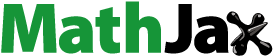
ABSTRACT
At present, the situation of water contamination seriously hinders the healthy cycle of natural environment and the development of human society. Capacitive deionization technology is a novel water treatment technology for remediating inorganic-contaminated ions from aqueous medium. This paper briefly introduces the related theory and reviews the current research and application in removing inorganic contaminants (heavy metal ions, anionic contaminants, other inorganic contaminants, etc.) in the aqueous solution. In addition, the influence of electrode materials, device design and environmental factors on capacitive deionization of inorganic contaminants was briefly summarized. In order to provide help for the further development and application of the capacitive deionization technology, the summary and prospect are also part of the article.
Graphic abstract
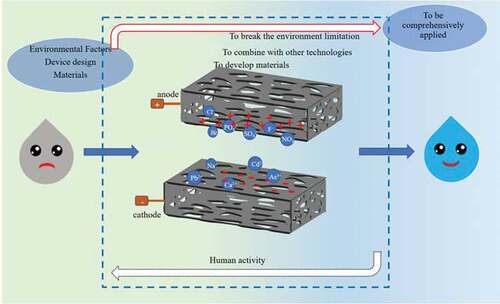
1. Introduction
Fresh water is a necessary, at the same time very scarce, resource for human life and social development. It only accounts for 2.5% of the total water resources, including more than half of the glaciers and other freshwater that cannot be directly used by human beings [Citation1]. In addition, industrial water consumption is increasing with the development of the social economy and which also bring water contamination. Therefore, many countries and regions are suffering from the dilemma of water shortage. And the contradiction of water shortage and pollution are currently urgent problems to be solved.
At present, there are many advanced technologies are used for wastewater treatment, such as physical method [Citation2–4], chemical method [Citation5,Citation6] and biological method [Citation7]. However, these traditional water treatment technologies showed some unsatisfactory aspects in terms of environmental and economic effects, such as secondary pollution, high cost and high energy consumption [Citation8]. Thus, researchers focused their objectives into developing a suited alternative deionization technology for existing technologies which has energy efficiency and environmental friendliness. Capacitive deionization (CDI) belongs to one of the physical adsorption technologies. And as a kind of water treatment technology with no secondary pollution, low energy consumption and little scaling, CDI technology attracts increasing attention and develops rapidly including preparation or modification materials, optimization of operational parameters, integration with other technologies, etc. It possesses a good development prospect in the wastewater treatment.
2. Theory of CDI technology
The theory of CDI is based on the double layer of electrochemistry [Citation9]. In the electrochemical system, there is a double layer at the interface of the electrode and target water. The double layer is capacitive and can be charged and discharged. The charging charge on one side of the electrode is provided by electrons or positive charges on the electrode, while the charging charge on the other side of the solution is provided by cations or anions in the solution [Citation10]. Therefore, when the electrodes are charged with a low voltage, the ions or charged particles in the solution enter the channel formed by the anode and cathode. Then they migrate towards the electrode with opposite polarity under the action of the electric field force (). These ions or charged particles are held in the electrical layer until the discharging step, which the voltage is reversed or removed. With the reverse of the charge, the ions are released from the surface of the electrode, and the double electric layer can be regenerated () [Citation11]. Through the above round, the purification is realized in the target water during the charging and discharging processes [Citation12].
3. Application of CDI technology in removing inorganic-contaminated ions
Nowadays, CDI can be characterized as a new topic. Compared with other water treatment methods, the process of CDI mainly removes the ions from the target contaminated water without the loss of water and the recovery rate of water is higher. In addition, the cost of CDI equipment maintenance is relatively lower and the chemical reagents are free in the post-treatment process to avoid secondary pollution. Therefore, the CDI technology has a broad development prospect with the comprehensive economic benefit and environmental safety. In recent years, a lot of studies on the removal of inorganic contaminating ions by CDI technology were carried out by many researchers.
