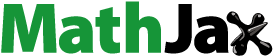
ABSTRACT
The presence of different soils composition complicates the transport behaviors of Cd(II) and thus makes it challenging to predict Cd(II) fate and transport. In this study, montmorillonite modified sand, humic acid modified sand and ferrihydrite modified sand were used as medium to explore the effect of modified sand content, pH and ionic strength (IS) on Cd(II) transport. The results revealed that the adsorption capacity of modified sand for Cd(II) was montmorillonite-coated sand>humic acid-coated sand>ferrihydrite-coated sand>quartz sand. While the increase of the coated sand content limited the transport ability of Cd(II) in sand columns. In addition, high IS and low pH promoted the mobility of Cd(II). The specific surface area, surface charge and adsorption sites of coated sand were the factors affecting the transport of Cd(II). This work provides new information on the transport and spread of Cd(II) in the soil and thus to better prevent Cd(II) from polluting the environment.
1. Introduction
Cadmium (Cd(II)) contamination of the environment has received worldwide attention. It is a nonessential element to most organisms that is highly toxic at relatively low tissue concentrations. It is widely distributed in the earth’s crust with an average concentration of about 0.1 mg kg−1 [Citation1]; yet, industrial and agricultural activities have increased its concentrations across many diverse areas [Citation2]. For example, mining activities, open dumping of unsorted wastes et al., as an important source of heavy metal pollution, give rise to acid drainage containing several hazardous heavy metals, such as lead, cadmium and chromium that easily infiltrate to groundwater [Citation3,Citation4]. At present, most researches focus on the removal of heavy metals in wastewater, especially looking for materials with high adsorption performance [Citation5–7]. However, Cd(II) can persist in the environment for long periods of time but also has the potential to be mobilized and transported between environmental compartments. Therefore, the study of soil Cd(II) pollution has become of great interest in the field of environmental protection in recent years [Citation8,Citation9]. Understanding how metals may be mobilized and subsequently transported from contaminated soils to different receiving environments is of particular interest. However, this is complicated by the naturally heterogeneous biogeochemistry of soils. Transport studies that use model porous media can help overcome some of this complexity, while still providing valuable information about key factors that govern the mobility of Cd(II).
Quartz sand has often been used to simulate porous media in model leaching studies [Citation10]; however, the unique structure, grain size distribution, particle shape, surface charge and mineral surface type confound the transfer of understanding to most soils where Cd(II) mobilization may be a problem [Citation11]. Some of this difficulty arises from the absence of clay minerals, organic matter and iron oxide, which are among the most reactive solid components in soil and sediments and can thus play an important role in the process of transport and fixation of pollutants [Citation12].
Clay particles, humic acid (HA) and ferrihydrite are the most common colloids in soil. Clay particles are widely distributed in subsurface environments and can provide excellent self-purification capability to heavy metal contaminated soils [Citation13]. Clay particles can bind heavy metals effectively on account of their large specific surface areas and net negative surface charges under the most commonly found soil pH. Clay particles can also facilitate the transport of contaminants through their ability to adsorb and transport contaminants in soil and compete with surface retention sites [Citation14]. HA is a macromolecular organic substance that can also play an important role in affecting the transport of pollutants in the environment. Functional groups in HA, such as carboxylic acid and phenolic groups, and its high surface area make it highly reactive to heavy metals. HA has been shown to improve the electrostatic and steric repulsion between nanoparticles and porous medium [Citation15]. Compared to other iron minerals, ferrihydrite has a strong adsorption capacity for Cd(II) due to its amorphous surface properties and the larger specific surface area [Citation16] [Citation17]., found that iron oxide colloids could promote the mobility of Cu2+ by providing a transport vector for the sorbed metal.
It has been reported that soil components were fixed on quartz sand to perform column transport experiments. Experiments studying the transport of heavy metals in iron oxide-coated sand have suggested that while the surface area of the coated sand is larger than that of uncoated quartz sand, the transport of heavy metals is increased in the coated sand [Citation18,Citation19]. Similarly, there is also HA fixed on the surface of quartz sand for nitrate tracer migration experiments [Citation20]. Subsequently, [Citation21] showed that by modifying the surface properties of kaolinite with goethite–HA, the cation exchange capacity increased to five times that of the kaolinite, with a simultaneous increase in the adsorption capacity for heavy metals.
These studies have shown that the modified quartz sand using soil components will affect the adsorption and transport behavior of pollutants in the medium. However, the accurately describe the extent to which heterogeneous media content promotes or inhibits heavy metal migration behavior has not yet been investigated. It is important that the difference in soil composition determines the migration and transformation of heavy metals in the soil. At the same time, changes in the soil environment not only control the surface properties of the soil particles but also change the shape of heavy metal ions, thereby changing the ability of the soil components to fix heavy metal ions. Therefore, the transport behavior of Cd(II) in the soil affected by soil components should be fully understood.
