ABSTRACT
Because of its unique reductive properties, nanoscale zero-valent iron (NZVI) has been widely used in soil and groundwater remediation. It is crucial to understand the fate of NZVI in soils. In this work, a range of laboratory experiments was conducted to examine the adsorption, transformation, and transport of NZVI. The NZVI particles showed relatively strong sorption onto vertic shajiang-aquic cambosols, typic-hapli-udic argosols and typic ochri-aquic cambosols and isotherms can be well described by the Langmuir model. When cultivated in the soils, the NZVI was oxidized within seven days and the particle size also increased with average size reaching around 100 nm at 28 days. Columns were packed with quartz sand, sandy soil and diatomite as saturated porous media to evaluate the role of soil colloids in affecting the transport of NZVI. The results showed that NZVI transport in the columns was strongly affected by medium types, dispersion agent types, and soil colloids. Comparisons of breakthrough curves of different types of dispersed NZVI and corresponding colloid-NZVI revealed that natural soil colloids facilitated NZVI transport in porous media. Findings from this work can provide useful information to better evaluate the applications and impacts of NZVI as an environmental remediation agent.
1. Introduction
Because of its special physicochemical properties, nanoscale zero-valent iron (NZVI) becomes one of the most popular metal nano materials and is widely used in the field of electronic industry, chemical industry, medicine and so on [Citation1–Citation3]. With strong reducibility and large specific surface area, NZVI is also regarded as a good pollution remediation agent that attracts much research interest [Citation4–Citation6]. Although the technology that uses NZVI to treat pollutants in soils and water has been developed, there are still some issues related to the nature and destiny of NZVI applied in soil that need to be solved [Citation7,Citation8]. For example, the NZVI could be coalescence thus greatly reduce the reaction surface area in soil [Citation9–Citation11]. When entered into the soil, the NZVI is easily oxidized and forms a passivation layer, which reduces its activity and thus limits the practical applications [Citation12–Citation14]. To address these issues, various surface modification methods have been used to change the surface properties of NZVI to improve its stability [Citation15], which has promoted the applications of this novel technology. However, the theoretical mechanism of NZVI’ adsorption, migration and transformation in soil was still remains unclear.
It is worth noting that NZVI is often retained in soils through adsorption when it is applied to the soil systems. The retention and accumulation of NZVI onto different types of soils thus have attracted much research interest [Citation16–Citation19]. A large number of literature has reported that the adsorbed NZVI particles can be coalesced in soils and gradually lose their activities [Citation20–Citation23]. In addition, there are also a few studies reporting the release of NZVI particles from sludge [Citation24–Citation28]. A new synthesized NZVI is usually easy to be spontaneously oxidized when it is exposed to the air [Citation29]. This will wrap a layer of iron oxide to form a core-shell structure on the NZVI that stops the rapid oxidation [Citation30–Citation35]. This protective layer of iron oxide can reach about 5 nm thickness to prevent further oxidation [Citation36–Citation38]. When NZVI is immersed into water, however, the protection layer may be broken so that the NZVI will gradually lose its activity [Citation39–Citation41]. Because there is always water in soil pores, it is anticipated the adsorbed NZVI in soils might also lose its activity due to the contact with water [Citation42,Citation43]. Nevertheless, little research has been conducted to determine the transformation of NZVI in soils.
Transport and migration of NZVI in porous media have been well studied in the literature [Citation44–Citation49]. The transport of NZVI particles in saturated porous media is mainly affected by the nature of flow, particle, and media such as surfactant type, flow rate, solution chemistry (pH, ionic strength), type and concentration of nano material, and medium properties [Citation50–Citation56]. Because soil particles have strong sorption ability to NZVI, one would expect that NZVI should have limited mobility in soil and groundwater systems under most of the environmental conditions [Citation53]. However, as colloids are ubiquitous in soils, the nanoparticle would also be adsorbed onto colloids and thus be transported when the colloids are released from soils [Citation57]. Colloid-facilitated transport of contaminants including heavy metals, pesticides, antibiotics, radionuclides, etc., has been reported in many previous studies [Citation58,Citation59]. To the best of the authors’ knowledge, however, none of the previous studies have examined the colloid-facilitated transport of NZVI in porous media on the adsorption of nano materials in soil and synergetic migration between NZVI and soil colloid.
The overarching objective of this study was to understand the sorption, transformation, and colloid-facilitated transport of NZVI in soils under various conditions. NZVI particles were synthesized and dispersed with the aid of different surfactants. A range of laboratory experiments was conducted to determine the adsorption and transformation behaviors in soils. Column breakthrough experiments were also performed to reveal the governing mechanisms of colloid-facilitated transport of the nanoparticles.
