ABSTRACT
M-dichlorobenzene can be degraded through biotrickling filter (BTF). However, its degradation needs to be enhanced, and the mechanism of enhancement is unclear. In this study, Zn(II) was taken as the biological growth promoter; the polysaccharides and proteins, zeta potential, Fourier transform infrared spectroscopy, x-ray photoelectron spectroscopy, and the enzyme activity of the strains were analyzed. The results indicated that Zn(II) at 5mg/L promoted the growth of the strain. When the inlet concentration was 505.74 mg/m3 and the empty bed resistance time (EBRT) was 90 s, the removal efficiency of BTF2 was 86.15%. In BTF2, the enzyme activity, polysaccharide, and protein content increased, while the zeta potential decreased. The changes of zeta potential indicated that there existed an electrostatic interaction between extracellular polymeric substance (EPS) and Zn(II). The results of Fourier transform infrared spectroscopy and x-ray photoelectron spectroscopy confirmed that there was a bond between the EPS surface functional groups and Zn(II).
1. Introduction
Dichlorobenzene is used as an intermediate or solvent widely in many industries such as dyes, medicines, and pesticides. When it is exposed to the environment, m-dichlorobenzene is toxic to human endocrine, immune and reproductive systems. M-dichlorobenzene also has a certain risk of carcinogenesis [Citation1]. Besides, m-dichlorobenzene and its derivatives can be enriched through the food chain, which can cause irreversible damage to the ecosystem [Citation2]. As a result, m-dichlorobenzene is classified as a priority pollutant by the US Environmental Protection Agency [Citation3]. Therefore, we should pay more attention to the harmless treatment of m-dichlorobenzene.
The degradation of volatile organic pollutants by BTF has attracted more and more attention due to the advantages of no secondary pollution, simple operation and low cost [Citation4–6]. In the BTF, volatile organic pollutants diffused to the surface of the biofilm through the liquid phase, then the pollutants were converted into harmless substances through the metabolism of microorganisms [Citation7]. For instances, Dewidar applied a lab-scale BTF inoculated with a mixed fungal consortium to treat 2-ethylhexanol, and the maximum elimination capacity of 2-ethylhexanol was 32 g/m3·h [Citation8]. Zhang reported that two biogas-purification systems for simultaneous efficient removal of H2S and decamethylcyclopentasiloxane, the results showed that high elimination for both H2S (1.86 kg/(m3·d) and D5 (0.282 kg/(m3·d)) was achieved [Citation9]. When the inlet loading ranges were 18–36 g/m3·h for toluene and 0–225 g/m3·h for methanol, respectively, the removal efficiency of toluene varied from 30 to 80%, while removal efficiency of methanol remained almost constant at > 90% [Citation10]. Sun reported that ceramic pellets were used as packing material to establish a BTF to purify waste gas containing styrene, and results indicated that the removal efficiency (RE) of styrene could be reached up to 95% [Citation11]. Aguirre reported that in BTF, the removal efficiencies were 100% for ammonia and H2S, 73% for methanol, 63% for trimethylamine, 41% for dimethylsulphide, and 32% for 2,5-dimethylpirazyne at day 538, respectively [Citation12]. Previous studies have proven that volatile organic pollutants can be degraded by BTF.
However, in the traditional BTF, both the utilization efficiency of microorganisms and the microbial activity were low, which resulted in poor degradation performance [Citation13]. To improve the performance of BTF to degrade volatile organic pollutants, it is necessary to strengthen the degradation performance of the BTF system. It has been reported that the addition of a suitable concentration of metal ions as a biological growth promoter can promote the growth and activity of microorganisms. For example, Co(II) at a low concentration level can promote the growth of serratia marcescens [Citation14]. Calcium and magnesium ions can promote the activity of microorganisms and effectively improve the diversity of microorganisms [Citation15]. Some metal ions are cofactors of microbial cell enzymes, such as Cu(Zn)-superoxide dismutase, carbonase and alcohol dehydrogenase [Citation16]. Ding reported that Zn (II) promote the production of polysaccharides and proteins in EPS; in addition, specific EPS produced by B. vallismortis in the presence of Zn(II) could have a wide range of potential applications, allowing optimization and improvement of the capacity of EPS to remove heavy metals from effluent [Citation17]. In the solid culture, when the concentration of Zn(II) was 10–100 mg/L, the growth was accelerated; in the liquid culture, when the concentration of Zn(II) was 60 mg /L, the polysaccharide content of the mycelia was the highest, which was increased by 141.8% compared with the control group [Citation18]. Metal ions can not only promote the growth of microorganisms but also can be applied to the degradation of pollutant gas in BTF. For example, Liu reported that the average elimination capacity and removal efficiency of chlorobenzene in the presence of Fe3+ and Zn2+ increased from 61.54 to 65.79 g/m3·h and from 80.93% to 89.37%, respectively, at an EBRT of 60s [Citation19]. Wang studied the effects of Zn(II) on biological removal of ethylbenzene in BTF and the optimal concentrations of Zn(II) was 1 mg L−1, the removal efficiency increased from 86% to 90% [Citation20]. Previous research had been confirmed that metal ions can promote the activity of microorganisms in the BTF, but the enhancing mechanism is not yet clear. Therefore, it is worthy of exploring the mechanism of metal ions to promote biodegradation performance.