In this study, the effect of coated sand on the transport of Cd(II) was investigated by a series of adsorption and column experiments. The objectives of this study were to (1) compare the transport of Cd(II) in different types of coated sand; (2) study the effect of coated sand content on Cd(II) transport; and (3) test the effects of environmental change on Cd(II) transport in the coated sand.
2. Materials and methods
2.1 Materials
CdCl2(5/2H2O) was purchased from Aladdin industry corporation, Shanghai, China. The montmorillonite and humic acid (HA) was purchased from Tianjin Guangfu Fine Chemical Research Institute, Tianjin, China. Quart sand (Shanxi Zhouzhi Country Quartz Sand Company, China) was used in this study as the porous medium with size ranges: 0.4–0.8 mm.
The modification of the sand with HA followed previously established procedures [Citation22]. Prior to that, the HA was purified according to Yang’s method [Citation23]. The clean quartz sand was mixed with the HA solution (30 mg L−1) in a beaker for 24 h, after which 1.0 M HCl was added dropwise to the mixture while stirring to ensure optimal reaction. The quartz sand was then removed and dried at 100°C. The dry modified sand was then cleaned using 0.001 M NaCl and HCl solutions until the wash solution was free of impurities. Finally, the cleaned HA-modified sand was washed using DI water and dried at 105°C.
Prior to treating the sand with montmorillonite ((Na,Ca)0.33(Al,Mg)2[Si4O10](OH)2·nH2O), the organic matter was removed from the clay using H2O2 (30%), after which the clay was dispersed and fractionated to obtain particles with a hydrodynamic diameter <2 μm using gravity sedimentation. The supernatant was thoroughly mixed with the quartz sand and 50 mg L−1 polyacrylamide, then dried at 100°C for 24 h. Finally, the montmorillonite coated sand (forthwith, ‘M-coated sand’) was washed with DI water to remove all unattached polyacrylamide and dried at 100°C for 24 h [Citation20].
Ferrihydrite was prepared by preparing a 500 mg L−1 ferrous nitrate (as Fe(NO3)3 · 9H2O) solution and adjusting its pH to 7.5 by adding 1.0 M KOH. The ferrihydrite precipitated in the process. The ferrihydrite suspension was centrifuged at 3000 r min−1 for 10 min, after which the residue was suspended in 1 L of DI water to get 10 g L−1 of ferrihydrite suspension. 40 mL of the ferrihydrite suspension was mixed with 100 g quartz sand and shaken for three days. Finally, the ferrihydrite-coated sand (forthwith, ‘FH-coated sand’) was washed three times sequentially with 1.0 M HNO3, 1.0 M NaOH and then DI water [Citation19].
A scanning electron microscale and energy-dispersive spectrometer (SEM-EDS) was used to investigate the elemental composition and surface morphology of the coated quartz sands. The Zeta potential of the sands was determined using a Zeta-Plus analyzer and a method described in an earlier study [Citation22]. Briefly, the sand was sonicated for 10 min and soaked in background solution for 6 h; then, the Zeta potential of the solution was measured as the zeta potential of the sand in solution (shown in ).
Table 1. The Zeta potential of coated quartz sand
2.2 Batch experiment
The adsorption of Cd(II) onto the different types of modified sand was tested through two sets of batch experiments. (1) Kinetic adsorption experiment: 10 g of each of the modified sands and the unmodified control were equilibrated with 25 mL of the Cd(II) spike solution (CCd = 10 mg L−1) in separate 50 mL centrifuge tubes. The solution pHs were adjusted to 6 ± 0.2 using 0.01 M HCl and 0.01 M NaOH, after which the mixtures equilibrated in a rotating incubator shaker (180 r min−1 at 25°C) for intervals of 5, 10, 20, 40, 60, 120, 240, 480, 720, and 1440 min. (2) Isothermal adsorption experiment: the sorption isotherms were determined by equilibrating 10 g of the modified sands and the control with 25 mL of spike solutions with different Cd(II) concentrations (0.5, 1, 2, 4, 7, 10, 15 and 20 mg L−1) in 50 mL centrifuge tubes. Each mixture was equilibrated as in the first batch for 24 h. The supernatants were filtered through a 0.22 μm filter, after which the Cd(II) concentrations in the filtrate were measured by flame atomic absorption spectrometry (Z-2000, Hitachi, Tokyo, Japan). All tests were performed in duplicate.
