which is correlated with other researches on diatoms. These changes may be due to the high level of anthracene in the cell membrane and increases in native fatty acid in the membrane to alleviate adverse effects of PAHs on the cell (Bolik et al., Citation2022). Similar variations in fatty acid composition including palmitic acid (C16:0), 11-octadecenoic acid (C18:1n9) and α-linolenic acid (C18:3n3) were observed in D. tertiolecta at 3000 μg l‒1 of anthracene. In our study, docosahexaenoic acid (omega-3 fatty acid) was found to be increasing only at 3000 μg l‒1 of anthracene. It is likely related to the dose-dependent expression of LacsA gene which mediating the fatty acids biosynthesis (Othman, Pick, Hlaili, & Leboulanger, Citation2023).
Our study revealed that in both species of algae, changes in proteins and α-linolenic acid (C18:3n3) content had similar trends but different results were obtained for other fatty acids and LacsA gene expression, under different dose of phenanthrene. It supports the idea that LacsA gene expression and fatty acids profiles are species dependent. Regarding other research on D. tertiolecta, it seems that C18:3 type of fatty acids are the regulatory compounds which can control fatty acid flux within the cell. In fact, the cellular content of C18 fatty acid can negatively regulate the expression levels of the genes mediating the upstream fatty acid synthesis cycle, such as the LacsA gene. It is likely that the feedback control mechanism is that when the C18 fatty acid reaches a certain level, the LacsA gene is down-regulated to control the fatty acid biosynthesis and lipid accumulation and consequently maintain the fatty acid profile in D. tertiolecta. Any variation in fatty acid profile may affect the fatty acid composition of membranes and eventually impairs the membrane integrity and exerts adverse effects on the cell (Millar, Smith, & Kunst, Citation2000).
According to our results (not shown here), PAHs had dose-dependent harmful effects on the amount of chlorophyll and photosynthesis. The preliminary increase of 16:3 ω4 and 18:3 ω3 indicates restoration of photosynthetic activity (Bolik, Albrieux, Schneck, Demé, & Jouhet, Citation2022). In this regard, Morales-Loo and Goutx (Citation1990) reported that D. tertiolecta treated with crude oil showed an increase in sterol synthesis (Morales-Loo & Goutx, Citation1990). The synthesis of digalactosyl-diacyl-glycerol and the intensification of 3ω unsaturation indicate the initial stages of the photosystem I reconstruction process (Nikookar, Moradshahi, & Hosseini, Citation2005). The metabolism of phospholipids is effective on membrane characteristics and permeability. Studies have shown that phosphatidyl glycerol is involved in photosynthetic electron transfer and increasing it leads to better photosynthetic performance (Bolik, Albrieux, Schneck, Demé, & Jouhet, Citation2022). The increase in lipid content is probably due to the increase in glycerol, to maintain the osmotic state and prevent the entry of NaCl in the algae (Cruz Powell, Citation2021).
Also, the results may support other research where microalgae exposure to PAHs has caused up-regulation of some genes such as LacsA and related encoded proteins mediating the metabolism and content of lipid in the cells. Biodegradation mechanisms to remove PAHs from the media are complicated due to the hydrophobic PAHs entering the hydrophobic regions of the membrane (González-Gaya et al., Citation2019). It was shown that PAHs including pyrene, fluoranthene and benzo-pyrene caused LacsA upregulation and an increase in the long-chain acyl-CoA synthesis enzyme involved in lipid metabolism in diatoms (Bopp & Lettieri, Citation2007).