In this study, m-dichlorobenzene was selected as the target pollutant, and Zn(II) was added as a biological growth promoter. The main aims were as follows: (1) to determine the appropriate concentration of Zn(II) to be added; (2) to build Zn(II) enhanced degradation system and measure the degradation performance in the BTF; (3) to analyze the mechanism of Zn(II) enhanced degradation system. An enhanced technology to improve microbial activity was provided in this research, which can support a technical reference for the biological treatment of volatile organic pollutants, especially for m-dichlorobenzene.
2. Materials and methods
2.1. Chemicals, culture media and inoculation bacteria
M-dichlorobenzene with a purity of 99.5% was purchased from Shanghai Jingchun Biochemical Technology Company. As a bio-enhancer, ZnCl2 with a purity of 98% was purchased from General-Reagent Company. LB solid medium: Take 4 g of LB solid medium powder, dilute to 100 mL, adjust the pH to 7, and sterilize for use. Inorganic salt culture medium: K2HPO410.5 g, KH2PO4 4.5 g, (NH4)2SO4 1 g, dilute to 1000 mL, adjust the pH to 7, and sterilize for use. Circulating nutrient solution composition: MgSO4 0.03 mg/L, CoCl2•6H2O 0.42 mg/L, AlCl3•6H2O 0.05 mg/L, MgSO4 0.067 g/L, Na2MoO4•2H2O 0.15 mg/L, K2HPO4 0.14 g/L, KH2PO4 0.04 g /L, (NH4)2SO4 0.01 g/L, glucose 0.08 mg/L, pH 7.0–8.0.
Strain DH-1, which belongs to Bacillus agrobacterium, was screened from the root-soil of an annual potted reed plant in Yancheng Institute of Technology [Citation2]. LB solid medium is used for strain activation, and inorganic salt medium is used for strain cultivation.
2.2. Operation condition of BTFs
In this study, two parallel BTFs (BTF1 and BTF2) were used to treat m-dichlorobenzene contaminated gas (schematic of the BTF system was shown in ). The simulated gas formed by mixing dichlorobenzene and air by the air pump was the target pollution gas. 3 m3/h of simulated gas was blown into the BTF (diameter 100 mm; height 700 mm) by the pump. Hollow spheres and polyurethane sponge blocks were mixed according to a volume ratio of 1:1 as the combined packing materials of the BTF. These combined packing materials have advantages of high load, easy cleaning, good air permeability and strong water absorption [Citation21,Citation22]. Also, BTF1 was a blank condition without any addition, and BTF2 was added with Zn(II). The optimal concentration of Zn(II) was determined by shaking flask experiments. The process of the shake flask experiment is as follows: (1) 120 mg/L of m-dichlorobenzene, 10% concentration of bacteria liquid, and different concentrations of Zn(II) were added to 50 mL of the inorganic salt medium; (2) culturing the above inoculum for 48 h at 25°C; (3) measuring the concentration of m-dichlorobenzene and the OD600 of the bacterial solution and determine the optimal concentration of Zn(II) based on experimental data.
Figure 1. The schematic of the BTF system. (1) Air compressor, (2) gas valve, (3) buffer tank, (4) rotameter, (5) m-Dichlorobenzene gas generator, (6) mixed gas tank, (7) inlet gas sample port, (8) BTF column, (9) nutrient solution tank, (10) peristaltic pump, (11) packaging material, (12) outlet gas sample port
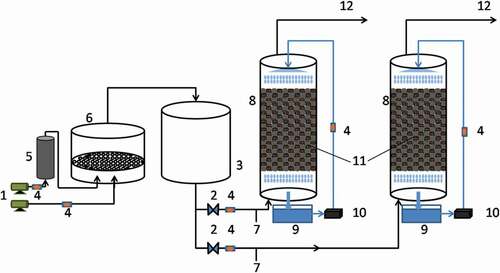
The experiment temperature was kept at 25°C. After the packing material was soaked in the pure bacterial solution for a week, it was put into the BTFs. The BTFs have been in operation for 100 days, 0–25 days were the start-up operation stage of BTFs, and 26–100 days were the stable operation stage. The intake air concentration was controlled at 0–1000 mg/m3, the empty bed residence time was 30 s, 60 s, and 90 s, and the spray volume of each tower body was 10 L/h.