3.1 Removal of heavy metals
In 1997, Farmer et al. [Citation13] first reported the study on CDI of heavy metal (Cr6+), which opened the research direction of the application of CDI technology in the removal of heavy metals. Until now, CDI-based technologies have been widely used to removal various heavy metal ions from water media. Gaikwad et al. [Citation14] applied CDI technology to simultaneously remove chromium (VI) ions and fluoride ions. They found that the maximum electrosorption capability reached to 97.1% and 94.2% respectively for chromium (VI) ions and fluoride ions when the optimized flow rate was 16 mL min−1 and applied voltage was 1.2 V. Dai et al. [Citation15] studied the electrosorption of As (III) in aqueous solutions by using activated carbon as the electrode. In the electrosorption process, As (III) could be efficiently oxidized to As (V) and then As (V) was accumulated in the EDL of the anode. In addition, some other researches in removing heavy metals also include cadmium [Citation16], lead [Citation17] and copper plasma [Citation18], etc.
3.2 Removal of anionic contaminants
CDI also had significant effect in the removal of anionic contaminants including perchlorate, bromide ions, nitrate, chloride, phosphate and so on [Citation19–21]. Zafra et al. [Citation19] studied the electrosorption of anions on highly porous carbon aerogels. They took chloride, nitrate and phosphate as adsorption targets and clearly evidenced that the carbon aerogel predominantly favored the electrosorption of these three kinds of anionic contaminants.
3.3 Removal of other inorganic contaminants
In addition, CDI technology was also applied to remove of K+ [Citation22], Na+ [Citation23], Ca2+ [Citation24], Mg2+ [Citation25] and other inorganic contaminants. On the basis of these works, Mossad et al. [Citation26] used a portable prototype of CDI unit to remove salt from the brackish groundwater. In this study, the total water recovery rate was between 75% and 80%, which demonstrated that the CDI equipment had excellent potential on reducing salinity and hardness at the remote brackish water source. In addition, Yoon et al. [Citation27] found the CDI with the Ca-alginate coated-electrode showed superior ion removal performance for hardness species such as calcium ions than that of the conventional CDI.
At present, the CDI technology has been further applied to industrial wastewater treatment, including power plant wastewater, coking wastewater [Citation28,Citation29], etc. In addition, the CDI technology has been put into practical production applications. The CDI industrial wastewater reuse units were performed in Changzhou (China) by Este Purification Equipment Company, and the CDI technology was applied in cold rolling wastewater treatment by Baosteel Group Company [Citation30]. Although the CDI technology has been applied in industrial production, there are still some influence factors which need attention and improvement for the higher efficiency in the application processes.
4. Influence factors
4.1 Materials
CDI technology is supported by the electrochemical double electric layer between the electrode and water. Thus, the formation of the double electric layer is determined by the properties of electrode materials, and then it will affect the adsorption effectivity [Citation9]. Therefore, it is important to prepare the electrode materials with high specific surface area, high specific capacitance, low resistance, environmental stability and good hydrophilicity. Some validated materials, mainly including metal oxides, conductive polymers and carbon materials were used to prepare electrodes. The electrodes prepare with metal oxides or conductive polymers work based on the Faraday capacitance principle, so that they will be consumed by electrochemical reactions. However, carbon material electrodes work on the principle of double electric layer theory with no consumption of raw materials and more environmental friendliness. In this paper, the influence of various properties of carbon electrode on CDI is briefly described (), and the corresponding methods to improve the deionization performance are introduced herein.
4.1.1 Pore size and specific surface area
The specific surface area (SSA) of material determines the number of adsorption sites to a certain extent, and at the same time, the pore size might affect the adsorption rate. In addition, the certain mutual influence between these two factors indicates that the impact on the deionization performance of the material needs to be comprehensively assessed (). Large SSA of the materials provides large internal resistance with more micropores (the width <2 nm), but the ion mobility will decrease. As well, the materials with large pores have smaller internal resistance and higher ion movement rate, while the SSA decreases accordingly [Citation12]. However, the negative correlation between SSA and pore size is not completely linear.
