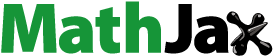
ABSTRACT
It is very important to develop effective and reusable functional materials for arsenic removal from water. In this study, one carbon fiber nanocomposite mat modified with ceria oxide nanoparticles was successfully prepared by electrospinning. The adsorption performances of the composite for different arsenic species were systematically investigated. The composite possessed good performance for selective adsorption of AsⅤ at pH 12 without obvious coexisting ion interference effects. The adsorption processes fitted well with the quasi-first-order dynamics and Langmuir isotherm models. The composite mat is stable and reusable, and can be easily isolated from water after use. The prepared composites were characterized by scanning electron microscope and energy-dispersive spectroscope (SEM-EDS), x-ray diffraction spectrometry (XRD) and Fourier transform infrared spectrum (FTIR), and the possible adsorption mechanism was also discussed.
1. Introduction
Arsenic (As) is one of the most crucial pollutants with strong carcinogenicity [Citation1], and it can be mainly present in the species of arsenite (AsIII) and arsenate (AsV) in water [Citation2–4]. Both the health effects and environmental fate of arsenic depend not merely on its total concentration, but on its species [Citation5,Citation6]. Compared with arsenate, arsenite is more nocuous and harder to be removed from water [Citation7]. Adsorption process has been widely applied for arsenic pollution control in water because of its high efficiency, flexibility and simplicity [Citation8]. The previous study showed that cerium oxide performed good adsorption properties for trivalent arsenic [Citation9]. However, there are still some problems to be solved for the adsorbents. For example, AsIII is normally to be oxidized into AsV before adsorption in order to achieve satisfactory efficiency [Citation10]. In addition, the powdered adsorbents are always very difficult to be collected after use. In order to enhance the selectivity and capacity, the adsorbents are normally modified with nanoparticles [Citation11]. However, the nanoparticles on the surface of the composite loaded by conventional chemical methods are generally unstable [Citation12]. When the composites are blended in water, the loaded nanoparticles are very easy to drop off and pose secondary pollution risks [Citation13]. In consequence, developing a safe, stable, efficient and selective arsenic adsorption material is significant and urgent.
Due to their higher surface area and porosity, carbon nanofibers are not only an excellent adsorbent, but also an ideal skeleton for nanoparticle modification [Citation14,Citation15]. Electrospinning technology can continuously produce nano-sized metal-organic polymer fibers [Citation16]. At present, there are three main routes to prepare metal oxides modified nanofibers by electrospinning: The first route is blending metal oxides. Nanometer cerium oxide is adopted by means of sol-gel, and then blended via electrospinning [Citation17,Citation18]. Although the composite fibers containing CeO2 nanoparticles can be prepared efficiently via this method, the loaded composites usually have a tight coating on the nanoparticles, and the nanoparticles covering the fiber surface cannot be completely exposed [Citation19]. The second route is impregnation-calcination. The nanofibers are impregnated in the relevant precursor solutions, and then the fibers are thermally treated to load the corresponding oxides. This method has the advantages of simple operation and large loading amounts, but the cerium oxide nanoparticles are mostly allocated on the fiber superficies and not firmly bound to the carbon nanofibers [Citation20]. Therefore, the loaded cerium oxide nanoparticles are easy to fall off into the solution when it is used, resulting in secondary pollution [Citation21,Citation22]. The third route is blending-calcination. Cerium salt solution and spinning precursor are blended, and the composite nanofibers are calcined at high temperature to obtain CeO2 modified carbon nanofibers. The cerium oxide nanoparticles formed by this method can be uniformly embedded in the surface and interior of the fibers [Citation23]. Cerium nitrate can be decomposed and oxidized into cerium oxide at high temperature [Citation24]. Decomposition of nitrate at high temperature can produce N2 gas, and fabricate holes and channels in the fibers, increasing the relatively special surface area and the reactive sites of carbon nanofibers [Citation23]. Nevertheless, the addition of cerium salt will change the viscosity and conductivity of the precursor polymer solution, which will increase the difficulty of electrospinning. Hence, it is significant to develop a new method for the synthesis of CeO2 nanofiber composites by electrospinning.