2.3. Analytical methods
The headspace method was used to record the peak area of m-dichlorobenzene on a gas chromatograph (Clarus580, PerkinElmer, USA), and the residual concentration value of m-dichlorobenzene was obtained by external standard method [Citation23]. The working conditions of the gas chromatograph were as follows: the initial value of the column temperature is 40°C, the highest value is 220°C, the heating rate is 10°C/min; the vaporization chamber temperature is 220°C; the detector temperature is 300°C. The standard curve is shown in Fig. S1.
To explore the enhancement mechanism of Zn(II) on the biodegradation of m-dichlorobenzene, the polysaccharides and proteins, zeta potential, Fourier transform infrared spectroscopy, x-ray photoelectron spectroscopy, and the enzyme activity of the strains were analyzed. To avoid interference with the operation of BTF, several sets of shake flask experiments were run to extract the EPS and enzymes of the strains. The operating conditions of the shake flask experiment refer to section 2.2. EPS was extracted with an improved thermal extraction method [Citation24]. The concentrations of polysaccharide and protein were determined by the anthrone method and the improved Lowry method [Citation25,Citation26]. Zetasizer Nano ZS90 was used to determine the Zeta potential value of EPS, and the sample processing method was referred to literature [Citation27]. EPS functional groups were analyzed by XPS (Thermo, America) and FTIR (Thermo, America) analysis. FTIR analysis method: 1 mg EPS powder sample is mixed with spectral pure potassium bromide (KBr) and pressed into tablets, the scanning wavelength is 400–4000 cm−1.
3. Results and discussion
3.1. Optimal concentration of Zn(II)
shows the OD600 and removal efficiency (RE) of m-dichlorobenzene under different Zn(II) concentrations. In this study, the addition of Zn(II) caused the variability of strain growth. When the concentration of Zn(II) was 0–2 mg/L, OD600 and RE increased with Zn(II) concentration. When the concentration of Zn(II) is 2 mg/L, OD600 and RE reach a peak. As the concentration increased, OD600 and RE of m-dichlorobenzene both decreased. And then both the OD600 and RE reached a higher peak at 5 mg/L compared to 2 mg/L. Zn(II) at 2 mg/L can promote the growth of microorganisms. When the concentration of Zn(II) increased, Zn(II) at 3 mg/L may have some short-term inhibitory effects on the cell membrane surface, resulting in inhibition of the growth of microorganisms. The possible reason is that the strains cannot adapt to the increasing concentration of Zn(II) in the beginning. Wang also found similar results that when the concentration of metal ions was less than 20 mg/L, it had a certain promotion effect on microbial activity; after 72 h, when the concentration of Al3+ and Cu2+ increased to more than 60 mg/L, the adaptability of microorganisms to metal ions gradually decreased [Citation28]. The sampling time for the shake flask experiment is only 48th hours. In future studies, the sampling time should be appropriately extended, such as 72nd hour, 96th hour, or even longer, to verify whether Zn(II) at 3 mg/L can promote the growth of microorganisms under a longer culture time.
Figure 2. The effect of Zn(II) concentration on the growth of strains and the degradation of m-dichlorobenzene
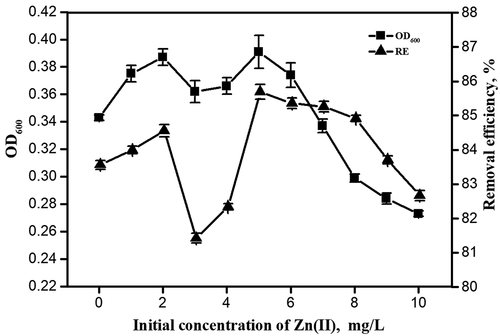
When 5 mg/L of Zn(II) was added, a relatively higher peak appeared, the OD600 was 0.39, which was 0.05 higher than the blank, indicating that the growth ability of the strains was greatly improved after adding an appropriate concentration of Zn(II); the RE of m-Dichlorobenzene was 85.69%, which was 2.13% higher than the blank. The possible reason is that Zn(II) at 5 mg/L could quickly establish material exchange channels through the cell membrane, which can promote the growth and degradability of microorganisms. When the concentration of Zn(II) was higher than 5 mg/L, the strains may be poisoned by high concentrations of heavy metals, inhibiting the aerobic synthesis and decomposition of microorganisms, resulting in a decrease in the number of strains and the removal rate of m-dichlorobenzene. Other researchers also have similar results. For instance, Xu studied the short-term and long-term effects of Mn2+ on the performance of anammox granular sludge. With the increase of Mn2+ concentration, the activity of anammox bacteria, heme C and extracellular polymer content increased first and then decreased [Citation29]. Wiens also found that Fe3+ (≤5 mu M) can promote the accumulation of microbial biomass, speed up their domestication process, and promote the secretion of extracellular polymeric substances [Citation30]. Therefore, the appropriate concentration of the biological growth promoter Zn(II) was 5 mg/L. When the concentration was lower or higher than the appropriate concentration, the strains were inhibited and the growth ability was significantly weakened.