The sorption kinetics were fitted to pseudo-first-order, pseudo-second-order, Elovich and two-constant equation models [Citation24], while the sorption isotherms were also described by Langmuir, Freundlich and Temkin models [Citation25]. The removal efficiency of Cd(II) by the modified sands and the control was expressed using Equationequation 1(1)
(1) :
Where, QE is the amount of Cd(II) adsorbed on the sand (mg g−1), m is the mass of the sand (g), V is the volume of the solution (ml), and c0 and ce are the initial and post-equilibration solution concentrations of Cd(II) (mg L−1), respectively.
2.3. Column transport experiments
A 15.0 cm long plexiglass column with an internal diameter of 3.0 cm was used for the column experiments. Before the experiment, the quartz sand was washed sequentially with 0.1 M NaOH and HCl solutions, after which it was rinsed with DI water until there were no visible impurities left [Citation26]. Approximately 160 g of the modified medium was wet-packed into the columns with deionized (DI) water using a glass stirring rod to ensure constant bulk density throughout, while removing trapped air in the column [Citation27]. The porosity of the media in the columns was 0.45, resulting in a total pore volume (PV) of 50 mL.
The experiment consisted of two parts. In the first part, quartz sand was evenly mixed with different proportions of montmorillonite (M) coated quartz sand (0, 5%, and 10%), HA (HA) coated quartz sand (0, 10%, and 30%) and ferrihydrite (FH) coated quartz sand (0, 10%, and 30%). The columns were initially leached with DI water for 3–4 h, until the effluent was free of visible impurities. The column was then flushed with a background electrolyte solution (pH 6, ionic strength (IS): 0.001 M, NaCl). Subsequently, 4 PVs of the 20 mg L−1 Cd(II) solution with the same pH and IS were pumped into the column from the bottom with a peristaltic pump. Then, the 4PVs background electrolyte solution was pumped into the column. Samples at the top-column outlet were collected in glass vials every 10 mL with an automatic fraction collector. The controlled flow rate was set to 1 ml min−1. The results were used to establish the optimal proportions for Cd(II).
In the second part of the experiment, the column was packed with the M-coated quartz sand (5%), HA-coated quartz sand (30%), and FH-coated quartz sand (30%). The experiment was repeated as a multifactorial experiment testing different combinations of pH (4, 6 and 8) and IS (0.1, 0.01 and 0.001 M). The pH of the injected solution was adjusted to 0.1 M HCl and 0.1 M NaOH, and the IS was modified using NaCl. The effluent Cd(II) concentrations were analyzed as described above. 10 mg L−1 of NaNO3 was used as the tracer solution to determine the dispersion coefficient of each column at the established flow velocity. The effluent NO3− concentration was measured at 201 nm by UV-visible spectrophotometry [Citation26]. All column experiments were repeated in duplicate.
2.4. Instrument information
In this study, the model of the flame atomic absorption spectrometer is Z-2000 of Hitachi, Tokyo, Japan; the model of scanning electron microscale and energy-dispersive spectrometer is S-4800 of Hitachi, Japan; the model of the Zeta-Plus analyzer is Zetasizer nano ZS90 of Malvern Instruments, UK; the model of the rotating incubator shaker is HZQ-F100 of Donglian Electron Technology Ltd., Harbin, China; the model of the peristaltic pump is HL-2B of Shanghai Huxi Analysis Instrument Factory Co., Ltd., China; the model of the automatic fraction collector is BS-16A-LCD(II) of Shanghai Huxi Analysis Instrument Factory Co., Ltd., China; the model of the UV-visible spectrophotometry is of UV-2800, Unico, USA.
2.5. Mathematical model
The advection-dispersion-reaction (ADR) model was employed to simulate Cd(II) transport to explain the retention of Cd(II) in modified sand columns. The mathematical equation of the model follows [Citation28] (EquationEq. 2)(2)
(2) :
Where R is the retardation factor, which reflects the equilibrium reaction in quartz sand column; Cw is the concentration of Cd(II) in pore water (mg L−1); D is the dispersion coefficient (cm2 min−1); z is the transport distance (cm); v is the flow rate of pore water (cm min−1); and k is the irreversible, first-order kinetic reaction rate constant (min−1), and Smax is the maximum deposited particle concentration. The parameters k and Smax were established using HYDRUS-1D, while the recovery values of the Cd(II) were measured with a mass balance
3. Results and discussion
3.1. Characterization of coated sands
As shown by the surface morphology of the coated sands, the surface of the quartz sand was very smooth, upon which the montmorillonite created an incomplete, relatively rough, irregular laminar cover (). The HA coating resulted in a smooth sheet and the ferrihydrite was more amorphous and rougher than the HA. These results were consistent with those presented by [Citation20]. The EDX spectra showed how the coatings changed the elemental composition of the surfaces: montmorillonite increased the amount of Al, Mg and Ca, HA added C and O and ferrihydrite increased the abundance of Fe ().