2. Materials and methods
2.1. Materials
The NZVI particles were synthesized in laboratory following the procedures of Li et al., 2015 [Citation31]. First, nitrogen gas (N2) was imported into deionized (DI) water for 30 min to dislodge the dissolved oxygen. Then, 10 g of analytical grade FeSO4 · 7H2O was dissolved in 100 mL of 30% ethanol and 70% DI water (v/v) under N2 atmosphere. Polyvinyl pyrrolidone (PVP-K30, 5%),cetyltrimethyl ammonium bromide (CTAB) or sodium dodecyl sulfonate (SDS) was added into the solution to make the NZVI distribute evenly in solute as the dispersion agent. The pH of the solutions was adjusted to about 6.8 with 3.8 M NaOH and KBH4 powder (1.8 g) was added gradually. The mixture was stirred with a homogenizer (Ultra Turrax IKA T25, IKA Instrument Equipment Co., LTD, Germany) for 20 min, followed by filtration using 0.2 μm membrane ceramic filter (Millipore). The particles were washed with DI water, rinsed with acetone to remove the rest of NaBH4 and water, and vacuum dried (DZG-6050SA, Shanghai Samsung Laboratory Instrument Co., LTD, China). For each experiment, fresh PVP dispersed NZVI (PVP-NZVI), CTAB dispersed NZVI (CTAB-NZVI) and SDS dispersed NZVI (SDS-NZVI) were made and used immediately. The dispersion ability of the four dispersion agents was determined.
typic-hapli-udic argosols, typic ochri-aquic cambosols and vertic shajiang-aquic cambosols (Chinese Soil Taxonomy System) were collected from 10 to 40 cm top soil layer locally. The soil samples were air dried and gently crushed, and then passed through 60 and 100 mesh sieves. The three soils were used in the adsorption and transformation experiments. Basic properties of the soils were determined following the standard procedures, and the results are shown in the supporting information (Table S1).
Quartz sand was obtained locally and sieved into 60–80 mesh. The sand was soaked using 20% hydrochloric acid for 24 h, wash it with tap water and then DI water to pH of 6.0–7.0, and dried at 65 ℃. Sandy soil was also collected locally and dried at 65°C prior to the experiments. Diatomite was obtained from Tianjin Kemiou Chemical.
Reagent Co., Ltd. They were used as the packed media in the column transport experiments. Basic physicochemical properties of the quartz sand, sandy soil and diatomite are also shown in the supporting information (Table S2).
2.2. NZVI characterization
The morphologies of the NZVI particles were examined using scanning electron microscopy (SEM, Zeiss microscope EVO LS15, Germany). The samples were coated with a gold layer prior to the analysis. Transmission electron microscopy (TEM, JEM-1400,Japan) at 120 kV was used to further examine the morphology of the NZVI particles and to determine their size distributions. After vacuum drying, the NZVI samples were measured for both full spectrum scan and iron element for narrow sweep using X-ray Photoelectron Spectroscopy (XPS, Thermo escalab 250Xi, USA).
2.3. Adsorption isotherms of NZVI onto soils
To determine the adsorption isotherms of NZVI on the three types of soils, 2.5 g of each soil was added into 50 mL centrifuge tubes. The NZVI suspensions (50 mL) with different concentrations (0, 50, 100, 200, 400, 800, 1000 mg/L) were added into the tubes, and a control (without soil) was also performed. The mixtures were vibrated at 220 rpm for 24 h under 25 ± 1 ℃. The centrifuge tubes were then centrifuged under the conditions of 4000 rpm for 20 min. To determine the aqueous phase equilibrium NZVI concentrations, 6 mL of the supernatants was taken and digested with 3 mL of concentrated hydrochloric acid under heated conditions and analyzed with an atomic absorption spectrophotometer (AAS, AA-7000, Japan) for the total iron concentrations. The final NZVI amount adsorbed by soil = [(the original NZVI concentrations – the measured NZVI of supernatant) – (the original NZVI concentrations – the measured NZVI concentrations of control)] V/M (V on behalf of the volume of the solution; M was the soil weight) [Citation16].
2.4. Adsorption kinetics of NZVI onto soils
The adsorption kinetics were measured using 2.5 g of each soil mixed with 50 mL NZVI at a concentration of 400 mg/L in 50 mL centrifuge tubes. The samples were vibrated at constant temperature (25 ± 1 ℃), 220 rpm for different time intervals (0.5, 1, 2, 4, 8, 12, 24, or 48 h). After centrifuge, NZVI concentrations in the aqueous phase were digested and then determined using the AAS.
2.5. Transformation of NZVI in soils
Two hundred and fifty grams of each soil were first added into a 500 mL glass bottle and 125 mg NZVI were then placed on the soil layer uniformly. Another 250 g of the same soil were added into the bottle to cover the NZVI. The glass bottles were all cultivated at 25℃. After removing the top layer of soil, soil samples were collected from NZVI layer with a plastic spoon for 1, 7, 28 days, respectively. The morphology of NZVI was tested by TEM and XRD, and the phase change of valence state transformation of NZVI was determined with XPS. The TEM and XPS methods were the same as the process refers to in section 2.2 NZVI characterization. In addition, the NZVI particles were characterized by powder X-ray diffraction (XRD, Ultima Ⅳ, Japan) using a Rigaku diffractometer and monochromatized Cu KR radiation (generator tension = 40 kV, current = 40 mA). Diffractograms were recorded from 5° to 85° (2θ) with a step size of 0.02° and a count time of 5 s per step.
