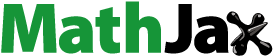
ABSTRACT
In this work, the optimum conditions for the synthesis of nano zero valent iron sulfide were studied in detail using orthogonal experiment. The palygorskite-supported sulfide-modified nanoscale zero-valent iron (S-nZVI/P) was prepared, characterized, and further applied to remove Congo red (CR) in aqueous solutions. The results of structural characterization showed that the Fe0 was coated with FeS layers. The influence of sulfidation conditions on the removal rat e of CR was investigated in detail, and the results revealed that S-nZVI/P prepared at 30°C with a Fe/S ratio of 1 was found to effectively remove CR. Moreover, S-nZVI/P showed a significantly enhanced removal rate towards CR as compared with nZVI/P and nZVI, suggesting that sulfidation treatment is a promising method to improve the reactivity of nZVI.
1. Introduction
Since the early 1990s, zero valent iron (ZVI) has been used as a promising reactant for the removal/degradation of pollutants in contaminated groundwater [Citation1]. Nano zero-valent iron (nZVI) technology has received increasing attention in groundwater treatment due to its remarkable activity as compared to ZVI, because nZVI has special properties such as small particle size, large specific surface area and macroscopic quantum tunneling effect [Citation2–Citation4]. Increasing research has suggested that nZVI can significantly improve the percentage of surface atoms (> 50%), reaction activity and reaction rate during the pollutant treatment process [Citation5–Citation7]. However, nZVI has poor stability, easily loses its activity, and is easy to oxidize or even spontaneously combust in air, which requires harsh operating conditions [Citation8]. In the in-situ remediation of the soil/groundwater pollution environment, the nZVI is easy to aggregate due to the magnetic properties, which can decrease the surface-active sites. Besides, the metallic iron is rapidly oxidized when exposed to air, leading to rapid inactivation. In addition, nano zero valent iron (nZVI) is hydrophilic, which limits its reaction with hydrophobic organic pollutants. Hence, the ability to control the agglomeration and oxidation of nZVI and improve its hydrophilicity is the key to enhancing the performance of nZVI.
Methods such as supporting on substrate, coating on stabilizer, doping with metal catalyst (Ni, Pd or Cu), or surface modification are developed to solve the above problems [Citation3,Citation9–Citation12]. Among all the strategies, supporting nZVI on inorganic clay mineral has aroused most research interest [Citation12–Citation14]. Clay is abundant in the earth and is a suitable substrate for most pollutants. Clay can not only prevent the agglomeration of nZVI, thus increasing the total surface area of the contact between nanoparticles and pollutants [Citation11], but can also promote the accumulation of pollutants on its surface due to the strong interaction. For example, Soliemanzadeh et al. found that the maximum sorption capacities of bentonite-supported nZVI were much higher (27.63 mg/g) than those of natural bentonite (4.61 mg/g) [Citation13]. The decolorization efficiencies of MO were greatly enhanced after suppoting nZVI on the Hangjin 2# clay [Citation14]. However, nZVI particles that are supported on the clay are still exposed to the environment, which can be passivated by an iron oxide or hydroxide film produced from the corrosion process, resulting in a reduced activity.