In this paper, ceria nanofiber composite mat with good performance and stability was successfully prepared by electrospinning. The as-prepared composite was successfully used to remove arsenic in water. During this process, polyacrylonitrile (PAN) was used as precursor polymer and underwent calcination treatment. The nanoparticles were embedded and uniformly distributed in the composite nanofibers. SEM-EDS, XRD and FRIR were used for characterization and to explore the adsorption mechanism. The objectives of this work were (1) to fabricate a stable, reusable and effective nanocomposite by electrospinning for selective adsorption arsenic species in water, and (2) to explore the adsorption performances of arsenic species (AsIII and AsV) on the composite.
2. Experimental
2.1. Materials and instrument
Polyacrylonitrile (PAN) (Bellingway Technology Junior K, Beijing); Nmeo N-dimethylformamide (DMF) (McLean Biochemical Technology Co. Ltd, Shanghai); Cerium nitrate hexahydrate (Komeo Chemical Reagent Co. Ltd., Tianjin); Arsenic standard storage solution (NRCCRM, China).
Electrospinning instrument (Yongkangleye Technology Development Co. Ltd., Beijing); NBD-1200 tube furnace (Henan Nuobadi Technology Co. Ltd., Zhengzhou); AFS-933 atomic fluorescence spectrophotometer (Jitian Instrument Co. Ltd., Beijing).
2.2. Materials characterization
Scanning electron microscope with energy-dispersive spectroscope (SEM-EDS, HITA-CHIS4800, Japan) was applied to investigate the surface structure and morphology of ceria-loaded carbon nanofiber mats and to analyze the surface elements composition of samples. The crystalline microstructures of the samples were measured by x-ray diffraction spectrometry (XRD, Bruker D8 Advance, Germany) within the scope of 5–90°, scanning rate 4° min−1. Fourier transformed infrared (FTIR, Nicolet iS50, USA) optical spectrum of the surface functional groups on the adsorbents were obtained in the wavenumber range of 500–4000 cm−1 under ambient temperature and pressure.
2.3. Preparation of the composite by electrospinning
After the systematic optimization of electrospinning process parameters and the ratio of precursor solution (10%wt PAN) was selected as electrospinning precursor with voltage 15 kV, receiving distance 15 cm, injection speed 0.05 mL h−1 and translation distance 80 mm. 0.1 g of cerium nitrate hexahydrate (CeNO3 · 6H2O), 1.0 g of polyacrylonitrile (PAN) and 10.0 g of N,N-dimethylformamide (DMF) were fully mixed and dissolved to prepare a uniform electrospinning precursor solution with cerium nitrate hexahydrate (CeNO3 · 6H2O) loading of 10%wt PAN. After continuous spinning for 24 h, the complete PAN composite fiber membrane was obtained, and then the membrane loaded with cerium oxide was obtained by heat treatment. A certain amount of composite fiber membrane was placed in a porcelain boat and put into an atmosphere tube furnace. The membrane was pre-oxidized in air with a flow rate of 120 mL min−1, heating up to 270°C from room temperature (heating rate 1°C min−1) and holding for 60 min. Then, the temperature was increased from 270°C to 800°C (ascending speed 5°C min−1) for 60 min with N2 (99.99%) as protective gas. When the temperature cooled down, cerium oxide carbon nanofiber mat (Ce-CNM) was obtained.
2.4. Adsorption experiments
Arsenic solution (100 mL, 1.0 mg L−1) was placed into a conical bottle (250 mL), and then 0.1 g of the composite mat was added. The mixture was oscillated for 24 h (150 r min−1) at room temperature. The residual arsenic in filtrate was detected by an atomic fluorescence photometer. The pH of the aqueous solution was adjusted to the target values (2.0–12.0) using definite concentrations of chlorhydric acid and sodium hydroxide. The adsorption pH for AsIII and AsV adsorption was optimized. The effects of temperature, initial concentration, volume and coexisting ions on the adsorption efficiency were investigated at the optimum pH for AsIII and AsV, respectively.