3.2. Kinetic adsorption and adsorption equilibrium test
The kinetic adsorption fitting curves of quartz sand, M-coated, HA-coated and FH-coated sand to Cd(II) are shown in . It could be seen from the figure that they all showed a rapid adsorption process at the initial stage, which indicated that the adsorption sites on the surface of heterogeneous media were quickly occupied by Cd(II). Then, the adsorption rate gradually slowed down and entered the slow adsorption stage, and finally the reaction reached dynamic equilibrium. The pseudo-first-order, pseudo-second-order, two-constant and Elovich equation models were used to simulate the kinetics adsorption of Cd(II) on coated sands. The adsorption kinetics that achieved the optimal fits for the different models to the experimental data on the coated sands are shown in . The kinetics data showed that the pseudo-second-order model was best fitted for the kinetics adsorption of Cd(II) on coated sands. The R2 of M-coated, HA-coated, FH-coated and quartz sand was 0.9999, 0.9809, 0.9933, and 0.9998, respectively, which suggested that Cd(II) adsorbs onto the coatings through chemisorption (i.e. electron exchange or sharing) [Citation29].
Table 2. Constants and correlation coefficients of kinetic models of Cd(II) adsorption on quartz sand with different coatings
Figure 2. Kinetic curves and Isothermal curves and fitted models of Cd(II) in modified media under experimental conditions, pH = 6.5, IS = 0.001 mol L−1, NaCl. (a: quartz sand; b: M-coated sand; c: HA-coated sand; d: FH-coated sand)
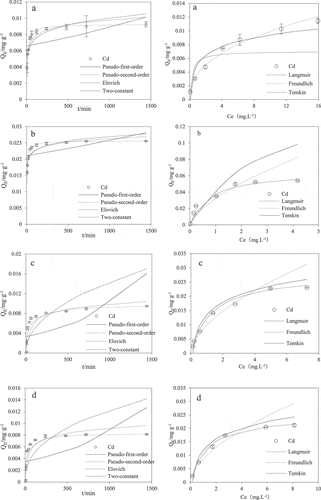
As shown in , the maximum adsorption of Cd(II) by quartz sand, M-coated, HA-coated, and FH-coated sand increased with the increase in the initial concentration of the Cd(II) solution. When the Cd(II) concentration reached a certain concentration, the upward trend of Cd(II) adsorption on the medium gradually slowed down, and the reaction gradually reached equilibrium. Afterward, the Langmuir, Freundlich and Temkin models were used to fit the adsorption data. Comparing the linear correlation coefficient (R2), it could be found that the Langmuir model had the best fitting effect for Cd(II) adsorption by modified quartz sand (). The R2 of M-coated, HA-coated and FH-coated sand were 0.9848, 0.9915, and 0.9975, respectively, thereby suggesting that monolayer molecules were adsorbed [Citation25]. The maximum adsorption capacity of the quartz sand was 0.007 mg g−1, which was improved considerably through coating by HA (0.028 mg g−1), ferrihydrite (0.025 mg g−1) and montmorillonite (0.0649 mg g−1). This is mainly because the coating increases the surface area of the quartz sand and also increases the functional groups on the surface of the quartz sand, thereby increasing the amount of Cd[II) absorbed by the coated quartz sand. The study of [Citation22] also showed that the adsorption of heavy metals on montmorillonite was the largest due to the largest specific surface area, followed by HA and ferrihydrite. The SEM results gave qualitative evidence, and [Citation22] also suggested that using these materials would alter the surface change and specific Cd(II] binding properties of the quartz sand. However, the quartz sand was well fitted by the Freundlich model. It may be that the uneven charge distribution on the surface of the quartz sand causes the uncharged areas on the surface of the quartz sand to adsorb Cd(II) through van der Waals force, which makes the adsorption tend to be adsorbed in multiple layers [Citation25].