It can be concluded that phenanthrene and anthracene had dose- and species-dependent effects on the synthesis and/or accumulation of fatty acids, the SDS-PAGE profile of proteins and LacsA gene expression in D. salina and D. tertiolecta. It seems that the LacsA gene may be mediated in α-linolenic acid, hexadecenoic acid and docosahexaenoic acid (omega-3) synthesis. α-linolenic acid can be suggested as a regulatory molecule to control fatty acid biosynthesis in D. salina and D. tertiolecta cells via changes in LacsA gene expression. D. salina and D. tertiolecta may be suggested as good candidates to accumulate fatty acids under contamination of PAHs such as phenanthrene and anthracene, probably via changes in LacsA gene expression. Thereby, further research is needed to find the regulation of LacsA gene expression under PAHs stress using blockers in fatty acids biosynthesis pathways.
Author contributions
J. Sajadinasab: original concept, drafting and editing manuscript, experimental design, culture experiments; R. Manaffar: analysis of gene expression and GC, drawing the graphs; H. Siavash: editing the manuscript; L. Porakbar: supplying reagent.
Acknowledgements
The authors wish to thank Dr. Imaneh Dehghani for editing the paper. Financial support by Urmia University is gratefully acknowledged.
Disclosure statement
No potential conflict of interest was reported by the author(s).
References
- Aksmann, A., & Tukaj, Z. (2008). Intact anthracene inhibits photosynthesis in algal cells: A fluorescence induction study on Chlamydomonas reinhardtii cw92 strain. Chemosphere, 74, 26–32. doi:10.1016/j.chemosphere.2008.09.064
- Andrews, J., & Keegstra, K. (1983). Acyl-CoA synthetase is located in the outer membrane and acyl-CoA thioesterase in the inner membrane of pea chloroplast envelopes. Plant Physiology, 72, 735‒740. doi:10.1104/pp.72.3.735
- Arias, L., Bauza, J., Tobella, J., Vila, J., & Grifoll, M. (2008). A microcosm system and an analytical protocol to assess PAH degradation and metabolite formation in soils. Biodegradation, 19, 425–434. doi:10.1007/s10532-007-9148-0
- Banciu, H. L., Enache, M., Rodriguez, R. M., Oren, A., & Ventosa, A. (2019). Ecology and physiology of halophilic microorganisms–Thematic issue based on papers presented at Halophiles 2019–12th International Conference on Halophilic Microorganisms, Cluj-Napoca, Romania, 24–28 June, 2019. FEMS Microbiology Letters, 366, fnz250. doi:10.1093/femsle/fnz250
- Barhoumi, R., Mouneimne, Y., Ramos, K. S., Safe, S. H., Phillips, T. D., Centonze V. E., Ainley C., Gupta M. S., & Burghardt R. C. (2000). Analysis of benzo[a]pyrene partitioning and cellular homeostasis in a rat liver cell line. Toxicological Sciences, 53, 264–270. doi:10.1093/toxsci/53.2.264
- Bolik, S., Albrieux, C., Schneck, E., Demé, B., & Jouhet, J. (2022). Sulfoquinovosyldiacylglycerol and phosphatidylglycerol bilayers share biophysical properties and are good mutual substitutes in photosynthetic membranes. Biochimica Et Biophysica Acta (BBA)-Biomembranes, 1864, 184037. doi:10.1016/j.bbamem.2022.184037
- Bopp, S. K., & Lettieri, T. (2007). Gene regulation in the marine diatom Thalassiosira pseudonana upon exposure to polycyclic aromatic hydrocarbons (PAHs). Gene, 396, 293‒302. doi:10.1016/j.gene.2007.03.013
- Borowitzka, M. A., & Siva, C. J. (2007). The taxonomy of the genus Dunaliella (Chlorophyta, Dunaliellales) with emphasis on the marine and halophilic species. Journal of Applied Phycology, 19, 567–590. doi:10.1007/s10811-007-9171-x