The adsorption kinetics and adsorption isotherm experiments were performed under the optimal adsorption conditions at room temperature with the initial concentration of 1.0 mg L−1 and in the range of 0.1–10 mg L−1.
In order to investigate the possible interferences by the co-existing ions in water, the effects of co-existing ions on the adsorption were tested. The concentration ratio of co-existing ions to arsenic was 100:1. The co-existing anions were NO3−, SO42-, F−, CO32-, PO43- and SiO32-. The co-existing cations are NH4+, Ca2+ and Mg2+ in water. The concentrations of arsenic and co-existing ions were 1.0 mg L−1 and 100 mg L−1, respectively.
2.5. Regeneration experiments
After the adsorbent was saturated, the adsorbent was put into a 1.0 M NaOH solution. The mixture was shaken for 24 hours at 150 rpm, and then washed to neutral pH with deionized water and dried at room temperature. After desorption, it was re-adsorbed and repeated for five times to evaluate the adsorption efficiency after each adsorption batch.
3. Results and discussions
3.1. Characterizations of material
3.1.1. SEM
shows the SEM photographs from the prepared carbon fiber composites. Compared with the original PAN fibers ()), the carbon fibers () undertook calcination and cerium oxide modification and had a convex surface with a lot of holes and channels. EDS characterization results showed that the convex particles contained Ce elements, which accounted for 0.54% of the total mass. The nanoparticles were further confirmed as CeO2. There were many holes and channels around CeO2 nanoparticles, which might be caused by CeO2 crystallization, Ce(NO3)3 and PAN thermal decomposition during heat treatment, and partial nitrate components decompose and escape [Citation25]. The results showed that the loaded CeO2 nanoparticles did not destroy the structure of carbon nanofibers, and cerium oxide nanoparticles were successfully modified on the carbon nanofibers.
3.1.2. XRD
The crystallinity of nanometer cerium oxide was measured via x-ray diffraction (XRD). In , the obvious protruding peaks at 28.549°, 33.084°, 47.488° and 56.347° are corresponding to the crystal planes of (111), (200), (220) and (311), respectively. The model diffraction peaks of cerium oxide fluorite structure are shown by the red line in , and the diffraction peaks of the composite material coincide with CeO2 (PDF#78-0694), indicating that the cerium precursor was completely converted into CeO2, which had good crystallinity and purity on PAN carbon nanofiber mats during heat treatment [Citation26]. Therefore, CeO2 was effectively modified on PAN carbon nanofiber mats.
3.1.3. FTIR
The composite before adsorption was characterized by FTIR spectrum ()). The wide bandwidth at 3433 cm−1 belongs to the characteristic peak of -OH [Citation27]. After adsorption of AsIII and AsV (), the peak moved to 3421 cm−1 and 3418 cm−1, respectively, and the peak intensity decreased [Citation28]. The peak of 1621 cm−1 is caused by the hydroxyl oscillation in the adsorbed water (H-O-H) on the composite surface [Citation29]. This peak decreased to 1590 cm−1 after adsorption, which indicated that the Ce-CNM adsorbent surface -OH participated in the adsorption. The peak caused by the oscillation of hydroxyl groups attached to metallic oxide (Ce-OH) appears at 1099 cm−1, and the peak at 1389 cm−1 appears is the deformation oscillation of -NH2. After AsIII and AsV adsorption, the characteristic peaks of (Ce-OH) disappeared and moved to 1260 cm−1 and 1262 cm−1 [Citation30]. The phenomenon might be caused by anion exchange of Ce-OH and arsenic anions, culminating in the formation of the Ce-O-As bond [Citation20].