2.6. Preparation of soil colloids
Soil colloids were extracted using the oscillation equilibrium method. Briefly, about 100 g of typic-hapli-udic argosols and 200 mL of DI water were mixed in a 1 L oscillation bottle and the mixture was shaken for 6 h at the speed of 150 rpm. After that, 600 mL DI water was slowly added and the bottle was stand still for 24 h. About 700 mL of supernatant were siphoned from the bottle and centrifuged at 8000 rpm for 90 min. The supernatant was poured out, and the soil colloids were collected for further experiments.
2.7. Preparation of NZVI and colloids-NZVI (C-NZVI) solutions
The NZVI solutions were prepared by adding 0.5 g of the NZVI into 50 mL of 0.1 mol/L NaCl solution and ultrasonicated for 20 min. The C-NZVI solutions were prepared by mixing 50 mL of above made NZVI solutions and 50 mL of 200 mg/L soil colloid solutions, dispersed with high-speed dispersion machine (Ultra Turrax IKA T25,German) for 30 min, and then ultrasonicated for 20 min. For the column experiments, PVP-NZVI, CTAB-NZVI and SDS-NZVI were combined with soil colloids and labeled as PVP-C-NZVI, CTAB-C-NZVI and SDS-C-NZVI, respectively. PVP-C-NZVI was selected as an example for the characterization experiments. The morphologies of the PVP-C-NZVI particles were examined using the TEM. After vacuum drying, the PVP-C-NZVI samples were crushed into powder for both XPS and XRD analyses using the methods described in section 2.2 NZVI characterization.
2.8. Colloid-facilitated transport of NZVI
Glass columns (diameter = 2.5 cm, length = 15 cm) with 20 m mesh of Teflon joints on both ends were packed with quartz sand, sandy soil, or diatomite as the porous media (Fig. S3). The wet-packing method was used to load the leaching medium into the glass tube. A peristaltic pump (BT300,China) was used to control flow through the packed column and an automatic fraction collector (BS JIAPENG, China) was employed to collect the effluent samples. At first, 10 pore volume (PV) of 0.1 mol/L NaCl solution was pumped into the column to precondition it to reach steady-state conditions. Subsequently, one pore volume of the nanoparticle suspension (PVP-NZVI, CTAB-NZVI, SDS-NZVI, PVP-C-NZVI, CTAB-C-NZVI, or SDS-C-NZVI) was applied to the column with a flow rate of 3 mL/min. Because if the rate is too fast, the adsorption amount of NZVI is small, if it is too slow, the NZVI is easy to agglomerate. At the end, the column was flushed with the 0.1 mol/L NaCl solution again to obtain the full breakthrough curve of the nanoparticles in the column. NZVI concentrations in the effluents were determined using the method described in section 2.4 adsorption kinetics of NZVI onto soils.
2.9. Statistical analyses
Statistical Analysis System (SAS) package version 9.2 (2010, SAS Institute, Cary, NC) was used to conduct all statistical analyses. Differences between the transformations of NZVI in different soil treatments were determined using the analysis of variance (ANOVA) and mean separation tests (Duncan’s multiple range test). The differences among means and correlation coefficients were considered extremely significant when P < 0.01 and significant when P < 0.05.
3. Results and discussion
3.1. Adsorption isotherms of NZVI onto soils
For all three soil samples (i.e., typic-hapli-udic argosols, typic ochri-aquic cambosols and vertic shajiang-aquic cambosols), the adsorbed amounts of NZVI increased with the initial NZVI concentrations, but the adsorption rates decreased (). When the initial concentrations of NZVI increased, the adsorbent and the NZVI had more chance to contact and collide, leading to the increased adsorption. With initial concentrations continued to increase, the adsorption sites on the surface of adsorbent kept being consumed, which would reduce the adsorption rate and eventually reach the equilibrium. The isotherms of NZVI adsorption onto the three soils were fitted well by the Langmuir isotherm model (Table S3) with the R2 values all more than 0.99. Based on the results of the Langmuir maximum adsorption capacity (qmax), the vertic shajiang-aquic cambosols showed the strongest ability to absorb NZVI, followed by typic-hapli-udic argosols and typic ochri-aquic cambosolsin a reducing order. In order to determine the influence of soil properties on NZVI adsorption, the adsorption capacity, soil texture, pH, and organic matter were analyzed by principal component analysis (Table S4). The results showed that the most related soil property with adsorption capacity was organic matter content, followed by pH, clay content, and silt content. The sand content was negatively correlated with the adsorption.
3.2. Adsorption kinetics of NZVI onto soils
The overall trends of the adsorption kinetics of NZVI onto the three soils suggest that the rate of adsorption was fast at the beginning and gradually slowed down (). The NZVI adsorption kinetics of vertic shajiang-aquic cambosols was the fastest and reached equilibrium within 8 h. The adsorption of NZVI onto typic ochri-aquic cambosolsand typic-hapli-udic argosols reached equilibrium in 24 h. For all three soils, the adsorption was more than 50% of the equilibrium adsorption amount in 2 h. The pseudo-first-order equation described the adsorption kinetics of NZVI onto the three soils very well with R2 values larger than 0.96 (Table S5).