3.2. Effect of Zn(II) on the degradation of m-dichlorobenzene in the BTF
The performance of the BTF is illustrated in . In the start phase, the intake air concentration was 1000 mg/m3. In 0–17 days, the RE of BTFs showed volatility changes. In 1–3 days, the RE of BTFs increased rapidly; in 4–6 days, the RE of BTFs decreased rapidly. The RE of BTFs in 7–17 days and 1–3 days had similar changes. The change in RE may be due to the adaptation of the microorganisms in the filler to the m-dichlorobenzene atmosphere. On the 25th day, the removal efficiency of m-dichlorobenzene gradually stabilized, the RE of BTF1 was 75.21% and BTF2 was 78.41%. The data showed that both BTF1 and BTF2 can be started on the 25th day. After the start phase, we studied the influence of different inlet gas concentrations and empty bed residence time on the performance of BTF1 and BTF2.
Keeping the intake air concentration at 1000 mg/m3 for 10 d, the REs of BTF1 and BTF2 both increase steadily. When the EBRT was 30 s, 60 s and 90 s, the REs of BTF1 were 68.56%, 75.40% and 79.01%, respectively; the REs of BTF2 were 71.76%, 78.60% and 82.21%, respectively. Keeping the intake air concentration at 500 mg/m3 for 10 d, the REs of BTF1 and BTF2 were significantly increased. When the EBRT was 30 s, 60 s, and 90 s, the removal rates of BTF1 were 73.81%, 81.56%, and 83.95%, respectively; the removal rates of BTF2 were 77.01%, 84.76%, and 86.15%, respectively. In order to eliminate interference, before changing the operating conditions of the trickling filter, control the intake air concentration to 0 mg/m3 and run for 5 d. When the intake air concentration was 0 mg/m3, the corresponding removal efficiency after 40 days, 70 days, and 95 days suddenly increased. On the one hand, the decrease of the intake air concentration led to the decreasing of the outlet air concentration; on the other hand, it also showed that microorganisms could effectively degrade m-dichlorobenzene with decreasing concentration.
Experimental data show that the addition of Zn(II) can promote the degradation efficiency of m-dichlorobenzene. In the stable operation stage, the degradation of m-dichlorobenzene of BTF2 was better than that of BTF1. Hao reported that Fe2+, Mn2+ and Zn2+ can improve the desulfurization efficiency of the BTF by 7 to 31%, and the corresponding mass concentration ratios are: 2%, 0.2%, and 0.5%, respectively [Citation31]. Zhang found that Mn2+ at the concentration of 35 mg/L can promote the RE of benzene in the BTF, and the RE reached a maximum of 91.7% [Citation32]. In addition, low inlet gas concentration and long empty bed residence time both can promote the RE of m-dichlorobenzene. When the gas concentration was 1000 mg/m3, the removal efficiency of m-dichlorobenzene decreased; when the gas concentration was reduced to 500 mg/m3, the RE of m-dichlorobenzene increased. High concentrations of exhaust gas have an inhibitory effect on the growth of microorganisms [Citation33]. Previous researches have similar results. Yousefinejad reported that REs >80% were obtained for the inlet concentrations > 3.5 g/m3, but a slight decrease of RE to 75% was observed for toluene concentrations ranging from 3.5 g/m3 to 3.8 g/m3 [Citation31]. He reported that the removal rate of dimethyl sulfide decreased with the decrease of EBRT. When the EBRT is 60 s, the removal rate of dimethyl sulfide is 75%; when the EBRT is 45 s, the removal rate of dimethyl sulfide is reduced to 50% [Citation34]. Studies have shown that the reduction of EBRT (90 s, 60 s, 30 s) is also not conducive to the tolerance of microorganisms to high concentrations of exhaust gas, and will lead to the accumulation of toxic metabolites and the deterioration of BTF performance [Citation35].