3.2. Adsorption of arsenic species
3.2.1. pH dependent adsorption of AsIII and AsV
The adsorption of AsIII and AsV by the composites under strongly acidic conditions (pH < 4.0) gradually increased with the increase of pH (). When the pH was above 4.0, the adsorption quantity for AsV decreased, while that for AsIII still increased and then kept constant between pH 6.0 and pH 10.0. In contrast, the adsorption efficiency of AsV sharply decreased when pH was higher than 4.0. It was notable that the adsorption efficiencies are significantly discriminated for AsIII and AsV under the strong alkaline conditions (pH>11, 87.8% for AsIII and 0.92% for AsV, respectively). The adsorption efficiency of AsV was 96.97% at pH 4.0, while less than 1.2% at pH 12.0. Based on the above results, the following conclusion could be reached that the as-prepared composite had a strong pH dependent property for selective adsorption of arsenic species. The existing states of inorganic arsenic ions (AsIII and AsV) are dominated by pH. When pH is below 9.23, AsIII ion is mostly present as H3AsO3, and when pH is above 9.23, it exists as H2AsO3−, HAsO32- and AsO33-. AsV mainly exists as electronegative ions (H2AsO4− and HAsO42-) in the restriction of pH 2.24–11.6. When pH is below 2.24, it is in the species of H3AsO4−. When pH is above 11.6, AsV is mainly in the form of AsO43-. The pHzpc of CeO2 in aqueous solution is about 6.7. When pH is below 6.7, CeO2 in solution is positively charged, and negatively charged when pH is above 6.7 [Citation3].
As to pentavalent arsenic, it is in the modality of H2AsO4− or HAsO42- in neutral or alkaline solutions. Negatively charged CeO2 needs to overcome the electrostatic repulsion force to adsorb AsV. As the alkalinity increases, the negative charge and amounts of hydroxyl groups on the surface of CeO2 increase. The enhancement of the electrostatic repulsion makes it more difficult to adsorb AsV. Under weak acidic conditions, chemisorption and electrostatic attraction exist between pentavalent arsenic and positively charged CeO2, which facilitates the adsorption of arsenic [Citation31].
The composite had stronger adsorption ability for AsIII in a wider range of pH compared with AsV. It was obvious that the adsorbent could also effectively remove AsIII even under the strong alkaline conditions (pH>11) (). The electrostatic repulsion between AsIII and adsorbent is weaker than that of AsV [Citation32]. The hydroxyl groups on the composite play key roles for adsorption [Citation7,Citation33]. Under the conditions at low pH values, the proton reaction occurs between the unbound hydrogen ions in solution and hydroxyl groups on composites, which results in the reduction of AsIII adsorption. When pH is high, the adsorbance of AsIII slightly decreased owing to the negative charges and the electrostatic repulsion force [Citation34].
3.2.2. Temperature influence
Temperature is always considered as one of the important factors on adsorption. The adsorption efficiencies of arsenic species were evaluated at 25°C, 35°C and 45°C, keeping the other factors unchanged. showed that the composite exhibited different adsorption properties for AsIII and AsV at different temperatures. When the temperature increased from 25°C to 45°C, the adsorption efficiency increased by 9.3% for AsIII and 5.8% for AsV, respectively.
3.2.3. Initial concentration influence
In order to investigate the possible effects of the concentration on the adsorption performances, the adsorption efficiencies with various initial concentrations (0.1 ~ 10.0 mg L−1) were determined under the optimal conditions. The adsorption efficiencies decreased when the initial concentration increased, which is possibly caused by the inadequate reactive sites on the surface of the adsorbents () [Citation35]. We found that the adsorption efficiency of AsIII was lower than that of AsV at high concentrations. The results demonstrated that the composite was able to reduce arsenic concentration below the drinking water criteria recommended by WHO (0.01 mg L−1) [Citation36], which enable the composite to have a bright application prospect for drinking water treatment.
Figure 6. (a) The adsorption efficiencies of arsenic at various initial concentrations. (b) Selective kinetic adsorption of AsIII at different initial concentrations by Ce-CNM (pH = 12)
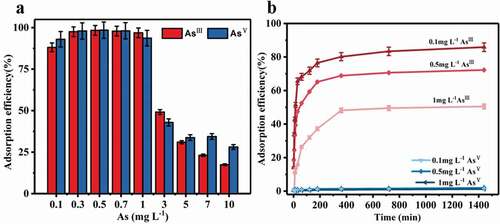
Using our prepared composite mat as adsorbent, AsIII could be selectively adsorbed by pH adjusting (pH>12). The selective adsorption properties of AsIII on the composite were investigated with different initial concentrations of AsIII (0.1–1.0 mg L−1) (). The results showed that the mat had good selective adsorption properties for trivalent arsenic with broad concentration range under strong alkaline conditions.