3.3. Transformation of NZVI in soils
After day 1 during cultivation, the particle size of NZVI in the Typic-Hapli-Udic Argosols was about 50 nm (Fig. S4a, supporting information), similar to the freshly synthesized NZVI. After 7 days, the size of NZVI increased to about 80 nm and then to about 100 nm after 28 days (Fig. S4b and S4c). The results showed that the particle size of NZVI gradually increased with the increasing of incubation time. This was probably due to the aggregation of NZVI or integration of the typic-hapli-udic argosols particles to NZVI. The same result occurred in the typic ochri-aquic cambosolsand the particle size of NZVI was larger than 100 nm after 28 days (Figure S4d-f). In the vertic shajiang-aquic cambosols, the size of NZVI particles also increased, and the shape of the particles became nonuniform and irregular (Fig. S4g-i). In addition, the NZVI particles were the biggest in the vertic shajiang-aquic cambosols. Among the three soils, the vertic shajiang-aquic cambosols had the highest organic matter and clay contents, which could be combined with the NZVI particles to change their size and shape.
XRD was used to test the phase transformation of NZVI in the three soils (). After cultivation one day in the typic-hapli-udic argosols, the characteristic peaks of iron oxide appeared at 32.96° and 55.21° (). After the samples were cultivated seven days, the characteristic peaks of iron oxide at 32.96° arose ()). The characteristic peaks ()) of iron oxide at 55.159° were observed at 28 days. In typic ochri-aquic cambosols, the characteristic peaks of iron oxide appeared at 32.96°, 64.14° and 69.13° after cultivated for one day, seven days, and 28 days, respectively (–)). In vertic shajiang-aquic cambosols, the characteristic peaks of iron oxide appeared at 64.08°, 32.96° and 55.21°, when the samples were cultivated after one day, seven days and 28 days (–)). However, there were no characteristic peaks of zero valent iron observed for all the tests. These results suggest that NZVI in soils was oxidized into ferric iron quickly and lost the diffraction peak of zero-valence iron [Citation55].
Figure 3. Phase transformation of NZVI in typic-hapli-udic argosols (a: 1 day, b: 7 days, and c: 28 days); typic ochri-aquic cambosols (d: 1 day, e: 7 days, and f: 28 days); and vertic shajiang-aquic cambosols (g: 1 day, h: 7 days, and i: 28 days).
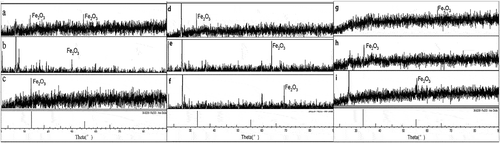
The results of XPS ((a–)) showed that, after cultivation one day in the typic-hapli-udic argosols, there were no zero-valence iron detected. There were only bivalent and trivalent iron, and its content rate were 6.52:1 ()). The bivalent and trivalent iron content rates were 4.28:1 after seven days ()) without zero-valence iron. When the samples cultured for 28 days, trivalent state iron was observed ()). It confirmed that the NZVI was oxidized quickly in soils. Similar phenomena were observed in the typic ochri-aquic cambosols and vertic shajiang-aquic cambosols ( & ).
3.4. Properties of soil colloids and C-NZVI
TEM results showed that soil colloid particles were larger, irregular shaped bar (Fig. S5a and S5c, supporting information); In C-NZVI solution, the C-NZVI was black ball shaped, and soil colloid was gray matter, which is similar with the report of others [Citation16,Citation43,Citation48]. C-NZVI was observed combining and sticking with soil colloids. TEM images confirmed that C-NZVI was the combination of colloids and NZVI and dispersed uniformly (Fig. S5b and Fig. S5d). The results suggested that natural soil colloids can be used to disperse NZVI particles in aqueous solutions.
3.5. XRD analysis of C-NZVI
XRD analysis was carried out with C-NZVI after the solution was vacuum dried. The characteristic peaks of C-NZVI binding (Figure S6a) at 45.33, 48.62 and 73.27 were characterized by Fe2O3 and Fe. This indicated that the substance contained both zero valence state iron and ferric iron, and part of NZVI was oxidized into ferric iron during the synthesis. XPS analysis of NZVI-C showed that the characteristic peaks (Fig. S6b) appeared at 710.5 and 719 eV, corresponding to zero valence iron and ferric iron, respectively. It confirmed that NZVI-C contained both zero valence and ferric iron, which is consistent with the results of XRD analysis.