Recently, many studies have revealed that surface sulfidation can control the corrosion and improve the conductivity of nZVI [Citation15–Citation17]. Because FeS has a higher electronegativity (5.02 V) than nZVI (4.04 V), the electrons generated from the corrosion process of nZVI can transfer to FeS. Moreover, FeS has a good electron conductivity and strong reductivity, which can improve the activity of nZVI. Kim et al. found that the reduction reactivity of nZVI toward TCE could be enhanced after coating FeS due to more efficient electron transfer [Citation18]. The sulfide of nZVI can be achieved by adding dithionite in the reducing process of ferric chloride by sodium borohydride. Han et al. and Gu et al. reported that Fe/FeS could be formed through the addition of dithionite during borohydride reduction of ferric chloride, and Fe/FeS showed an enhanced degradation rate towards trichloroethene (TCE) [Citation16,Citation19]. S-nZVI, obtained from a similar process, was also effective in treating Cd, and the removal capacity could be up to 85 mg/g [Citation20]. The S-nZVI also showed significantly increased degradation activity to organic dyes. Orange I removal kobs rate of S-nZVI is 71.8 times higher than the one without sulfidation [Citation21]. Many results showed that sulfidation conditions had great effects on target pollutants degradation efficiency [Citation15,Citation17,Citation19,Citation20]. For example, Cao et al. found that the removal of antibiotic florfenicol was strongly dependent on S/Fe and the surface-area-normalized reaction rate constant of antibiotic florfenicol on S-nZVI at the optimal S/Fe molar ratio (0.14) was 48 times higher than nZVI [Citation22]. Rajajayavel and Ghoshal reported that the highest TCE dechlorination rates could be achieved at Fe/S ratios ranging from 12–25, and rates decreased at both higher and lower Fe/S ratios [Citation23]. Accordingly, how the Fe/S ratio affects pollutant degradation and its mechanism remains to be studied. In our previous work, we found that the drop acceleration of potassium borohydride solution would affect the particle size and agglomeration of nZVI. Hwang et al. also found that the nZVI synthesis conditions such as reductant delivery rate, reaction time and nuclei concentration affected the nZVI particle size and agglomeration [Citation24]. Temperature is an important parameter affecting the synthesis process because it affects the speed of the molecules’ diffusion in solution. So, sulfidation conditions, such as temperature and time, should also affect the size and structure of S-nZVI, resulting in changes in performance.
To take advantage of clay supports, nZVI and surface sulfidation technology, a sulfurized nZVI supported on palygorskite clay (S-nZVI/P) was well prepared and used to degrade the Congo red. The effects of sulfidation time, sulfidation temperature and Fe/S on S-nZVI/P, its properties and reactivity were determined. Furthermore, the synergetic effects associated with clay supports and surface sulfidation were systematically analyzed.
2. Materials and methods
2.1. Chemicals
The reagents including Congo red (CR, ≥ 97.0%), potassium borohydride (KBH4, ≥ 99.9%), ferrous chloride tetrahydrate (FeCl2 · 4H2O, 99%) and sodium sulfite (Na2SO3, ≥98.0%) were of analytical grade and purchased from Sigma-Aldrich. Sodium hydroxide (≥99.5%), absolute ethanol (C2H5OH) and hydrochloric acid (HCl, 37.5%) were procured from Sino-pharm Chemical Reagent Company (Shanghai, China) and used as received. Jiangsu Junda Material Co., Ltd offered palygorskite clay for use in this study. All double-distilled water used in the experiment was deoxygenated by purging with N2 for 60 min.
2.2. Synthesis of S-nZVI/P
The synthesis of S-NZVI/P was proceeded by the following steps according to Quan et.al [Citation12]. Firstly, the acid-activated palygorskite was equilibrated with Fe2+ by ion exchange and centrifugal separation. Then, the Fe2+ species on the palygorskite clay were reduced to Fe0 using KBH4 and Na2SO3 (8g/L) together through the liquid-phase reduction method to S-nZVI/P. The entire process of the synthesis is N2-protected. The samples were washed with absolute ethanol instead of water to avoid the immediate oxidation of S-nZVI/P, and the obtained composites were put in an anaerobic tank. The resulting reaction can be expressed as:
Herein, such sulfidation conditions as temperature, reaction time, and Fe2+/S were deeply investigated. The process parameters and their selected levels are shown in . For three parameters at three levels each, the usual full factorial program would require 33 or 27 experiments. However, only nine experiments are needed in the Taguchi L9 orthogonal design [Citation25,Citation26]. The experimental layout for the S-nZVI/P synthesis process parameters, using the L9 orthogonal array, is shown in .
Table 1. Experimental variables and the levels of their settings.
Table 2. Experimental design and yields obtained.