3.2.4. Volume influence
The influences of different solution volumes on the properties of the composites were investigated under the optimal conditions (). The arsenic adsorption efficiency kept constant when the solution volumes were from 10 mL to 100 mL. The results indicated that the influence of solution volume on adsorption was very limited.
3.2.5. Coexisting ions influence
The adsorption efficiencies of AsIII and AsV with various co-existing ions were studied (). It was obvious that 100 times high concentrations of CO32-, F−, and NO3− had little negative effects on the adsorption. However, SiO32- and PO43- showed non-ignorable negative effects on adsorption. When high concentrations of SiO32- and PO43- were presented, the removal efficiencies decreased by 9% and 11% for AsIII, and by 28.3% and 13.7% for AsV, respectively. Phosphorus and arsenic are elements in the same family, while silicon and phosphorus are adjacent and have a similar radius. Moreover, phosphate (PO43-) and arsenate ions (AsO43-) have the similar tetrahedral configuration. The proximity of chemical property and the similar tetrahedral spatial structures enable SiOs2- and PO43- to compete with AsO43- during adsorption [Citation37]. The coexisting ions can bind with active sites and form inner orbital complexes, which results in a decrease in adsorption [Citation29]. Fortunately, the silicate and phosphate concentrations in natural water are generally low, and cannot cause obvious negative effects on adsorption [Citation38].
3.3. Adsorption dynamics
The adsorption rates of AsIII and AsV by the composite were tested under the optimization conditions. demonstrated that the adsorption efficiencies for both AsIII and AsV reached more than 50% within 15 minutes. At the prime stage, there were huge amounts of active sites on the composites available for arsenic. When more arsenic ions in solution diffuse to the active sites, the adsorption resistance increases. Finally, the pore diffusion becomes the dominant process, and the adsorption gradually reaches equilibrium. The adsorption data were fitted via first-order adsorption kinetic model formula (1) and second-order kinetic model formula (2):
Figure 9. (a) Adsorption kinetics of different arsenic species; (b) Pseudo second order dynamical linear fit of AsIII and AsV
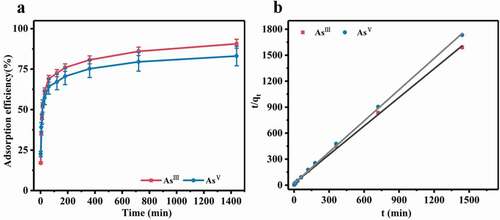
qe means the saturated adsorption efficiency (mg g−1); qt means the equalized adsorption efficiency (mg g−1); t is the time (min); K1 and K2 refer to the kinetic rate constants of the first-order model formula (min−1) and second-order model formula (g mg−1 min−1) (). The adsorption capacities via the first order kinetic model formula were in consistent with the experimental values. Compared with the first order kinetic equation (R2 = 0.8217 for AsIII and R2 = 0.8534 for AsV), the kinetic coefficients (R2) of second-order equation are 0.9989 and 0.9991, and the theoretical adsorption capacity calculated by fitting was in agreement with the experimental value. The data points in are uniformly distributed on the fitting lines, indicating the adsorption is preferably dominated by the second-order kinetic model and mainly chemical adsorption control [Citation39].
Table 1. Correlation kinetic fitting parameters of arsenic by Ce-CNM
3.4. Adsorption isotherm
The thermodynamic processes of AsIII and AsV adsorption (0.1–10.0 mg L−1) were studied by fitting the experimental data with Langmuir and Freundlich isothermal models.
Langmuir isothermal adsorption equation is as follows:
qmax is the saturated adsorption efficiency (mg g−1); kL represents constant (L mg−1); ce represents the balance concentration (mg L−1).