3.3. The mechanism of Zn(II) on the enhanced biodegradation of m-dichlorobenzene
3.3.1. The effect of Zn(II) on polysaccharides and proteins
Extracellular polymeric substances (EPS) generally exist on the surface of microorganisms, which can enrich nutrients in the environment and degrade them into small molecules by extracellular enzymes. To understand the enhancement effect of Zn(II), the polysaccharides and proteins in EPS was measured. As shown in , the polysaccharides increased significantly after 16 h. In the 0–24 h, the strains were inhibited by the toxicity of Zn(II), and the polysaccharide in EPS was lower than the blank; the strains gradually grew and reproduced after 32 h, the polysaccharide gradually increased. The polysaccharides content was the highest at 48 h, which increased by 45.89 ± 1.98 mg/L compared with the blank. As shown in , the protein content increased and then decreased. At 0–24 h, the protein content in EPS was inhibited by Zn(II); then as the tolerance of strains increased, the protein content increased. At 48 h, the protein content was the highest, which increased by 6.36 ± 0.33 mg/L. Compared with the blank group, the change trends of polysaccharide and protein in the Zn(II) added group were similar. It is concluded that Zn(II) at optimal concentration can promote the increase of polysaccharide and protein in EPS. It may be due to the ‘dilution effect’ of microorganisms and the functional hindrance of DH-1 bacteria, which reduced the toxicity of Zn(II) and promoted the growth of microorganisms [Citation36]. On the other hand, Zn(II) can also be used as an electron acceptor for microbial respiration, growth, and metabolism [Citation37].
3.3.2. Changes in enzyme activity
Catechol is an important intermediate product in the degradation process of aromatic compounds. Catechol dioxygenase is the key enzyme to degrade catechol. Under the catalysis of catechol dioxygenase, polycyclic aromatic hydrocarbons are converted into long carbon chains and enter the tricarboxylic acid cycle, and then degrade into carbon dioxide and water [Citation38]. According to the different ring opening positions, catechol dioxygenase can be divided into catechol 1, 2-dioxygenase (C12O) and catechol 2, 3-dioxygenase (C23O) [Citation39]. In this study, C12O enzyme activity was detected. As showed, the C12O enzyme activity of the two groups both increased, and the maximum was reached at 48 hours (5.79 ± 0.32 U, 7.07 ± 0.27 U). After 48 h, the C12O enzyme activity decreased. The trend of C12O enzyme activity in the two groups was similar, but the activity of Zn(II) added group was higher than that of the blank group. It can be seen from the slope of the curve that the enzyme activity growth rate of the blank group was slower than that of the system with Zn(II) added. The possible reason is that in the Zn(II) addition group, C12O is easier to bind to the substrate, so that the strain can obtain the energy required for growth more quickly. A more precise conclusion requires further scanning of the structure of the binding of C12O and the substrate. The promotion of activity and growth of strains in the presence of Zn(II) was further confirmed by the activity increasement of C12O enzyme.
3.3.3. EPS surface potential analysis
Zeta potential is an effective means to report the difference in surface charge of strains, which may be related to polysaccharides/proteins [Citation40,Citation41]. Due to the change of biological growth cycle and the change of adsorbate concentration, the zeta potential () of the strain EPS showed a certain fluctuation within 72 h. In the Zn(II) group, the zeta potential first decreased and then increased. Due to the addition of Zn(II), the potential within 24 h was higher than the blank. At 48 h, the zeta potential reached −20.73 mV. There may be two reasons. On the one hand, the adsorption of Zn(II) by the EPS at 48 h led to a decrease in the cations of the solution; on the other hand, the strains were negatively charged and Zn(II) enhanced the growth of the strain, increasing the potential value [Citation42]. In addition, the mutual attraction of microorganisms was greater than the repulsive force, and mutual aggregation was also conducive to the growth of strains. The Zeta potential value decreased after 48 h and the final potential value decreased due to the decay of the strain.
3.3.4. The interface effect of Zn(II) and EPS
The main components of EPS include polysaccharides and proteins. Proteins are hydrophobic substances and polysaccharides are hydrophilic substances [Citation43]. The Fourier transform infrared spectrum () shows that there are abundant functional groups on the EPS surface. In the fingerprint area, at the wave number of 900 ~ 600 cm−1, there are mainly in-plane rocking vibrations of methylene groups and in-plane vibrations of meta-substituted benzene rings (828 cm−1); at 1300 ~ 900 cm−1, there are mainly two groups of absorption peaks with C = O (1240 ~ 1160 cm−1: antisymmetric; 1160 ~ 1050 cm−1 is symmetric); C = C exists at 976 cm−1; at 1230 cm−1, there are also weaker acid halide functional groups. In the functional group region, at 1500 ~ 1300 cm−1, there are the bending vibration of methyl group (near 1385 cm−1) and the shear bending vibration of methylene group (near 1460 cm−1); at 2000 ~ 1500 cm−1 and 1535 cm−1, there is Amide-I amide from the protein, and the peak shape is wider; at 1670 cm−1, there is Amide-II amide. Compared with the free protein, Amide-I, Amide-II both showed up shifting [Citation44]; at 1734 cm−1, there is -COOH; at 2500 ~ 2000 cm−1, the peak is relatively strong and sharp, indicating that is C ≡ N; at 4000 ~ 2500 cm−1, 2965 cm−1 and 2933 cm−1, there are antisymmetric vibrations of methyl and methylene respectively; at 3400 ~ 3200 cm−1, the hydrogen bond causes the wavenumber to shift to low frequency and produces a very broad hydroxyl absorption peak [Citation45]. The result shows that there are many kinds of functional groups on the surface of EPS through Fourier infrared spectroscopy analysis.