3.6. Colloid-facilitated transport
Breakthrough curves of PVP-NZVI in quartz sand, sandy soil, and diatomite columns are shown in ). After the PVP-NZVI solution was pumped into the columns, all the three mediums retained some of the PVP-NZVI. With the increase of the pore volume of PVP-NZVI pumped, the iron concentrations gradually increased and then rapidly reached plateaus. After flushing with the 0.1 mol/L NaCl solutions, the iron breakthrough curves quickly returned to the background level, indicating no mobile particles in the pore water. The relative peak concentrations of PVP-NZVI transport in the quartz sand, sandy soil, and diatomite columns were 0.71 ()), 0.58 ()), and 0.19 ()), respectively. The breakthrough results showed that the PVP-NZVI had the highest mobility in quartz sand and lowest mobility in diatomite. The results indicated that the different characters such as the texture, surface charge, and the porosity of the porous media had a great impact on the transport of NZVI in porous media. In particular, diatomite is an excellent adsorbent, which is widely used in the field of water purification. Lin et al. observed that the diatomite has good adsorption properties of heavy metals in water [Citation34]. Findings from this experiment showed that diatomite might also be used to remove and treat PVP-NZVI from water.
Figure 6. Breakthrough curves of PVP-NZVI and PVP-C-NZVI in columns packed with quartz sand (A-a, A-d), sandy soil (A-b, A-e), and diatomite (A-c, A-f); Breakthrough curves of CATB -NZVI and CATB -C-NZVI in columns packed with quartz sand (B-a, B-d), sandy soil (B-b, B-e), and diatomite (B-c, B-f); Breakthrough curves of SDS-NZVI and SDS-C-NZVI in columns packed with quartz sand (C-a, C-d), sandy soil (C-b, C-e), and diatomite (C-c, C-f). C/C0 is the rate of the concentration of iron in the leaching solutions compared with the initial iron concentration.
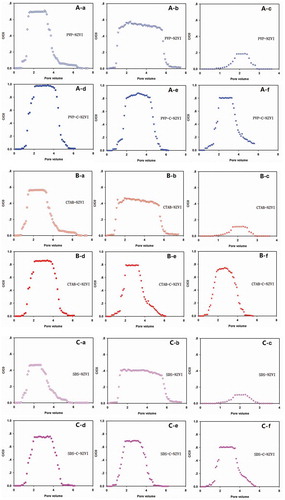
With the aid of soil colloids, PVP-C-NZVI showed high mobility in the three columns ()). The peak breakthrough concentrations of PVP-C-NZVI in quartz sand, sandy soil and diatomite column relative leaching concentration were 0.97 (-d)), 0.86 (-e)), and 0.8 (-f)), respectively. These values are much higher than that of PVP-NZVI under corresponding experimental conditions, indicating that soil colloids did facilitate the transport of NZVI in the three types of porous media. As discussed above, soil colloids and NZVI particles were integrated together, which might increase the surface charge as well as the stability of NZVI particles and thus promote their transport in porous media.
The breakthrough curves of CTAB-NZVI and SDS-NZVI in three kinds of porous media were similar to that of PVP-NZVI ( and )). The relative peak breakthrough concentrations of CTAB-NZVI in the quartz sand, sandy soil, and diatomite columns were 0.57 ()), 0.48 ()), and 0.12 ()), respectively. The relative peaking concentrations of SDS-NZVI in the quartz sand, sandy soil, and diatomite columns were slightly lower with values of 0.47 ()), 0.42 ()), and 0.1 ()), respectively. The dispersing agents (PVP, CTAB and SDS) played an important role in migration of NZVI in soil, and the PVP was the most effective dispersion agent that promoted the migration, followed by CTAB and SDS. The different soil types also affected the migration of NZVI. The mobility of all the surfactant-modified NZVI in the columns followed the order of quartz sand > sand soil > diatomite. The results also suggested that diatomite might be used as an adsorbent to remove CTAB-NZVI and SDS-NZVI from water.
Soil colloids also greatly enhanced the transport of both CTAB-NZVI and SDS-NZVI in the three columns (, )). The relative peak breakthrough concentrations of CTAB-C-NZVI in the quartz sand, sandy soil, and diatomite columns were 0.85 ()), 0.79 ()), and 0.72 ()), respectively. The relative peaking concentrations of SDS-C-NZVI in the quartz sand, sandy soil, and diatomite columns was 0.74 ()), 0.69 ()), and 0.61 ()), respectively. All these results further demonstrated that soil colloids facilitated the transport of NZVI in porous media.
The breakthrough results all showed that different dispersing agents had strong effects on the fate and transport of NZVI in porous media. Compared to the other two surfactants, PVP was the most effective dispersion agent that promoted the migration of NZVI in the columns. Further, the different medium types also showed strong effects on NZVI in the columns. The previous study showed that the relative concentration of C-NZVI in sand column was higher than that of soil columns [Citation41]. Other studies have also suggested that the texture of the medium can strongly affect the transport of colloids and nanoparticles in packed columns [Citation42,Citation43,Citation48]. On the other hand, Li et al. reported that soil minerals can provide some attachment sites to retain colloids and nanoparticles [Citation32], and Jiang et al. also observed that clay-rich medium thus should have high retention of colloids and nanoparticles [Citation52]. These are consistent with the results of this study.