Table 3. Constants and correlation coefficients of isotherm models of Cd(II) adsorption on quartz sand with different coatings
3.3. Effects of coated sands content on Cd(II) transport in sand columns
The experimental outflow Cd(II) breakthrough curves showed the retention of the metal in the sand columns and changes in the effluent concentration as the content of M-coated, HA-coated, FH-coated sand in the columns was modified (5, 10 and 30%) (). Generally, the retention rate of Cd(II) in modified sand column increased with the increase in coated sand content, while the transport capacity of Cd(II) was opposite. The breakthrough curves also showed the differences in the Cd(II) transport dynamics as the modified sand content was changed. The results clearly showed that Cd(II) mobility was reduced in the presence of the modified sands. This is likely to be achieved by higher specific surface areas, and the functional groups such as -OH and -COOH on the surface of the montmorillonite, HA and ferrihydrite also provide sites for Cd(II) adsorption, which resulted in greater Cd(II) ion adsorption [Citation20,Citation30,Citation31]. The peak breakthrough in the quartz sand only column was seen at 1.6 PV, while this was delayed until 4.8 PV equivalent of water had passed through the columns with 5% or 10% M-coated sand content. This is mainly because the montmorillonite could sorb heavy metals strongly on account of its cation exchange properties [Citation32]; hence, the multilayer surface of M-coated quartz sand achieved the greatest influence on cadmium transport.
Figure 3. Breakthrough curves of Cd(II) in four kinds of sand columns under different amounts of coated sand, pH = 6, IS = 0.001 M (a: quartz sand; b: M-coated sand; c: HA-coated sand; d: FH-coated sand)
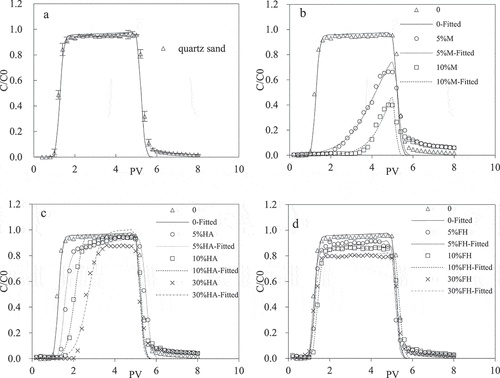
Moreover, the peak concentration in breakthrough curves decreased with increasing M-coated sand content, highlighting the greater retention of Cd(II) as the amount of coating in each column increased. A mass balance calculation found the recovery rate of Cd(II) to be 100.22, 37.45 and 18.59% in the 0, 5 and 10% M-coated sand columns, respectively (). The same general transport trends were seen in the HA-coated and FH-coated sand columns, although the effects of the modified sand content were not as great as with the M-coated column. This was reflected by the higher peak concentrations in the respective breakthrough curves than seen for the M-coated sand () and lower amounts of Cd(II) retained by the columns, for example: in the three columns with 10% coated sand content, more Cd(II) was retained by the M-coated sand than by HA- and FH-coated sands (). The negative charges on the phenolic and carboxylic acid functional groups on HA were likely to have increased the overall negative charges on the HA-modified sand, resulting in greater affinity for Cd(II) ion sorption. Ferrihydrite had a net positive charge, and the net surface-negative charge of FH-modified quartz sand was likely to have been less than that of the quartz sand, which helped to explain the relatively low retention of Cd(II) in the FH-coated sand columns [Citation33].
Table 4. The fitting parameters and recovery of Cd(II) in four sand columns under different conditions
The fitted parameters in the ADR model simulations agreed very well with the experimental data from the various columns (R2 > 0.94 for all but one experiment; ). The retention coefficient (k), the maximum deposited concentration (Smax) from the best model fitted and the corresponding R2 values are listed in . The k and Smax values were lowest for quartz sand and increased with the modified sand contents. The differences in these variables between the different substrates mirror the differences in Cd(II) retention rates identified above, where the column with 10% M-modified sand content had the highest k (0.1338 min−1) and Smax (1.0902 mg g−1) values (). The optimal Smax and k values of Cd(II) in coated sand columns, which indicates that the fixed colloid has a retarding effect on the transport of Cd[II). Similar/different values for k and Smax to those seen here have been reported previously by [Citation22].
3.4. Effects of IS on Cd(II] transport through coated sand columns
Previous studies have shown ionic strength of the mobile phase (e.g. soil porewater, groundwater) to be an important factor affecting Cd(II) transport in many porous environments [Citation34–36]. The breakthrough curves of Cd(II) affected by IS in coated-sand column is shown in . The results showed that there was little change in the transport of Cd(II) in the pure quartz sand columns under different ISs () due to the low adsorption capacity of the medium. The effect was more obvious in the modified sand columns where decreasing IS corresponded with enhanced the retention of Cd(II) and consequently lower Cd(II) concentrations in the effluent. It could be seen from that the Zeta potential value was negative, which indicated that the surface of the medium was negatively charged. The Zeta potential increased with the increase of IS, which led to the weakening of the electrostatic attraction between Cd(II) ions and the medium, then the migration of Cd(II) was enhanced. The increasing IS here was also likely resulting in the increased competition for cation exchange sites by Na+, reduction in the activity of the sorbing ions, as well as compression of the electric double layer at the sorbing surfaces [Citation37]. In addition, it was also highly likely that greater complexation for Cd(II) ions by Cl− ions would have contributed to the reduced retention of Cd(II) on the modified sand surfaces at higher ISs [Citation38].