Freundlich isotherm equation is as follows:
qe represents the equalized adsorbance (mg g−1); kF represents the constants of Freundlich formula (mg g−1), and n represents the Freundlich adsorption index. The parameters related to Langmuir and Freundlich formula are listed in . clearly illustrates that all the points were evenly distributed on both sides of the line. Although the curve in shows that the maximum adsorption capacity of AsIII was lower than AsV, the thermodynamic fitting parameter kL of AsIII was higher than that of AsV, denoting that the composite had stronger affinity for AsIII at low concentrations. The data in shows that the R2 values of Langmuir isotherm were 0.9969 (AsIII) and 0.7571 (AsV), and the R2 values of Freundlich isotherms were 0.9969 (AsIII) and 0.9135 (AsV), respectively. The adsorption of AsIII can be better described by monolayer adsorption process [Citation40,Citation41].
Table 2. Adsorption isotherms fitting parameters of arsenic on Ce-CNM
3.5. Regenerating and reusability
The adsorbents were regenerated by immersing the used mats in 100 mL of NaOH solution with varying concentrations (0–4.0 mol L−1) (). The optimized concentration of NaOH solution was 1 M with the desorption efficiencies of 97.7% (AsIII) and 98.5% (AsV). The composite mat can keep satisfactory adsorption efficiency for AsIII for the first recycle, but the recycle adsorption efficiency for AsV obviously decreased compared with the virgin one (30% for AsV after 5 cycles) (). The results were in agreement with the previous findings that the regeneration of the adsorbent was normally better for AsIII than AsV [Citation32]. This might be related to the more stable formation of internal orbital complex of AsV with the active center of cerium oxide on the adsorbent [Citation11]. Although the regeneration ability of the mat is limited, it is still economically valuable and environment-friendly for its easy collection without centrifugation after adsorption, which can reduce both the treatment cost and the risk of possible secondary contamination caused by nanoparticle release.
4. Conclusion
One CeO2-loaded carbon nanofiber mat was successfully prepared by electrospinning and applied to removal arsenic in water. The CeO2 nanoparticles were stably embedded and uniformly dispersed in carbon nanofibers. The experimental results showed that the composites performed good adsorption capacities for AsIII and AsV. The adsorption was pH and species dependent. The selective removal of AsIII from AsV can be successfully reached under strong alkaline conditions (pH>11). The used mats can be easily collected from the solution after use for regeneration, which greatly reduced the environmental risks caused by the possible nanoparticle release from the composite.
Authors’ contributions
Xiaoyang Wei did the experiment, analyzed data and wrote the draft. Qi Guo performed materials design and electrospinning, Yuan Li contributed to the design of the experiment and data analysis, Liwei Zheng did the experiment and collected the data, Zhiqiong Li did the work for electrospinning training, Kegang Zhang guided students to do experiment, Chun-Gang Yuan designed the experiment, was a major contributor in writing and revising the manuscript. All authors read and approved the final manuscript.
Availability of data and materials
All data generated or analysed during this study are included in this article.
Disclosure statement
The authors declare that they have no competing interests.
Additional information
Funding
References
- Zhang S, Bai J, Wang W, et al. Heavy metal contents and transfer capacities of phragmites australis and Suaeda salsa in the Yellow River Delta, China. Phys Chem Earth. 2018;104:3–8.
- Antón MAL, Somoano MD, Fierro JLG, et al. Retention of arsenic and selenium compounds present in coal combustion and gasification flue gases using activated carbons. Fuel Process Technol. 2007;88:799–805.
- Masue Y, Loeppert RH, Kramer TA. Arsenate and arsenite adsorption and desorption behavior on coprecipitated aluminum: ironhydroxides. Environ Sci Technol. 2007;41:837–842.
- Moe B, Peng H, Lu X, et al. Comparative cytotoxicity of fourteen trivalent and pentavalent arsenic species determined using real-time cell sensing. J Environ Sci. 2016;49:113–124.