In the Zn(II) added group, C ≡ N, C = O, C = C, acid halide and aromatic ring in EPS changed significantly. The shape of the C ≡ N peak becomes wider and the peak becomes shorter, indicating that C ≡ N in the sample is combined with metal ions. In the fingerprint area and functional group area, C = O is used as an acidic site [Citation46] and the peak shape has changed significantly, indicating that the surface of EPS can provide sites for bonding with Zn(II).
The EPS high-resolution x-ray photoelectron spectroscopy is shown in . The presence of some special functional groups on the surface of the EPS was confirmed by x-ray photoelectron spectroscopy analysis. As shown in , 284.7 eV corresponds to the characteristic peak of aromatic carbon, which is suspected to be m-dichlorobenzene or intermediate product on the surface of the extracellular polymer [Citation2]. The characteristic peak corresponding to 286.1 eV is C-OH, and the characteristic peak assigned to 287.7 eV is C = O, indicating that there are high concentrations of hydroxyl and carboxyl groups on the surface of the extracellular polymer, and the types of functional groups are abundant. The above functional groups are the main sites for the interaction between the EPS surface and the metal, which also proves the results that the surface of EPS can provide sites for bonding with Zn(II). is the high-resolution Zn 2p x-ray photoelectron spectroscopy energy spectrum of EPS. In , 1025.4 eV is (-COO)2Zn under the Zn2p3/2 orbital. It shows that after adding Zn(II), the carboxyl groups on the EPS surface of the strain combined with Zn(II), and during the interfacial interaction, Zn(II) was fixed to the surface of the EPS by the carboxyl groups. 1043.0 eV is the oxidation state of Zn, ZnO or Zn(OH)2 under the Zn2p1/2 orbital [Citation47], indicating that a large amount of oxygen participates in the interface between the EPS surface and Zn(II) during the growth of the strains.
4. Conclusion
The addition of Zn(II) can significantly promote the growth of strains in BTF. When the intake air concentration is 505.74 mg/m3 and the EBRT is 90 s, the removal efficiency of BTF2 is 86.15%. The removal rate of BTF2 is 3.20% higher than that of BTF1. Through the study of the chemical composition, adsorption performance and surface functional groups of the extracellular polymer of the strain, the enhancement mechanism of the bio-accelerator is analyzed. The higher the content of polysaccharide and protein in the extracellular polymer, the better the growth of the strain. At 48 h, the strain was in the logarithmic phase and grew vigorously. Compared with the blank, the polysaccharide content and protein content of the strain added with Zn(II) were respectively increased 5.89 ± 1.98 mg/L, 6.36 ± 0.33 mg/L. The C12O enzyme activity in the added Zn(II) system increased to the maximum value of 7.07 ± 0.27 U within 48 hours, which was an increase of 1.28 U compared to the blank system, indicating that the presence of Zn(II) promoted the activity and growth of the strains. The Zeta potential shows the variability of the EPS surface properties. At 48 h, the Zeta potential with Zn(II) added can reach up to −20.73 mV. The results of the Fourier transform infrared spectrum and x-ray photoelectron spectrum showed that the carboxyl groups on the surface of the extracellular polymer formed a bond with Zn(II), which promoted the growth of strains.
Supplemental Material
Download MS Word (1 MB)Disclosure statement
No potential conflict of interest was reported by the author(s).
Supplementary material
Supplemental data for this article can be accessed here.
Additional information
Funding
References
- Erqi N, Zheng G, Gao D, et al. Emission characteristics of VOCs and potential ozone formation from a full-scale sewage sludge composting plant[J]. Science of Total Environment. 2019;659:664–672.
- Yang B, Sun Z, Wang L, et al. Kinetic analysis and degradation pathway for m-dichlorobenzene removal by brevibacillus agri DH-1 and its performance in a biotrickling filter[J]. Bioresour Technol. 2017;231:19–25.
- Wang T, Li Q, Lv Y, et al. Characteristics and countermeasures of volatile organic compounds emission in chin[J]. Environ Sci. 2013;12:4756–4763.