Current research on soil colloids mainly concentrates on their roles in migrating of heavy metals and organic pollutants [Citation36,Citation43]. Many studies have indicated that chemical pollutants can attach to colloidal particles and be carried in hydrological pathways, known as colloid-facilitated contaminant transport [Citation43,Citation48]. As the first of its kind, this work demonstrated that soil colloids might also be able to carry engineered nanoparticles such as NZVI to enhance their mobility in porous media. The role of soil colloids was facilitators that promote the transport of NZVI in porous media. Because the NZVI will eventually enter into the soil, and the transformation, adsorption and interaction with soil colloids in the soil are seldom investigated [Citation60-Citation62]. When the NZVI enter the soil, the NZVI was easily oxidized and adsorbed (, , ). In this work, however, the soil colloids may slowdown the oxidation process, could decrease the adsorption of NZVI and make the NZVI easily migrates () because of the soil colloids could bond the NZVI (Fig. S5, supporting information). The aggregation group has common property including surface charge compared with other soil colloids and was easily migration in soil, which increased the potential hazards. This was systematic, meaningful and worth attention.
4. Conclusion
In summary, batch adsorption experimental results showed that three types of natural soils strongly adsorbed the NZVI particles with relatively fast kinetics. The Langmuir maximum adsorption capacity of NZVI onto the vertic shajiang-aquic cambosols was the highest (10.08 mg/g), followed by typic-hapli-udic argosols (8.99 mg/g) and typic ochri-aquic cambosols (6.98 mg/g). When cultivated in the three soils, the NZVI particles underwent morphological and structural transformations: the particles were quickly oxidized and their sizes increased with the culture time. Although the particles were dispersed with different dispersion agents, all the NZVI particles showed limited transport in three types of packed columns, particularly in the sandy soil and diatomite columns. The presence of soil colloids, however, greatly enhanced the transport of the NZVI particles in all the columns under the tested conditions. This suggested that soil colloids facilitated the transport of NZVI particles in porous media. As a result, when the NZVI entered into the soil after disposing wastewater or other application of NZVI, the pollution area of NZVI was rapidly expanded because of the existence of soil colloids.
Supplemental data for this article can be accessed here.
Acknowledgement
This research was funded by National Key R&D Program of China (2017YFD0200702), the National Natural Science Foundation of China (Grant No. 31572201), Shandong Province Key R&D Program (2017CXGC0306), Taishan industrial experts programme (LJNY201609), Shandong agricultural innovation team (SDAIT-17-04), Key laboratory of humic acid fertilizer of Ministry of Agriculture ([2018]1), the projects of commercialization of research findings of Shandong Province (Grant No. [2014] 183), the founds of the engineering laboratory of Shandong agricultural university & Quanlin fertilizers Inc. (QL2016-29; QL2016-34), the Great innovation projects in agriculture of Shandong Province (Grant No. [2013] 136).
Disclosure statement
No potential conflict of interest was reported by the authors.
Additional information
Funding
References
- Khalil AME, Eljamal O, Amen TWM, et al. Optimized nano-scale zero-valent iron supported on treated activated carbon for enhanced nitrate and phosphate removal from water. ChemEng J. 2017;309:349–365.
- Xie Y, Dong H, Zeng G, et al. The interactions between nanoscale zero-valent iron and microbes in the subsurface environment: a review. J Hazard Mater. 2017;321:390–407.
- Alexis A, Porubcan SX. Colloid straining within saturated heterogeneous porous media. Water Res. 2011;45:1796–1806.
- Tosco T, Papini MP, Viggi CC. Nanoscale zerovalent iron particles for groundwater remediation: a review. J Clean Prod. 2014;77:10–21.
- Bradford SA, Kim HN, Haznedaroglu BZ. Coupled factors influencing concentration-dependent colloid transport and retention in saturated porous media. Environ Sci Technol. 2009;43(18):6996–7002.
- Biruck DY, Mallavarapu M, Chen Z, et al. Environmental application and ecological significance of nano-zero valent iron. J Environ Sci. 2016;44:88–98.
- Chekli L, Bayatsarmadi B, Sekine R, et al. Analytical characterisation of nanoscale zero-valent iron: A methodological review. Anal Chim Acta. 2016;903:13–35.
- Chen J, Xiu Z, Lowry GV. Effect of natural organic matter on toxicity and reactivity of nano-scale zero-valent iron. Water Res. 2011;45(5):1995–2001.
- Chen H. Metal based nanoparticles in agricultural system: behavior, transport, and interaction with plants. Chem Spec Bioavailab. 2018;30(1):123–134.
- Diao Z, Xu Z, Jiang D, et al. Bentonite-supported nanoscale zero-valent iron/persulfate system for the simultaneous removal of Cr(VI) and phenol from aqueous solutions. ChemEng J. 2016;302:213–222.
- Dong H, Guan X, Irene MCL. Fate of As (V)-treated nano zero-valent iron: determination of arsenic desorption potential under varying environmental conditions by phosphate extraction. Water Res. 2012;46:4071–4080.
- Dallas P, Sharma VK, Zboril R. Silver polymeric nanocomposites as advanced antimicrobial agents: classification, synthetic paths, applications, and perspectives. Adv Colloid Interfac. 2011;166:119–135.