Figure 4. Breakthrough curves of Cd(II) in four kinds of sand columns under three different IS and pH (a: quartz sand; b: 5%M-coated sand; c: 30%HA-coated sand; d: 30%FH-coated sand)
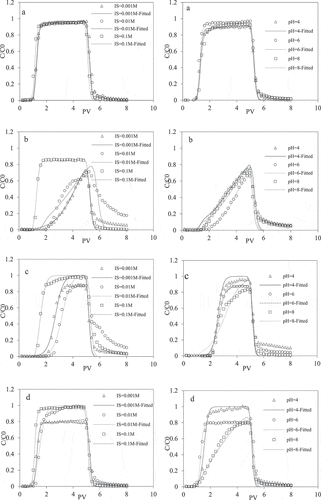
It could be found that under different ISs, montmorillonite had the greatest effect on Cd(II) migration, followed by kaolinite and HA. This is mainly because montmorillonite has strong cation exchange capacity and strong adsorption performance. This was consistent with the results of previous adsorption experiments. The mobility of Cd(II) was relatively high in HA-coated sand columns, mainly because the coated HA was relatively smoother than the surface of clay minerals and had a weak retention capacity for Cd(II). These effects were reflected by the fitting parameters: Smax generally decreased as the IS increased, which was consistent with the experimental results. Likewise, its appeared to result in unexpectedly high values for k in the 5% M, 30% HA and 30% FH-coated sand columns at 0.01 M. The reasons for these are not immediately obvious, which may be due to the poorer model fit for this experiment at 0.01 M (R2 0.7819, 0. 8508, and 0.8913, respectively; ).
3.5. Effects of pH on cadmium transport through coated sand columns
The breakthrough curves of Cd(II) affected by pHs in coated sand column is shown in . It could be found that Cd(II) had a high mobility in quartz sand columns at different pH, and approximately the entire content leaves the sand column. In the quartz sand column, the peak value of the Cd(II) penetration curve was 0.89 at pH = 4, when the pH value increased to 6 and 9, the peak value of the penetration curve increased to 0.95 and 0.92, respectively. It indicated that acidic environment would inhibit the migration of Cd(II), and Cd(II) was easier to migrate under neutral and alkaline conditions. This was because the increase in pH would cause the Zeta potential on the surface of the quartz sand to decrease (), the migration of Cd(II) in the sand column would be suppressed as the pH increased.
The breakthrough curves for Cd(II) in the coated sand columns were significantly influenced by the different pHs (). Increasing pH resulted in enhanced the retention of Cd(II) and a decrease in effluent concentrations. Most of the Cd(II) was recovered from columns subject to the lowest pH treatments, with the 30% FH-coated sand column binding the lowest amount of Cd(II) (7.9% at pH 4; ), while the most Cd(II) was retained by the 5% M-coated sand column (62.55% at pH 6). The fitted ADR models were fitted experimental results relatively well (R2 > 0.949 for all treatments) and the value of Smax was consistent with the Cd(II) recovery calculated independently from the mass balance, showing an increase with increasing pH. These effects were likely to arise at low pH due to increased protonation of Si-O−, Al-O− groups in montmorillonite and competition with Cd(II) for adsorption sites, which would weaken the binding ability of modified quartz sand, resulting in more ions flowing out [Citation39]. The carboxylic acid and phenolic functional groups on HAs were likely to be negatively charged due to proton dissociation at higher pH, allowing them to form stable complexes with the Cd(II) [Citation40]. In addition, the precipitation was formed by combination of OH− and Cd(II) under high pH, and fixed in the sand column resulting in the transport of Cd(II) was decreased. In pure quartz sand columns, the Cd(II) concentration was detected at approximately 0.8 PV, after which the Cd(II) concentration rapidly increased and peaked at 2PV; while the Cd(II) in the outflow in the coated sand column was approximately detected at 1 PV and the peak value was delayed. This is mainly because the coating material has the ability to adsorb Cd(II). According to the previous adsorption experiments, montmorillonite had the strongest adsorption capacity for Cd(II), which was consistent with our migration results.