- Qiao W, Luo F, Lomheim L, et al. Natural attenuation and anaerobic benzene detoxification processes at a chlorobenzene-contaminated industrial site inferred from field investigations and microcosm studies[J]. Environ Sci Technol. 2017;52(1):22–31.
- Wan X, Guo C, Liu Y, et al. Kinetic research on dechlorinating dichlorobenzene in aqueous system by nano-scale nickel/iron loaded with CMC/NFC hydrogel[J]. Chemosphere. 2017;194:297–305.
- Liu J. Application on potential of biosurfactants in hydrophobic VOCs biodegradation [J]. China Sciencepaper. 2014;9:355–359.
- Zhou Q, Zhang L, Chen J, et al. Performance and microbial analysis of two different inocula for the removal of chlorobenzene in biotrickling filters[J]. Chem Eng J. 2016;284:174–181.
- Dewidar A, Sorial G. Effect of surfactin on removal of semi-volatile organic compound: emphasis on enhanced biofiltration performance[J]. Environ Res. 2020;193:110532.
- Zhang Y, Yu K, Oshita K, et al. Economic assessment of biogas purification systems for removal of both H2S and siloxane from biogas[J]. Renewable Energy. 2021;168:119–130.
- Kalantar M, Zamir S, Ferdowsi M, et al. Removal of toluene in a biotrickling filter in the presence of methanol vapors: experimental study, mathematical modeling, and kinetic parameters optimization[J]. J Environ Chem Eng. 2020;9(1):104617.
- Sun Z, Yang B, Wang L, et al. Toluene-styrene secondary acclimation improved the styrene removal ability of biotrickling filter[J]. Chem Speciation Bioavailability. 2017;29(1):54–59.
- Aguirre A, Gentina JC, Malhautier L, et al. Characterization of the microbial community in a biotrickling filter treating a complex mixture of gaseous compounds causing odor nuisance[J]. J Chem Technol Biot. 2021(6). DOI:https://doi.org/10.1002/jctb.6697.
- Kennes C, Veiga MC. Air pollution prevention and control: bireactors and bioenergy[M]. Weinheim: Wiley-Blackwell; 2013.
- Devinny J, Deshusses M, Webster T, et al. Biofiltration for air pollution control[M]. China Resources Press;2017.
- Chen H, Liu S. Cooperative adsorption based kinetics for dichlorobenzene dechlorination over Pd/Fe bimetal[J]. Chem Eng Sci. 2015;138:510–515.
- Wang D, Yan L, Ma X, et al. Ultrasound promotes enzymatic reactions by acting on different targets: enzymes, substrates and enzymatic reaction systems[J]. Int J Biol Macromol. 2018;119:453–461.
- Ding P, Song W, Yang Z, et al. Influence of Zn(II) stress-induction on component variation and sorption performance of extracellular polymeric substances (EPS) from bacillus vallismortis[J]. Bioprocess & Biosystems Engineering. 2018;41(6):781–791.
- Chen Y, Zhang X, Gu J, et al. Effects of Calcium Ion and Zinc Ion on the mycelium growth of flammulina velutipes[J]. Anhui Agricultural Science. 2020;48(4):43–45.
- Liu N, Li D, Li K, et al. Enhanced biodegradation of chlorobenzene via combined Fe3+ and Zn2+ based on rhamnolipid solubilisation[J]. J Environ Sci. 2021;103:108–118.
- Wang L, Yang C, Cheng V, et al. Effects of surfactant and Zn (II) at various concentrations on microbial activity and ethylbenzene removal in biotricking filter[J]. Chemosphere. 2013;93(11):2909–2913.
- Mei Y, Cheng Z, Wang J, et al. Removal of mixed waste gases by a biotrickling filter packed with a novel combined packing material[J]. Huanjing Kexue. 2015;36(12):4389–4395.
- Cheng Y, He H, Yang C, et al. Effects of anionic surfactant on n-hexane removal in biofilters[J]. Chemosphere. 2016;150:248–253.
- Dobrowolski A, Mitua P, Rymowicz W, et al. Efficient conversion of crude glycerol from various industrial wastes into single cell oil by yeast Yarrowia lipolytica[J]. Bioresour Technol. 2016;207:237–243.
- Chrzanowski L, Dziadas M, Lawniczak L, et al. Biodegradation of rhamnolipids in liquid cultures: effect of biosurfactant dissipation on diesel fuel/B20 blend biodegradation efficiency and bacterial community composition[J]. Bioresour Technol. 2012;111:328–335.
- Chen Y, Zhang P, Guo J, et al. Functional groups characteristics of EPS in biofilm growing on different carriers[J]. Chemosphere. 2013;92(6):633–638.
- Lazarova V, Manem J. Biofilm characterization and activity analysis in water and wastewater treatment[J]. Water Res. 1995;29(10):2227–2245.