- Bouchez, M., Blanchet, D., & Vandecastelle, J. P. (1995). Degradation of polycyclic aromatic hydrocarbons by pure strains and by defined strain associations: Inhibition phenomena and cometabolism. Applied Microbiology and Biotechnology, 43, 156‒164. doi:10.1007/BF00170638
- Breuer, G., Evers, W. A. C., de Vree, J. H., Kleinegris, D. M. M., Martens, D. E., Wijffels, R. H., & Lamers, P. P. (2013). Analysis of fatty acid content and composition in microalgae. Journal of Visualized Experiments, 80, e50628. doi:10.3791/50628-v
- Cagliari, A., Margis, R., dos Santos Maraschin, F., Turchetto-Zolet, A. C., Loss, G., & Margis-Pinheiro, M. (2011). Biosynthesis of Triacylglycerols (TAGs) in plants and algae. International Journal of Plant Biology, 2, e10. doi:10.4081/pb.2011.e10
- Cerezo, M. I., & Agusti, S. (2015). PAHs reduce DNA synthesis and delay cell division in the widespread primary producer prochlorococcus. Environmental Pollution, 196, 147–155. doi:10.1016/j.envpol.2014.09.023
- Chen, H., Zhang, Z., Tian, F., Zhang, L., Li, Y., Cai, W., & Jia, X. (2018). The effect of pH on the acute toxicity of phenanthrene in a marine microalgae Chlorella salina. Scientific Reports, 8, 175‒177. doi:10.1038/s41598-018-35686-9
- Coustets, M., Al-Karablieh, N., Thomsen, C., & Teissié, J. (2013). Flow process for electroextraction of total proteins from microalgae. The Journal of Membrane Biology, 246, 751‒760. doi:10.1007/s00232-013-9542-y
- Croxton, A. N., Wikfors, G. H., & Schulterbrandt-Gragg, R. D., III. (2015). The use of flow cytometric applications to measure the effects of PAHs on growth, membrane integrity, and relative lipid content of the benthic diatom, nitzschia brevirostris. Marine Pollution Bulletin, 91, 160‒165. doi:10.1016/j.marpolbul.2014.12.010
- Cruz Powell, I. A. (2021). Characterization of a novel glycerol-3-phosphate dehydrogenase (GPD2) in the alga Chlamydomonas reinhardtii. Dissertations and theses in biological sciences. Lincoln: University of Nebraska. Retrieved from https://digitalcommons.unl.edu/bioscidiss/117)
- Fulda, M., Shockey, J., Werber, M., Wolter, F. P., & Heinz, E. (2002). Two long‐chain acyl‐CoA synthetases from Arabidopsis thaliana involved in peroxisomal fatty acid β‐oxidation. The Plant Journal: For Cell and Molecular Biology, 32, 93‒103. doi:10.1046/j.1365-313X.2002.01405.x
- González-Gaya, B., Martínez-Varela, A., Vila-Costa, M., Casal, P., Cerro-Gálvez, E., Berrojalbiz, N., Lundin, D., Vidal, M., Mompeán, C., Bode, A., & Jiménez, B. (2019). Biodegradation as an important sink of aromatic hydrocarbons in the oceans. Nature geoscience, 12(2), 119–125. doi:10.1038/s41561-018-0285-3.
- Hu, Q., Sommerfeld, M., Jarvis, E., Ghirardi, M., Posewitz, M., Seibert, M., & Darzins, A. (2008). Microalgal triacylglycerols as feedstocks for biofuel production: Perspectives and advances. The Plant Journal: For Cell and Molecular Biology, 54, 621‒639. doi:10.1111/j.1365-313X.2008.03492.x
- Jimenez, M., Aranda, F. J., Teruel, J. A., & Ortiz, A. (2002). The chemical toxic benzo[a]pyrene perturbs the physical organization of phosphatidylcholine membranes. Environmental Toxicology and Chemistry, 21, 787–793. doi:10.1002/etc.5620210415
- Ji, Z., Yang, Y., Zhu, Y., Ling, Y., Ren, D., Huo, Z., & Zhang, N. (2022). Toxic effects on Dunaliella salina. Mysidopsis Bahia, and Mugilogobius Chulae from Ship Exhaust Gas Closed-Loop Scrubber Wash Water Research Square, 1, 10.21203/rs.3.rs–1324578/v1.