2.3. Characterization of S-nZVI/P
X-ray photoelectron spectroscopy (XPS) experiment was carried out on a RBD upagraded Thermo ESCALAB 250 system with an Al Kα X-ray having asource power of 150W. The specific surface area of the samples was determined with an ASAP 2020 (Micromeritics, USA) at 77K. A Hitachi S4800 scanning electron microscope (SEM) was equipped with an EX-250 energy spectrum analysis system to calibrate the charge of the sample and collect the binding energy map of C1S, O1S and other elements on the sample surface for analysis. All samples should be attached to carbon film.
2.4. Batch experiment
All the batch experiments in aqueous solutions were implemented in 500 mL glass bottles. For S-nZVI/P reduction decolorization, S-nZVI/P (1.6 g/L) was compounded with 250 mL 20 mg/L of CR solutions. Then, it was immediately sealed and vapor-bathed at a constant temperature of 25 ± 0.5°C and vibrated at a speed of 200 rpm for 180 min. At defined time intervals, 5.0 mL of the solution was withdrawn and filtrated for further analysis. The analysis method referenced our former research. All the samples were tested using a T60 UV-VIS Spectrophotometer (Beijing Purkinje General Instrument Co. Ltd) at 488 nm of maximum absorbance wavelength [Citation27]. The nZVI/P removal experiment is a comparison and all the experiments are the same as S-nZVI/P.
3. Results and discussion
3.1. Optimum synthesis condition
The experimental layout for the S-nZVI/P synthesis process parameters, using the L9 orthogonal array and the removal rate of the CR, is shown in . Obviously, the sulfidation conditions have a great effect on the removal rate of CR. The highest removal rate was 99.62%, while the lowest was 49.62%. The mean of experimental at different levels of the reaction parameter for temperature, time and ratio Fe/S is summarized in . Fe/S has the greatest influence on the removal rate, and the reaction time has a little influence. According to the synthetic temperature analysis, the 30°C CR removal effect is the worst at 20°C and 40°C when the difference is not obvious. The optimal reaction conditions for the synthesis of S-nZVI/P are C1A1B3, i.e. the optimum synthesis conditions for Fe/S-ratio 1:1, reaction temperature 20°C, reaction time 3 h.
Table 3. Range analysis of the orthogonal synthesis experiment.
Experimental data were also analyzed using design-expert 8.0.7.1 software, and the results are shown in . It instanced some configuration for the quadratic response surface plot in the optimization of two variables. The surface shown in ) recommended a minimum point in the visual inspection of the surfaces. The surface shown in ,) presented the saddle point; it could usually be described as the critical point. The saddle point is an inflexion point between a relative maximum and a relative minimum point [Citation28]. There was no maximum point in , which meant that optimal optimization results could not be obtained by using the quadratic response surface plot from orthogonal experiments, on account of three-level factorial designs being infrequent and its inefficiency for higher numbers of variables. The interaction of factors on the CR removal rate was also affected by the following factors: C > A > B.
3.2. Characterization of S-nZVI/P
In order to analyze the pore structure and specific surface area of S-nZVI/P, N2 sorption was conducted. As seen in , the N2 adsorption/desorption isotherm of S-nZVI/P exhibits a typical type-IV isotherm with an obvious hysteresis loop at a wide relative pressure range (0.4–1.0), indicating that numerous mesopores and macropores exist in the S-nZVI/P structure. Moreover, the special surface area of S-nZVI/P is 115.01 m2/g, which is much larger than that of nZVI/P (25.63 m2/g). After surface sulfidation, the more dispersed Fe sulfide phases covered the surface of the clay substrate, resulting in an increased specific surface area. With a larger specific surface area, S-nZVI/P can provide more adsorption sites for target pollutant accumulation. As seen from the pore size distribution curve, the mesopore size of S-nZVI/P is distributed at about 37.5 nm. These results indicate that surface sulfidation can increase the specific surface area and promote the formation of a porous structure, which is beneficial to target the transport and adsorption of pollutants.