- Abbasnezhad H, Gray M, Foght J, et al. Two different mechanisms for adhesion of gram-negative bacterium, pseudomonas fluorescens LP6a, to an oil-water interface[J]. Colloids & Surfaces B-Biointerfaces. 2008;62(1):36–41.
- Wang S, Cheng S, Xiao X, et al. Effect of metal ions in wastewater on microbial activity of activated sludge[J]. Journal of Shaanxi University of Science﹠Technology. 2018;36:23–41.
- Xu J, Zhang Z, Chen Q, et al. The short- and long-term effects of Mn2+ on biogranule-based anaerobic ammonium oxidation (anammox)[J]. Bioresour Technol. 2017;241:750.
- Wiens J, Vasil A, Schurr M, et al. Iron-regulated expression of Alginate production, mucoid phenotype, and biofilm formation by pseudomonas aeruginosa[J]. Mbio. 2014;5(1):e01010–13.
- Hao T. Experimental study on SO2 removal by bio-trickling[D]. Xi’an University of Architecture and Technology; 2015.
- Zhang C, Du L, Lu Y, et al. Enhancement effect of additives on treatment of high temperature benzene series gases by biotrickling filter[J]. Modern Chemical Industry. 2018;38:107–110.
- Xia G, Zhou X, Hu J, et al. Simultaneous removal of carbon disulfide and hydrogen sulfide from viscose fibre waste gas with a biotrickling filter in pilot scale[J]. J Clean Prod. 2019;230:21–28.
- Zhang C, Du L, Lu Y, et al. Enhancement effect of additives on treatment of high temperature benzene series gases by biotrickling filter[J]. Modern Chemical Industry. 2018;38:107–110.
- Lebrero R, Rodríguez E, Estrada J, et al. Odor abatement in biotrickling filters: effect of the EBRT on methyl mercaptan and hydrophobic VOCs removal[J]. Bioresour Technol. 2012;109:38–45.
- He Q, Yao K. Impact of alternative electron acceptors on selenium(IV) reduction by anaeromyxobacter dehalogenans[J]. Bioresour Technol. 2011;102(3):3578–3580.
- Soudi M, Ghazvini P, Khajeh K, et al. Bioprocessing of seleno-oxyanions and tellurite in a novel bacillus sp strain STG-83: a solution to removal of toxic oxyanions in presence of nitrate[J]. J Hazard Mater. 2009;165(1–3):71–77.
- Xu B, Xiong C, Deng M, et al. Genetic diversity of catechol 1,2-dioxygenase in the fecal microbial metagenome[J]. J Basic Microbiol. 2017;57(10):883–895.
- Han L, Liu P, Sun J, et al. Engineering catechol 1,2-dioxygenase by design for improving the performance of the cis, cis-muconic acid synthetic pathway in escherichia coli[J]. Sci Rep. 2015;5(1):13435.
- Lavaisse L, Hollmann A, Nazareno M, et al. Zeta potential changes of saccharomyces cerevisiae during fermentative and respiratory cycles[J]. Colloids Surf B Biointerfaces. 2019;174:63–69.
- Li Z, Lu P, Zhang D, et al. Population balance modeling of activated sludge flocculation: investigating the influence of extracellular polymeric substances (EPS) content and zeta potential on flocculation dynamics[J]. Separation and Purfication Technology. 2016;162:91–100.
- Priyakshree B, Najrul H, Gitashree D, et al. Adhesion of gram-negative bacteria onto α-Al2O3, nanoparticles: a study of surface behaviour and interaction mechanism[J]. J Environ Chem Eng. 2018;6(4):3933–3941.
- Huang X, Xu Y, Liu C, et al. A review on the interactions between engineered nanoparticles with extracellular and intracellular polymeric substances from wastewater treatment aggregates[J]. Chemosphere. 2019;219:766–783.
- Sankhla A, Sharma R, Yadav R, et al. Biosynthesis and characterization of cadmium sulfide nanoparticles–an emphasis of ζ potential behavior due to capping[J]. Mater Chem Phys. 2016;170:44–51.
- Nikaido H, Vaara M. Molecular basis of the permeability of bacterial outer membrane[J]. Microbiol Rev. 1985;49:1–32.
- Ramrakhiani L, Ghosh S, Mandal A, et al. Utilization of multi-metal laden spent biosorbent for removal of glyphosate herbicide from aqueous solution and its mechanism elucidation[J]. Chem Eng J. 2019;361:1063–1077.
- Morozov I, Belousova O, Ortega D, et al. Structural, optical, XPS and magnetic properties of Zn particles capped by ZnO nanoparticles[J]. J Alloys Compd. 2015;633:237–245.