4. Conclusions
In summary, these were components in many soils and our results demonstrate how they could greatly influence dissolved Cd(II) transport. The adsorption capacity of quartz sand could be significantly increased by modifying it with these montmorillonite, HA, and ferrihydrite. The maximum sorbing capacity of quartz sand increased by 9.2, 3.6, and 4-fold when coated using montmorillonite, ferrihydrite, and HA, respectively. Furthermore, we explored the effects of changes in natural factors (pH and IS) on Cd(II) transport. Cd(II) had the weakest migration ability in M-coated sand columns, followed by HA-coated sand columns and quartz sand columns. The recovery rate of Cd(II) in HA-coated sand columns and FH-coated sand columns decreased with increasing pH. It was beneficial to transport behavior of Cd(II) in the M, HA and FH-coated sand columns at higher IS. In this work, the transport and transformation behavior of Cd(II) in different soil components was beneficial to provide a theoretical basis for the treatment of heavy metal pollution in different soils. Therefore, further studies are needed under unsaturated conditions, instantaneous flow rates and chemical perturbations to demonstrate and quantify the behavior of Cd(II) transport in porous media.
Disclosure statement
No conflict of interest exists in the submission of this manuscript, and the manuscript is approved by all authors for publication. This study did not involve any human subjects. I would like to declare on behalf of my co-authors that the work described was original research that has not been published previously, and not under consideration for publication elsewhere, in whole or in part. All the authors listed have approved the manuscript that is enclosed.
Additional information
Funding
References
- Matei N, Popescu A, Radu GL, et al. Cadmium and lead occurrence in soil and grape from Murfatlar Vineyard. Ovidius University Annals of Chemistry. 2015;26(1):37–40.
- Xiao X, Zhang J, Wang H, et al. Distribution and health risk assessment of potentially toxic elements in soils around coal industrial areas: a global meta-analysis. SciTotal Environ. 2020;713:135292.
- Dong J, Li B, Bao Q. In situ reactive zone with modified Mg(OH)2 for remediation of heavy metal polluted groundwater: immobilization and interaction of Cr(III), Pb(II) and Cd(II). J Contam Hydrol. 2017;199:50–57.
- Kumarasinghe U, Kawamoto K, Saito T, et al. Evaluation of applicability of filling materials in permeable reactive barrier (PRB) system to remediate groundwater contaminated with Cd and Pb at open solid waste dump sites. Process SafEnviron Prot. 2018;120:118–127.
- He H, Xiang Z, Chen X, et al. Biosorption of Cd(II) from synthetic wastewater using dry biofilms from biotrickling filters. Int J Environ Sci Technol. 2018;15(7):1491–1500.
- Huang Y, Yang C, Sun Z, et al. Removal of cadmium and lead from aqueous solutions using nitrilotriacetic acid anhydride modified ligno-cellulosic material. RSC Adv. 2015;5(15):11475–11484.
- Wu M, Liu H, Yang C. Effects of pretreatment methods of wheat straw on adsorption of Cd(II) from waterlogged paddy soil. Int J Environ Res Public Health. 2019;16(2):205.
- Li J, Xu Y. Immobilization remediation of Cd-polluted soil with different water condition. J Environ Manage. 2017;193:607–612.
- Li L, Zhaoli YN. Kinetics and thermodynamics of Cd(II) adsorption on to soil colloids. J Shanxi Agric Univ. 2016;36:786–792.
- Wang Y, Yin X, Sun H, et al. Transport of vanadium (V) in saturated porous media: effects of pH, ionic-strength and clay mineral. Chem Speciation Bioavailability. 2016;28(1–4):7–12.
- Zhang L, Hou L, Wang L, et al. Transport of fullerene nanoparticles (nC60) in saturated sand and sandy soil: controlling factors and modeling. Environ Sci Technol. 2012;46(46):7230–7238.
- Gavrilescu M. The role of colloidal systems. In: Fanun M, editor. Environmental Protection. Amsterdam: Elsevier; 2014. p. 397–451.
- Zhang T, Wang W, Zhao Y, et al. Removal of heavy metals and dyes by clay-based adsorbents: from natural clays to 1D and 2D nano-composites. Chem Eng J. 2021;420:127574.
- Chen H, Gao B, Yang L, et al. Montmorillonite enhanced ciprofloxacin transport in saturated porous media with sorbed ciprofloxacin showing antibiotic activity. J Contam Hydrol. 2015;173:1–7.
- Lv X, Gao B, Sun Y, et al. Effects of humic acid and solution chemistry on the retention and transport of cerium dioxide nanoparticles in saturated porous media. Water Air Soil Pollut. 2014;225:2167–2176.
- Liu J, Zhu R, Liang X, et al. Synergistic adsorption of Cd(II) with sulfate/phosphate on ferrihydrite: an in situ ATR-FTIR/2D-COS study. Chem Geol. 2018;477:12–21.
- Wang D, Paradelo M, Bradford SA, et al. Facilitated transport of Cu with hydroxyapatite nanoparticles in saturated sand: effects of solution ionic strength and composition. Water Res. 2011;45(18):5905–5915.