- Juhasz, A. L., & Naidu, R. (2000). Bioremediation of high molecular weight polycyclic aromatic hydrocarbons: A review of the microbial degradation of benzo [a] pyrene. International Biodeterioration & Biodegradation, 45, 57–88. doi:10.1016/S0964-8305(00)00052-4
- Kanaly, R. A., & Harayama, S. (2000). Biodegradation of high-molecular weight polycyclic aromatic hydrocarbons by bacteria. Journal of Bacteriology, 182, 2059–2067. doi:10.1128/JB.182.8.2059-2067.2000
- Kottuparambil, S., & Park, J. (2019). Anthracene phytotoxicity in the freshwater flagellate alga Euglena agilis Carter. Scientific Reports, 9, 15323. doi:10.1038/s41598-019-51451-y
- Leung, P. T., Yi, A. X., Ip, J. C., Mak, S. S., & Leung, K. M. (2017). Photosynthetic and transcriptional responses of the marine diatom Thalassiosira pseudonana to the combined effect of temperature stress and copper exposure. Marine Pollution Bulletin, 124, 938‒945. doi:10.1016/j.marpolbul.2017.03.038
- Lin, H., Shenm, H., & Lee, Y. K. (2018). Cellular and molecular responses of Dunaliella tertiolecta by expression of a plant medium chain length fatty acid specific acyl-acp thioesterase. Frontiers in Microbiology, 9, 619. doi:10.3389/fmicb.2018.00619
- Lobb, K., & Chow, C. K. (2015). Fatty acid classification and nomenclature. In C. K. Chow (Ed.), Fatty acids in foods and their health implications (pp. 1‒5). Oklahoma State University. doi:10.1201/9781420006902.
- Mashek, D. G., Li, L. O., & Coleman, R. A. (2007). Long-chain acyl-CoA synthetases and fatty acid channeling. Future Lipidology, 2, 465‒476. doi:10.2217/17460875.2.4.465
- McNaught, K. J., Kuatsjah, E., Zahn, M., Prates, É. T., Shao, H., Bentley G. J., Pickford A. R., Gruber J. N., Hestmark K. V., Jacobson D. A., & Poirier, B. C. (2023). Initiation of fatty acid biosynthesis in Pseudomonas putida KT2440. Metabolic Engineering, 76, 193‒203. doi:10.1016/j.ymben.2023.02.006
- Millar, A. A., Smith, M. A., & Kunst, L. (2000). All fatty acids are not equal: Discrimination in plant membrane lipids. Trends in Plant Science, 5, 95‒101. doi:10.1016/S1360-1385(00)01566-1
- Morales-Loo, M. R., & Goutx, M. (1990). Effects of water-soluble fraction of the Mexican crude oil “Isthmus Cactus” on growth, cellular content of chlorophyll a, and lipid composition of planktonic microalgae. Marine Biology, 104, 503‒509. doi:10.1007/BF01314357
- Nikookar, K., Moradshahi, A., & Hosseini, L. (2005). Physiological responses of Dunaliella salina and Dunaliella tertiolecta to copper toxicity. Biomolecular Engineering, 22, 141‒146. doi:10.1016/j.bioeng.2005.07.001
- Okere, U., & Semple, K. (2012). Biodegradation of PAHs in ‘pristine’soils from different climatic regions. Journal of Bioremediation and Biodegradation S, 1, 1‒11. doi:10.4172/2155-6199.S1-006
- Oliva, A., & Llabres, M. (2019). Validation of a size-exclusion chromatography method for bevacizumab quantitation in pharmaceutical preparations: Application in a biosimilar study. Separations, 6, 43. doi:10.3390/separations6030043