Table 1. Adsorption comparison of materials with different specific surface areas, pore volumes and pore size
It is evident from the comparison in that some other factors contribute to the removal capacity besides high SSA and pore size. The total pore volume and the distribution of various pore sizes also have an important effect on adsorption. The small pore size has a negative effect on the electrosorption as the increase of the overlap effect of electric field, which indicating the micropores have little contribution to adsorption due to the serious overlap effect and large mass transfer resistance. Consequently, the structural properties of materials will determine the efficiency of electrode. Huang et al. [Citation39] treated activated carbon with nitric acid and found that the micropore volume of the modified activated carbon was 0.283 cm3 g−1, which was smaller than that of the unmodified one (0.359 cm3 g−1). But the electrode adsorption capacity was 15% higher than that of the unmodified activated carbon. Moreover, one study showed that the medium pore size of the material played a major role in the adsorption of carbon aerogels [Citation40], but not all the experiment obtained the same result. Han et al. [Citation41] studied the influence of pore size distribution on the performance of capacitive deionized carbon electrode and found that both mesoporous and microporous contributed to the CDI. The similar conclusions were obtained by Porada et al. [Citation42]. However, Li et al. [Citation43] investigated the desalination performance of carbon aerogels with different pore sizes as electrode adsorption materials, and the results showed that the pore size had little effect with high concentration, while the SSA had more positive influence. Generally, the property of electrode materials should be discussed with the combination of pore size and SSA.
4.1.2 Pore structure
The pore structure of the material seriously affects the adsorption effect, especially the adsorption of different types of ions has a great difference. Peng et al. [Citation44] reported the adsorption behavior of different types of ions on materials with different pore structures, and then established the relationship between the electrochemical capacitive ability and ion dimensions. The study showed that one-valent cations had better mobility on two-dimensional materials than three-dimensional materials, while three-dimensional materials had better adsorption effect on divalent and trivalent cations than two-dimensional materials.
Previous studies showed that the formation of pores was independently controlled in the preparation of electrode [Citation45]. The micropores in aerogels are affected by carbonization process, while mesopores and macropores are affected by the preparation conditions and drying methods [Citation46]. The structural differences of materials obtained by different drying methods are shown in . During the natural drying, severe capillary tension at the solid-liquid-gas interfaces occurred under normal temperature and pressure, which led to structural shrinkage of materials. When the freeze-drying process is implemented, freezing rate and precursor concentration are important factors affecting pore structure [Citation47]. In addition, rapidly frozen process can produce small ice crystals that generate more micropores and macropores. Therefore, Mi et al. [Citation48] adopted the unidirectional freeze-drying method to prepare the aerogel with an orderly tubular structure in the vertical direction (). The aerogel achieved adsorption equilibrium quickly and had a good adsorption effect on Cu2+ in solution, indicating that the orderly tubular structure was profitable for ions to reach the adsorption site.
Figure 3. Different drying methods in the preparation of RF-CAs [Citation91]
![Figure 3. Different drying methods in the preparation of RF-CAs [Citation91]](/cms/asset/3d31bcdc-b028-42c3-aae5-8caebac3fad2/tcsb_a_1990798_f0003_oc.jpg)
Figure 4. (a)1% GO (Graphene oxide) aqueous suspension, (b) optical image of the GO emulsion gel, (c) low magnitude SEM image of GO aerogel, (d) high magnitude SEM image of GO aerogel cross section and (e) lateral section [Citation48]
![Figure 4. (a)1% GO (Graphene oxide) aqueous suspension, (b) optical image of the GO emulsion gel, (c) low magnitude SEM image of GO aerogel, (d) high magnitude SEM image of GO aerogel cross section and (e) lateral section [Citation48]](/cms/asset/1832c003-c2f0-4dd5-9866-78135e88cef3/tcsb_a_1990798_f0004_oc.jpg)
4.1.3 Electrochemical performance
The electrochemical properties of the electrode mainly include charge efficiency, conductivity, specific capacitance, and resistance. Each of these properties significantly affects the electrosorption capacity and the electrosorption rate, emphasizing the importance of electrochemical performance.