- El-Temsah YS, Joner EJ. Ecotoxicological effects on earthworms of fresh and aged nano-sized zero-valent iron (NZVI) in soil. Chemosphere. 2012;89(1):76–82.
- FrançOis M, Ana E, Nathalie L, et al. Nano-Fe° encapsulated in microcarbon spheres: synthesis, characterization, and environmental applications. ACS Appl Mater Inter. 2012;4:6235–6241.
- Boente C, Sierra C, Martínez-Blanco D, et al. Nanoscale zero-valent iron-assisted soil washing for the removal of potentially toxic elements. J Hazard Mater. 2018;350:55–65.
- Jiemvarangkul P, Zhang W, Lien HL. Enhanced transport of polyelectrolyte stabilized nanoscale zero-valent iron (nZVI) in porous media. Chem Eng J. 2011;170:482–491.
- Jośko I, Oleszczuk P. Manufactured nanomaterials: the connection between environmental fate and toxicity. Crit Rev Environ Sci Technol. 2013;43:2581–2616.
- Praetorius A, Tufenkji N, Goss KU, et al. The road to nowhere: equilibrium partition coefficients for nanoparticles. Environ Sci-Nano. 2014;1:317–323.
- Dale AL, Lowry GV, Casman E. Much ado about a: reframing the debate over appropriate fate descriptors in nanoparticle environmental risk modeling. Environ Sci-Nano. 2015;2:27–32.
- Cornelis G. Fate descriptors for engineered nanoparticles: the good, the bad, and the ugly. Environ Sci-Nano. 2015;2:19–26.
- Vítková M, Rákosová S, Michálková Z, et al. Metal(loid)s behaviour in soils amended with nano zero-valent iron as a function of pH and time. J Environ Manage. 2017;186:268–276.
- Kaegi R, Voegelin A, Ort C. Fate and transformation of silver nanoparticles in urban wastewater systems. Water Res. 2013;47:3866–3877.
- Xie B, Jiang Y, Zhang Z, et al. Co-transport of Pb (II) and Cd (II) in saturated porous media: effects of colloids, flow rate and grain size. Chem Speciation Bioavailability. 2018;30(1):135–143.
- Xue W, Huang D, Zeng G, et al. Nanoscale zero-valent iron coated with rhamnolipid as an effectivestabilizer for immobilization of Cd and Pb in river sediments. J Hazard Mater. 2018;341:381–389.
- Li Z, Wang L, Meng J, et al. Zeolite-supported nanoscale zero-valent iron: new findings onsimultaneous adsorption of Cd(II), Pb(II), and As(III) in aqueoussolution and soil. J Hazard Mater. 2018;344:1–11.
- Kim JH, Lee Y, Kim EJ. Exposure of iron nanoparticles to Arabidopsis thaliana enhances root elongation by triggering cell wall loosening. Environ Sci Technol. 2014;48:3477–3485.
- Liu F, Shan C, Zhang X, et al. Enhanced removal of EDTA-chelated Cu(II) by polymeric anion-exchanger supported nanoscale zero-valent iron. J Hazard Mater. 2017;321:290–298.
- Liang Z, Wen Q, Wang X, et al. Chemically stable and reusable nano zero-valent iron/graphite-like carbon nitride nanohybrid for efficient photocatalytic treatment of Cr(VI) and rhodamine B under visible light. Appl Surf Sci. 2016;386:451–459.
- Liang B, Xie Y, Fang Z. Assessment of the transport of polyvinylpyrrolidone-stabilised zero-valent iron nanoparticles in a silica sand medium. J Nanopart Res. 2014;16:1–11.
- Huang D, Hu Z, Peng Z, et al. Cadmium immobilization in river sediment using stabilized nanoscale zero-valent iron with enhanced transport by polysaccharide coating. J Environ Manage. 2018;210:191–200.
- Li X, Yang Y, Gao B, et al. Stimulation of peanut seedling development and growth by zero-valent iron nanoparticles at low concentrations. Plos One. 2015;10:1–12.
- Li Z, Dong H, Zhang Y, et al. Enhanced removal of Ni(II) by nanoscale zero valent iron supported on Na-saturated bentonite. J Colloid Interfac Sci. 2017;497:43–49.
- Li Y, Cheng W, Sheng G, et al. Synergetic effect of a pillared bentonite support on SE(VI) removal by nanoscale zero valent iron. Appl Cata B-Environ. 2015;174:329–335.
- Ling L, Pan B, Zhang W. Removal of selenium from water with nanoscale zero-valent iron: mechanisms of intraparticle reduction of Se(IV). Water Res. 2015;71:274–281.
- Ma X, Gurung A, Deng Y. Phytotoxicity and uptake of nanoscale zero-valent iron (nZVI) by two plant species. Sci Total Environ. 2013;443:844–849.
- Magee BR, Lion LW, Lemley AT. Transport of dissolved organic macromolecules and their effect on the transport of phenanthrene in porous media. Environ Sci Technol. 1991;25:323–331.