- Lian F, Liu X, Gao M, et al. Effects of Fe-Mn-Ce oxide-modified biochar on As accumulation, morphology, and quality of rice (Oryza sativa L.). Environ Sci Pollut Res. 2020;27:18196–18207.
- Zhang M, Zhao Q, Xue P, et al. Do Si/As ratios in growth medium affect arsenic uptake, arsenite efflux and translocation of arsenite in rice (Oryza sativa)? Environ Pollut. 2017;229:647–654.
- Asere TG, Verbeken K, Tessema DA, et al. Adsorption of As(III) versus As(V) from aqueous solutions by cerium-loaded volcanic rocks. Environ Sci Pollut R. 2017;24:20446–20458.
- Tong S, Deng H, Wang L, et al. Multi-functional nanohybrid of ultrathin molybdenum disulfide nanosheets decorated with cerium oxide nanoparticles for preferential uptake of lead (II) ions. Chem Eng J. 2018;335:22–31.
- Wang Y, Du Y, Yan J, et al. TiO2 pillared montmorillonite in-situ growth of CeOx/MnOy nanoparticles for effective arsenic (III) adsorption in wastewater. Environ Sci Pollut R. 2020;27:17986–17996.
- Zhang S, Niu H, Cai Y, et al. Arsenite and arsenate adsorption on coprecipitated bimetal oxide magnetic nanomaterials: MnFe2O4 and CoFe2O4. Chem Eng J. 2010;158:599–607.
- Gupta K, Bhattacharya S, Nandi D, et al. Arsenic(III) sorption on nanostructured cerium incorporated manganese oxide (NCMO): a physical insight into the mechanistic pathway. J Colloid Interf Sci. 2012;377:269–276.
- Sun W, Li Q, Gao S, et al. Exceptional arsenic adsorption performance of hydrous cerium oxide nanoparticles: part B. Integration with silica monoliths and dynamic treatment. Chem Eng J. 2012;185–186:136–143.
- Zhang D, Niu H, Zhang X, et al. Strong adsorption of chlorotetracycline on magnetite nanoparticles. J Hazard Mater. 2011;192:1088–1093.
- Singh K, Travlou NA, Bashkova S, et al. Nanoporous carbons as gas sensors: exploring the surface sensitivity. Carbon. 2014;80:183–192.
- Zhang Y, Shang Z, Shen M, et al. Cellulose nanofibers/reduced graphene oxide/polypyrrole aerogel electrodes for high-capacitance flexible all-solid-state supercapacitors. Acs Sustain Chem Eng. 2019;7:11175–11185.
- Zhang J, Rao Q, Jin B, et al. Cerium oxide embedded bilayer separator enabling fast polysulfide conversion for high-performance lithium-sulfur batteries. Chem Eng J. 2020;388:124120.
- Lu P, Qiao B, Lu N, et al. Photochemical deposition of highly dispersed Pt nanoparticles on porous CeO2 nanofibers for the water- gas shift reaction. Adv Funct Mater. 2015;25:4153–4162.
- Zhang Z, Gao D, Xue D, et al. Co and CeO2 co-decorated N-doping carbon nanofibers for rechargeable Zn–air batteries. Nanotechnology. 2019;30:395401–395408.
- Sordello F, Berruti I, Gionco C, et al. Photocatalytic performances of rare earth element-doped zinc oxide toward pollutant abatement in water and wastewater. Appl Catal B-environ. 2018;18:31206–2.
- Chen B, Zhu Z, Ma J, et al. Surfactant assisted Ce–Fe mixed oxide decorated multiwalled carbon nanotubes and their arsenic adsorption performance. J Mater Chem A. 2013;1:11355.
- Gao X, Liu S, Zhang Y, et al. Physicochemical properties of metal-doped activated carbons and relationship with their performance in the removal of SO2 and NO. J Hazard Mater. 2011;188:58–66.
- Yu Y, Zhang C, Yang L, et al. Cerium oxide modified activated carbon as an efficient and effective adsorbent for rapid uptake of arsenate and arsenite: material development and study of performance and mechanisms. Chem Eng J. 2017a;315:630–638.