Charge efficiency refers to the ratio of equilibrium adsorption capacity to total charge [Citation49], which is 1 in the ideal case. But due to the existence of homo-valent ion repulsion, charge efficiency is generally less than 1 [Citation8] and it ranges from 0.3 to 0.8 in practice [Citation9]. On the basis of this concept, Huang et al. [Citation50] designed test and proved that the electrosorption capacity of the activated carbon fiber would increase with the increase of charge efficiency.
Besides, the electrical conductivity, specific capacitance and resistance also have great impacts on the electrosorption capacity of materials. The high electrical could ensure that the voltage uniformly distribute throughout the electrode and the low resistance could consume low heating [Citation12]. For example, Shen et al. [Citation51] prepared hollow ZIFs-derived nanoporous carbons (HZCs) with better electrochemical performances (low resistance and high specific capacitance) than the solid ZIFs-derived nanoporous carbons (SZCs) electrodes. Based on the better performances, the HZCs had a high electrosorption capacity of 15.31 mg g−1, while the electrosorption capacity of SZCs is less than 10 mg g−1. Generally, materials with high conductivity, large specific capacitance and low resistance have faster ion mobility, which contributes to better electrosorption performance.
4.1.4 Hydrophilicity of the electrode
The hydrophilicity of the electrode materials plays a vital role on the performance of adsorption, which is positive for polar pollutants removing in the aqueous medium. Thus, different hydrophilic materials should be selected according to the constituents of the target water to obtain higher benefits.
The hydrophobicity of materials is controlled by two factors, namely surface free energy (SFE) and roughness. Shaker et al. [Citation52] introduced normalized surface free energy (NSFE) as a combination of SFE and roughness to predict the contact angle of liquids on non-ideal low-energy surfaces. For a specific material, the surface energy is determined by the elements and constituents, while the surface roughness can be adjusted by introducing the heterogeneous atoms [Citation53]. Ye et al. [Citation54] prepared a low-density, mechanically compressible and water-self-recovering aerogel using PVA (polyvinyl alcohol) and GO, and modulated the amphiphilicity of the aerogel by adjusting the ratio of these two materials. In addition, previous study found that the addition of TiO2/SiO2 to the electrode improved the hydrophilicity and wettability of the electrode plate, and thus improved its adsorption efficiency [Citation55].
4.2 Device design
The design of CDI device also has a significant effect on the operation and the whole electrosorption process. The plate size, channel design and operation mode all affect the flow and contact area of the solution, while the plate spacing affects the size of the double layer.
4.2.1 Plate size
The difference of the sizes and specifications of the electrode plates with the same area would lead to the difference of the flow rate and process of the solution in the device, which eventually lead to the difference in the adsorption capacity. Generally, the long and narrow electrode has better adsorption effect under the same other test conditions. In addition, the sorption of ions is relatively high with the increase of electrode size and therefore the better capacity is to be obtained [Citation9,Citation56].
4.2.2 Plate spacing
The size of the double electric layer is affected by the distance between the adjacent plates, which has a certain effect on the adsorption. Smaller spacing provides larger thickness of the electric double layer and shorter distance of ions to the electric double layer, resulting in the faster adsorption rate. Moreover, the force of the electrode plate on ions decreases with the increase of the distance, and finally the adsorption amount decreases. In a previous study [Citation57], as the thickness of the feed channel increased from 3 mm to 8 mm, the salt adsorption capacity decreased from 17.7 mg g−1 to 16.3 mg g−1. In addition, if the plate spacing is too small to affect the water flow, it is easy to cause a short circuit. Hence, the electrode plates should be kept at an appropriate distance as far as possible to make the voltage distribution uniform.