- Othman, H. B., Pick, F. R., Hlaili, A. S., & Leboulanger, C. (2023). Effects of polycyclic aromatic hydrocarbons on marine and freshwater microalgae–A review. Journal of Hazardous Materials, 441, 129869. doi:10.1016/j.jhazmat.2022.129869
- Pei, G., Li, X., Liu, L., Liu, J., Wang, F., Chen, L., & Zhang, W. (2017). De novo transcriptomic and metabolomic analysis of docosahexaenoic acid (DHA)-producing Crypthecodinium cohnii during fed-batch fermentation. Algal Research, 26, 380‒391. doi:10.1016/j.algal.2017.07.031
- Shishlyannikov, S. M., Nikonova, A. A., Klimenkov, I. V., & Gorshkov, A. G. (2017). Accumulation of petroleum hydrocarbons in intracellular lipid bodies of the freshwater diatom Synedra acus subsp. radians. Environmental Science and Pollution Research, 24, 275‒283. doi:10.1007/s11356-016-7782-y
- Steinberg, S. J., Morgenthaler, J., Heinzer, A. K., Smith, K. D., & Watkins, P. A. (2000). Very long-chain acyl-CoA synthetases: Human “bubblegum” represents a new family of proteins capable of activating very long-chain fatty acids. Journal of Biological Chemistry, 275, 35162‒35169. doi:10.1074/jbc.M006403200
- Subashchandrabose, S. R., Krishnan, K., Gratton, E., Megharaj, M., & Naidu, R. (2014). Potential of fluorescence imaging techniques to monitor mutagenic PAH uptake by microalga. Environmental Science & Technology, 48, 9152‒9160. doi:10.1021/es500387v
- Sutherland, J. B., Freeman, J. P., Selby, A. L., Fu, P. P., Miller, D. W., & Cerniglia, C. E. (1990). Stereoselective formation of a K-region dihydrodiol from phenanthrene by Streptomyces flavovirens. Archives of Microbiology, 154, 260–266. doi:10.1007/BF00248965
- Sutherland, J. B., Rafii, F., Khan, A. A., & Cerniglia, C. E. (1995). Mechanisms of polycyclic aromatic hydrocarbon degradation. In L. Y. Young & C. E. Cerniglia (Eds.), Microbial transformation and degradation of toxic organic chemicals (pp. 169–306). New York, N.Y: Wiley-Liss.
- Sutherland, J. B., Selby, A. L., Freeman, J. P., Fu, P. P., Miller, D. W., & Cerniglia, C. E. (1992). Identification of xyloside conjugates formed from anthracene by Rhizoctonia solani. Mycological Research, 96, 509–517. doi:10.1016/S0953-7562(09)81100-3
- Tan, N. H., Liew, K. S., Shaleh, S. R. M., & Lim, L. S. (2021). Growth performance of brown-golden marine microalga, Isochrysis sp. cultivated in alternative algal culture media. Borneo Journal of Marine Science and Aquaculture (BjoMSA), 5, 57‒62. doi:10.51200/bjomsa.v5i2.3150
- Touliabah, H. E., El-Sheekh, M. M., Ismail, M. M., & El-Kassas, H. (2022). A review of microalgae- and cyanobacteria-based biodegradation of organic pollutants. Oxycedrus Needles and Berries Molecules, 27, 1141. doi:10.3390/molecules27031141
- Walne, P. R. (1970). Studies on the food value of nineteen genera of algae to juvenile bivalves of the genera Ostrea, Crassostrea, Mercenaria, and Mytilis. Fish Investigation, 26, 1–62.
- Zhang, X., Yu, T., Li, X., Yao, J., Liu, W., Chang, S., & Chen, Y. (2019). The fate and enhanced removal of polycyclic aromatic hydrocarbons in wastewater and sludge treatment system: A review. Critical Reviews in Environmental Science and Technology, 49, 1425–1475. doi:10.1080/10643389.2019.1579619