The surface chemical composition of S-nZVI/P was analyzed with X-ray photoelectron spectroscopy (XPS) and the result is shown in . Significant Fe2p and weak S2p binding energy peaks were found on the surface of palygorskite. In the S (2p) spectrum of S-nZVI/P, a broad single peak was fitted with doublets at 162.7 ± 0.5 and 164.38 ± 0.4 eV in ), which typically corresponded to sulfide (S2-) and polysulfide (Sn2-) species. The polysulfide species disclosed that some oxidation of the surface S2- might have happened [Citation29,Citation30]. As calculated, the contents of S2- and Sn2- are 37.5% and 36.42%, respectively, which is consistent with previous studies [Citation19,Citation22].
Due to the surface sulfidation, Fe0 would be oxidized into different valence states, and the content of Fe0 will affect the reduction efficiency of the target pollutant. As seen from the high-resolution XPS spectrum of the Fe 2p region, the peaks located at 707 eV and 719 eV correspond to Fe0 2p3/2 and Fe0 2p1/2, indicating the existence of Fe0 in the FeS-coated Fe0 structure. The broad peaks of Fe 2p1/2 at 723 eV account for the oxidized iron species (Fe2+), suggesting that Fe0 was oxidized in the sulfidation process, and iron oxide or Fe-S compounds were formed.
SEMs were performed to reveal the detailed morphology of S-nZVI/P, and the images are shown in . After supporting S-nZVI particles, the clay shows a rough surface. Moreover, the S-nZVI particles are uniformly dispersed on the surface of the matrix without obvious agglomeration. The EDS spectrum ()) of S-nZVI/P also confirms that the clay substrate was well coated with S-nZVI. As seen from the EDS mapping, the Fe and S element are highly dispersed on the surface of the clay, so nanoparticles was wrapped by some ferrous sulfide. Iron sulfides are commonly understood as either semiconductors or metallic conductors because of the presence of delocalized electrons in the layers [Citation18]. Hence, the FeS-Fe composites are deposited on the surface of the clay substrate, which can promote electron transfer and mitigate the corrosion of nZVI.
3.3. Kinetics of CR removal
To investigate the reduction performance of the obtained S-nZVI/P, CR was chosen as a target contaminant. shows the profile of CR removal by S-nZVI/P, nZVI/P and nZVI at an initial concentration of 20 mg/L. Obviously, CR was rapidly removed by S-nZVI/P within the first 1h, and then removed slowly in the following two hours. According to the previous reports, the removal of Congo red is a multi-step process including redox reactions and adsorption [Citation2,Citation27]. After 3 h, the CR removal rates of S-nZVI/P, nZVI/P and nZVI were 99.62%, 77.3% and 56.29%, respectively. The higher removal rate of S-nZVI/P can be ascribed to the following aspects: Firstly, the clay supports prevented the agglomeration of nanoparticles, and enhanced the activity of S-nZVI. Secondly, FeSx covered the nZVI surface, which can further mediate the aggregation of nZVI and increase the specific surface area. Thirdly, sulfur in S-nZVI can inhibit hydrogen recombination and thus atomic hydrogen reduction can be enhanced, which implies that the reactivity of S-nZVI on the surface is towards CR rather than H2O. The interior Fe-S bonds was broken by dissolved oxygen under oxic conditions which modified them to sulfhydryl groups for object contaminant adsorption and led to an increase removal capacity [Citation31,Citation32].
Figure 5. Kinetics of S-nZVI/P, nZVI/P and nZVI removal CR (dosage 1.6 g/L, C0 = 20 mg/L, room temperature).
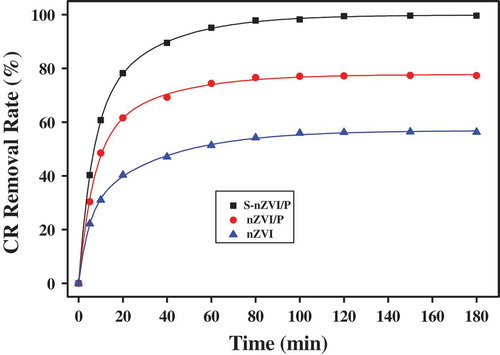
In order to better describe the kinetics of removing Congo red by S-nZVI/P,nZVI/P and nZVI, the experimental data are simulated in . The reaction kinetics processes of S-nZVI/P and nZVI/P are described as equations 4, 5 and 6:
where y is the removal rate (%) and x is the reaction time. The two-phase exponential decay function could be described with time constant parameters. The fitting results are shown in . The R-square was greater than 0.99, indicating that the fitting result of S-nZVI/P was better than that of nZVI/P and nZVI. The fit statuses were all succeeded.