- Liu Y, Sun X, Zhou Z, et al. Electrospun Ce–Ni–O composite nanofibers for highly selective propane detection at high temperature based on its rapid reaction kinetics. J Mater Chem A. 2014;2:14038.
- Jing M, Zhang X, Fan X, et al. CeO2 embedded electrospun carbon nanofibers as the advanced electrode with high effective surface area for vanadium flow battery. Electrochim Acta. 2016;16:31819–9.
- Cui Q, Dong X, Wang J, et al. Direct fabrication of cerium oxide hollow nanofibers by electrospinning. J Rare Earth. 2008;26:664–669.
- Shan C, Dong H, Huang P, et al. Dual-functional millisphere of anion-exchanger-supported nanoceria for synergistic As(III) removal with stoichiometric H2O2: catalytic oxidation and sorption. Chem Eng J. 2018;18:31284–1.
- Guo H, Li W, Wang H, et al. A study of phosphate adsorption by different temperature treated hydrous cerium oxides. Rare Met. 2011;30:58–62.
- Li R, Li Q, Gao S, et al. Exceptional arsenic adsorption performance of hydrous cerium oxide nanoparticles: part A. Adsorption capacity and mechanism. Chem Eng J. 2012;185–186:127–135.
- Wen Z, Lu J, Zhang Y, et al. Facile inverse micelle fabrication of magnetic ordered mesoporous iron cerium bimetal oxides with excellent performance for arsenic removal from water. J Hazard Mater. 2019;383:121172.
- Li Z, Deng S, Yu G, et al. As(V) and As(III) removal from water by a Ce–Ti oxide adsorbent: behavior and mechanism. Chem Eng J. 2010;161:106–113.
- Wu B, Lo IMC. Surface functional group engineering of CeO2 particles for enhanced phosphate adsorption. Environ Sci Technol. 2020;54:4601–4608.
- Yu Y, Zhang C, Yang L, et al. Cerium oxide modified activated carbon as an efficient and effective adsorbent for rapid uptake of arsenate and arsenite: material development and study of performance and mechanisms. Chem Eng J. 2017b;315:630–638.
- Sharma R, Singh N, Gupta A, et al. Electrospun chitosan – polyvinyl alcohol composite nanofibers loaded with cerium for efficient removal of arsenic from contaminated water. J Mater Chem A. 2014;2:16669–16677.
- Olivera S, Chaitra K, Venkatesh K, et al. Cerium dioxide and composites for the removal of toxic metal ions. Environ Chem Lett. 2018;16:1233–1246.
- Bi X, Zeng C, Westerhoff P. Adsorption of arsenic ions transforms surface reactivity of engineered cerium oxide nanoparticles. Environ Sci Technol. 2020;54:9437–9444.
- Liu X, Gao M, Qiu W, et al. Fe-Mn-Ce oxide-modified biochar composites as efficient adsorbents for removing As(III) from water: adsorption performance and mechanisms. Environ Sci Pollut R. 2019;26:17373–17382.
- Feng Y, Lu H, Liu Y, et al. Nano-cerium oxide functionalized biochar for phosphate retention: preparation, optimization and rice paddy application. Chemosphere. 2017;185:816–825.
- Yin Y, Yang X, Zhou X, et al. Water chemistry controlled aggregation and photo-transformation of silver nanoparticles in environmental waters. J Environ Sci. 2015;34:116–125.
- Zhou Y, Jin Q, Zhu T, et al. Adsorption of chromium (VI) from aqueous solutions by cellulose modified with-CD and quaternary ammonium groups. J Hazard Mater. 2011;187:303–310.
- Zhou H, Gao B, Zhou Y, et al. Facile preparation of 3D GO/CNCs composite with adsorption performance towards [BMIM][Cl] from aqueous solution. J Hazard Mater. 2017;337:27–33.
- Zhou Y, Wang X, Zhang M, et al. Removal of Pb(II) and malachite green from aqueous solution by modified cellulose. Cellulose. 2014;21:2797–2809.