- Moralesa VL, Zhang W, Gao B. Impact of dissolved organic matter on colloid transport in the vadose zone: deterministic approximation of transport deposition coefficients from polymeric coating characteristics. Water Res. 2011;45:1691–1701.
- Nel A, Xia T, Mädler L. Toxic potential of materials at the nanolevel. Sci. 2006;311:622–627.
- Park HJ, Kim HY, Cha S. Removal characteristics of engineered nanoparticles by activated sludge. Chemosphere. 2013;92:524–528.
- Petersen EJ, Zhang L, Mattison NT. Potential release pathways, environmental fate, and ecological risks of carbon nanotubes. Environ Sci Technol. 2011;45:9837–9856.
- Porubcan AA, Xu S. Colloid straining within saturated heterogeneous porous media. Water Res. 2011;45:1796–1806.
- Raychoudhury T, Tufenkji N, Ghoshal S. Aggregation and deposition kinetics of carboxymethyl cellulose-modified zero-valent iron nanoparticles in porous media. Water Res. 2012;46:1735–1744.
- Roy SB, Dzombak DA. Chemical factors influencing colloid-facilitated transport of contaminants in porous media. Environ Sci Technol. 1997;31:656–664.
- Lin Y, Tseng H, Wey M, et al. Characteristics of two types of stabilized nano zero-valent iron and transport in porous media. Sci Total Environ. 2010;408:2260–2267.
- Sun Y, Lei C, Eakalak K, et al. Nanoscale zero-valent iron for metal/metalloid removal from model hydraulic fracturing wastewater. Chemosphere. 2017;176:315–323.
- Dong L, Lin L, Li Q, et al. Enhanced nitrate-nitrogen removal by modified attapulgite-supported nanoscale zero-valent iron treating simulated groundwater. J Environ Manage. 2018;213:151–158.
- Saccà ML, Fajardo C, Nande M. Effects of nano zero-valent iron on Klebsiella oxytoca and stress response. Microb Ecol. 2013;66:806–812.
- Sen TK, Khilar KC. Review on subsurface colloids and colloid-associated contaminant transport in saturated porous media. Adv Colloid Interfac Sci. 2006;119:71–96.
- Mines PD, Uthuppu B, Thirion D, et al. Granular activated carbon with grafted nanoporous polymer enhances nanoscale zero-valent iron impregnation and water contaminant removal. Chem Eng J. 2018;339:22–31.
- Sun YP, Li XQ, Cao J. Characterization of zero-valent iron nanoparticles. Adv Colloid Interfac Sci. 2006;120:47–56.
- Tang SCN, Lo IMC. Magnetic nanoparticles: essential factors for sustainable environmental applications. Water Res. 2013;47:2613–2632.
- Jiang Y, Yu L, Sun H, et al. Transport of natural soil nanoparticles in saturated porous media: effects of pH and ionic strength. Chem Speciation Bioavailability. 2017;29(1):186–196.
- Harifi-Mood AR, Hadavand-Mirzaie F. Adsorption of Basic violet 16 from aqueous solutions by waste sugar beet pulp: kinetic, thermodynamic, and equilibrium isotherm studies. Chem Spec Bioavailab. 2015;27(1):8–14.
- Tian YA, Gao B, Silvera-Batista C, et al. Transport of engineered nanoparticles in saturated porous media. J Nanopart Res. 2010;12:2371–2380.
- Liu A, Liu J, Han J, et al. Evolution of nanoscale zero-valent iron (nZVI) in water: microscopic and spectroscopic evidence on the formation of nano- and micro-structured iron oxides. J Hazard Mater. 2017;322:129–135.
- Ko I, Kim J, Kim K. Adsorption properties of soil humic and fulvic acids by hematite. Chem Spec Bioavailab. 2005;17(2):41–48.
- Wang Q, Snyder S, Kim J. Aqueous ethanol modified nanoscale zerovalent iron in bromate reduction: synthesis, characterization, and reactivity. Environ Sci Technol. 2009;43:3292–3299.
- Wang X, Liu P, Fu M, et al. Novel sequential process for enhanced dye synergistic degradation based on nano zero-valent iron and potassium permanganate. Chemosphere. 2016;155:39–47.
- Xing M, Wang J. Nanoscaled zero valent iron/graphene composite as an efficient adsorbent for Co(II) removal from aqueous solution. J Colloid Sci. 2016;474:119–128.
- Xi Y, Mallavarapu M, Naidu R. Reduction and adsorption of Pb2+ in aqueous solution by nano-zero-valent iron—a SEM, TEM and XPS study. Mater Res Bull. 2010;45:1361–1367.
- Yan W, Lien HL, Koel BE. Iron nanoparticles for environmental clean-up: recent developments and future outlook. Environ Sci: Proce Impact. 2013;15:63–77.
- Zhang M, He F, Zhao DY. Degradation of soil-sorbed trichloroethylene by stabilized zero valent iron nanoparticles: effects of sorption, surfactants, and natural organic matter. Water Res. 2011;45:2401–2414.