Table 4. Fitting results of S-nZVI/P, nZVI/P and nZVI removing CR.
4. Conclusion
In this study, we prepared S-nZVI/P by treating nZVI/P and nZVI with sodium dithionite for CR degradation from aqueous solutions. The effects of Fe/S, temperature and sulfidate time on the CR degradation were investigated using orthogonal experiment. The degradation rate achieved the maximum value at an Fe/S ratio of 1 at 30°C for 3 h. Both the SEM and XPS analyses showed that the sulfur species was fully loaded onto nZVI/P particles, and the nZVI/P was coated with FeS layers in the resultant material. In the degradation experiments, S-nZVI/P showed an enhanced removal rate towards CR as compared to nZVI/P and nZVI. CR removal by S-nZVI/P agreed with the kinetics model. The removal mechanism entails chemical adsorption of CR onto S-nZVI/P, and atomic hydrogen reduction. The excellent degradation performance of S-nZVI/P makes it a promising material for the removal of pollutants in aqueous solutions.
Disclosure statement
No potential conflict of interest was reported by the authors.
Additional information
Funding
References
- Gillham RW, O’Hannesin SF. Enhanced degradation of halogenated aliphatics by zero‐valent iron. Ground Water. 1994;32:10.
- Yang Hsin S, Chih Ping T. Fast decolorization of azo-dye Congo red with zerovalent iron nanoparticles and sequential mineralization with a fenton reaction. Environ Eng Sci. 2010;29:929–933.
- O’Carroll D, Sleep B, Krol M, et al. Nanoscale zero valent iron and bimetallic particles for contaminated site remediation. Adv Water Res. 2013;51:104–122.
- Crane RA, Scott TB. Nanoscale zero-valent iron: future prospects for an emerging water treatment technology. J Hazard Mater. 2012;211-212:112–125.
- Kang S, Liu S, Wang H, et al. Enhanced degradation performances of plate-like micro/nanostructured zero valent iron to DDT. J Hazard Mater. 2016;307:145–153.
- Cao J, Xiong Z, Lai B. Effect of initial pH on the tetracycline (TC) removal by zero-valent iron: adsorption, oxidation and reduction. Chem Eng J. 2018;343:492–499.
- Zhang SH, Wu MF, Tang TT, et al. Mechanism investigation of anoxic Cr(VI) removal by nano zero-valent iron based on XPS analysis in time scale. Chem Eng J. 2018;335:945–953.
- Ling L, Zhang WX. Sequestration of arsenate in zero-valent iron nanoparticles: visualization of intraparticle reactions at angstrom resolution. Environ Sci Technol Lett. 2014;1:305–309.
- You W, Weng Y, Wang X, et al. Synthesis and adsorption properties of hierarchically ordered nanostructures derived from porous CaO network. ACS Appl Mater Interfaces. 2016;8:33656–33665.
- Liu T, Zhao L, Sun D, et al. Entrapment of nanoscale zero-valent iron in chitosan beads for hexavalent chromium removal from wastewater. J Hazard Mater. 2010;184:724–730.
- Quan G, Sun W, Yan J, et al. Nanoscale zero-valent iron supported on biochar: characterization and reactivity for degradation of acid Orange 7 from aqueous solution. Water Air Soil Pollut. 2014;225:2195–2204.
- Quan G, Zhang J, Guo J, et al. Removal of Cr(VI) from aqueous solution by nanoscale zero-valent iron grafted on acid-activated attapulgite. Water Air Soil Pollut. 2014;225:1979–1989.
- Akbar S, Majid F. Synthesis of clay-supported nanoscale zero-valent iron using green tea extract for the removal of phosphorus from aqueous solutions. Chin J Chem Eng. 2017;25:924–930.