- Han P, Wang X, Cai L, et al. Transport and retention behaviors of titanium dioxide nanoparticles in iron oxide-coated quartz sand: effects of pH, ionic strength, and humic acid. Colloids Surf A Physicochem Eng Aspects. 2014;454(1):119–127.
- Mähler J, Persson I. Rapid adsorption of arsenic from aqueous solution by ferrihydrite-coated sand and granular ferric hydroxide. Appl Geochem. 2013;37(10):179–189.
- Jerez J, Flury M. Humic acid-, ferrihydrite-, and aluminosilicate-coated sands for column transport experiments. Colloids Surf A Physicochem Eng Aspects. 2006;273(1–3):90–96.
- Unuabonah EI, Olu-Owolabi BI, Adebowale KO. Competitive adsorption of metal ions onto goethite–humic acid-modified kaolinite clay. Int J Environ Sci Technol. 2016;13(4):1043–1054.
- Yin X, Jiang Y, Tan Y, et al. Co-transport of graphene oxide and heavy metal ions in surface-modified porous media. Chemosphere. 2019;218:1–13.
- Yang Y, Koopal LK. Immobilisation of humic acids and binding of nitrophenol to immobilised humics. Colloids Surf A Physicochem Eng Aspects. 1999;151(1–2):201–212.
- Wang J, Guo X. Adsorption kinetic models: physical meanings, applications, and solving methods. J Hazard Mater. 2020b;390:122156.
- Wang J, Guo X. Adsorption isotherm models: classification, physical meaning, application and solving method. Chemosphere. 2020a;258:127279.
- Jiang Y, Zhang X, Yin X, et al. Graphene oxide-facilitated transport of Pb2+ and Cd2+ in saturated porous media. Sci Total Environ. 2018;631-632:369–376.
- Sun H, Gao B, Tian Y, et al. Kaolinite and lead in satu rated porous media: facili tated and impeded tran sport. J Environ Eng. 2010;136(11):1305–1308.
- Hao C, Gao B, Hui L, et al. Effects of pH and ionic strength on sulfamethoxazole and ciprofloxacin transport in saturated porous media. J Contam Hydrol. 2011;126(1):29–36.
- Luo X, Yu L, Wang C, et al. Sorption of vanadium (V) onto natural soil colloids under various solution pH and ionic strength conditions. Chemosphere. 2017;169:609–617.
- Du H, Peacock CL, Chen W, et al. Binding of Cd by ferrihydrite organo-mineral composites: implications for Cd mobility and fate in natural and contaminated environments. Chemosphere. 2018;207:404–412.
- Qu C, Chen W, Fein JB, et al. The role of interfacial reactions in controlling the distribution of Cd within goethite−humic acid−bacteria composites. J Hazard Mater. 2021;405:124081.
- Qu C, Chen W, Hu X, et al. Heavy metal behaviour at mineral-organo interfaces: mechanisms, modelling and influence factors. Environ Int. 2019;131:104995.
- Ma J, Guo H, Lei M, et al. Enhanced transport of ferrihydrite colloid by chain-shaped humic acid colloid in saturated porous media. SciTotal Environ. 2018;621:1581–1591.
- Braun A, Klumpp E, Azzam R, et al. Transport and deposition of stabilized engineered silver nanoparticles in water saturated loamy sand and silty loam. SciTotal Environ. 2015;535:102–112.
- Torkzaban S, Bradford SA, Vanderzalm JL, et al. Colloid release and clogging in porous media: effects of solution ionic strength and flow velocity. J Contam Hydrol. 2015;181:161–171.
- Zhou D, Jiang X, Lu Y, et al. Cotransport of graphene oxide and Cu(II) through saturated porous media. Sci Total Environ. 2016;550:717–726.
- Zhang Y, Zhu C, Liu F, et al. Effects of ionic strength on removal of toxic pollutants from aqueous media with multifarious adsorbents: a review. SciTotal Environ. 2018;646:265–279.
- Tan Y, Yin X, Wang C, et al. Sorption of cadmium onto Mg-Fe Layered Double Hydroxide (LDH)-Kiwi branch biochar. Environ Pollut Bioavailability. 2019;31(1):189–197.
- Altin O, Ozbelge OH, Dogu T. Effect of pH, flow rate and concentration on the sorption of Pb and Cd on montmorillonite: II. Modelling. J Chem Technol Biot. 2015;74(12):1139–1144.
- Wang Y, Li Y, Zhang Y, et al. Effects of macromolecular humic/fulvic acid on Cd(II) adsorption onto reed-derived biochar as compared with tannic acid. Int J Biol Macromol. 2019;134:43–55.