- Li X, Zhao Y, Xi B, et al. Decolorization of methyl orange by a new clay-supported nanoscale zero-valent iron: synergetic effect, efficiency optimization and mechanism. J Environ Sci (China). 2017;52:8–17.
- Fan D, O’Brien Johnson G, Tratnyek PG, et al. Sulfidation of nano zerovalent iron (nZVI) for improved selectivity during in-situ chemical reduction (ISCR). Environ Sci Technol. 2016;50:9558–9565.
- Han Y, Yan W. Reductive dechlorination of trichloroethene by zero-valent iron nanoparticles: reactivity enhancement through sulfidation treatment. Environ Sci Technol. 2016;50:12992–13001.
- Fan D, Lan Y, Tratnyek PG, et al. Sulfidation of iron-based materials: a review of processes and implications for water treatment and remediation. Environ Sci Technol. 2017;51:13070–13085.
- Kim EJ, Kim JH, Azad AM, et al. Facile synthesis and characterization of Fe/FeS nanoparticles for eEnvironmental applications. ACS Appl Mater Interfaces. 2011;3:1457–1462.
- Gu Y, Wang B, He F, et al. Mechanochemically sulfidated microscale zero valent iron: pathways, kinetics, mechanism, and efficiency of trichloroethylene dechlorination. Environ Sci Technol. 2017;51:12653–12662.
- Su Y, Adeleye AS, Keller AA, et al. Magnetic sulfide-modified nanoscale zerovalent iron (S-nZVI) for dissolved metal ion removal. Water Res. 2015;74:47–57.
- Xu C, Zhang B, Wang Y, et al. Effects of sulfidation, magnetization, and oxygenation on azo dye reduction by zerovalent iron. Environ Sci Technol. 2016;50:11879–11887.
- Cao Z, Liu X, Xu J, et al. Removal of antibiotic florfenicol by sulfide-modified nanoscale zero-valent iron. Environ Sci Technol. 2017;51:11269–11277.
- Rajajayavel SR, Ghoshal S. Enhanced reductive dechlorination of trichloroethylene by sulfidated nanoscale zerovalent iron. Water Res. 2015;78:144–153.
- Hwang YH, Kim DG, Shin HS. Effects of synthesis conditions on the characteristics and reactivity of nano scale zero valent iron. Appl Catal, B. 2011;105:144–150.
- Carlson R, Simonsen G, Descomps A. Orthogonal experiments in the development of organic synthetic processes. Org Process Res Dev. 2009;13:798–803.
- Sharma P, Verma A, Sidhu RK, et al. Process parameter selection for strontium ferrite sintered magnets using Taguchi L9 orthogonal design. J Mater Process Technol. 2005;168:147–151.
- Quan G, Zhao H, Yan J. Degradation of Congo red by integration of supported nanoscale zero-valent iron with photo-catalytic oxidation. Desalin Water Treat. 2017;82:114–120.
- Bezerra MA, Santelli RE, Oliveira EP, et al. Response surface methodology (RSM) as a tool for optimization in analytical chemistry. Talanta. 2008;76:965–977.
- Boursiquot S, Mullet M, Abdelmoula M, et al. The dry oxidation of tetragonal FeS1-xmackinawite. Phys Chem Miner. 2001;28:600–611.
- Mullet M, Boursiquot S, Abdelmoula M, et al. Surface chemistry and structural properties of mackinawite prepared by reaction of sulfide ions with metallic iron. Geochim Cosmochim Acta. 2002;66:829–836.
- Wu D, Peng S, Yan K, et al. Enhanced As(III) sequestration using sulfide-modified nano-scale zero-valent iron with a characteristic core–shell structure: sulfidation and as distribution. ACS Sustain Chem Eng. 2018;6:3039–3048.
- Dong H, Zhang C, Deng J, et al. Factors influencing degradation of trichloroethylene by sulfide-modified nanoscale zero-valent iron in aqueous solution. Water Res. 2018;135:1–10.