4.2.3 Operation mode
The operation modes of CDI are generally divided into flow-by system and flow-through system. In the flow-by system, the solution flows between the two electrodes, while in the flow-through system, the solution flows through the electrodes (). The flow-through mode increases the contact area between the electrode and the solution, but the operation of this model requires self-supporting and easily permeable materials, which needs materials with higher requirements. Remillard Marielle et al. [Citation58] conducted a direct comparison of flow-through and flow-by operational mode using four types of electrode materials to better understand the influence of operational mode on CDI performance, and concluded that flow-through CDI had a higher average salt adsorption rate but flow-by mode tended to have higher salt adsorption capacity and better charge efficiency. Thus, under comprehensive consideration, the flow-by system is the main operation mode of CDI for simple design, low requirements for electrode materials and high removal efficiency.
Figure 5. CDI operation mode (a) Flow-by system and (b) flow-through system [Citation92]
![Figure 5. CDI operation mode (a) Flow-by system and (b) flow-through system [Citation92]](/cms/asset/958b934f-e782-4ae3-9c36-a821db2cd92b/tcsb_a_1990798_f0005_oc.jpg)
4.3 Environmental factors
Multiple studies show that the adsorption effect of CDI technology is also affected by environmental factors (), such as voltage, solution concentration and pH value [Citation59–60]. In addition, some researchers also explored the influence of various environmental factors and obtained the optimal operating parameters through response surface method or orthogonal test method, so that the electrode material could play a better adsorption performance [Citation61–63].
4.3.1 Applied voltage
Voltage is one of the important factors affecting capacitive adsorption. Hou et al. [Citation64] found that with the increase of voltage, the potential difference between the electrodes would be strengthened, and the double electric layer would become thicker and the charge density formed by free electrons on the electrode surface would increase accordingly, which led to requiring more ions to be adsorbed for neutralization. In addition, the increase of voltage would increase the driving force on ions, thus more micropores could be used to improve the adsorption capacity of the electrode [Citation65]. However, the voltage should not be too high because of the hydrolysis voltage in the solution. Under the ideal conditions, the standard potential of water is 1.23 V [Citation66]. When the design voltage is over the hydrolysis voltage, water molecules will undergo hydrolysis reaction, which changes the pH value of the solution, and then generates gas or precipitation. For example, when the voltage was higher than 0.6 V (the reduction potential of Cu2+), Cu2+ was reduced to Cu or Cu2O as presented in EquationEqs. (1)(1)
(1) -(Equation3
(3)
(3) ), and the products would decrease the effective surface area and cause serious degradation of electrode properties [Citation67].
Moreover, in view of economy, increasing the voltage means more energy consumption and higher costs, which did not meet the requirements of industrial and commercial development.
4.3.2 Flow rate
The flow rate is related to the residence time of the solution in the electrosorption device [Citation68]. The higher flow rate provides shorter residence time of the solution in the device and this further leads to little adsorption of ions, so that the quality of the treated water is not up to the standard. Nevertheless, when the flow rate was below a certain range, the adsorption effect was not good and the energy consumption of the device operation would increase if the water flow was too low [Citation26]. Agartan et al. [Citation69] have same result and speculated that within a limited range of flow rates, ions delivered effectively to the less accessible regions of the electrodes with increasing flow rate, which enhanced the mass transportation of the ions and the better utilization of the electrodes.
4.3.3 Ion concentration of the solution
Ion concentration is another vital factor affecting capacitance adsorption. Some studies [Citation70,Citation71] showed that the electrode obtained better electrosorption capacity in high solution concentration. Oren [Citation72] thought that it depended on the increase of the diffuse double-layer capacity and Hawks et al. [Citation73] attributed this phenomenon to the decrease of resistance. However, Sun et al. [Citation74] revealed that once the concentration was too high, the adsorption capacity of the electrode would decrease, and some studies had the same results [Citation75,Citation76]. This indicated that the advantage of electrosorption could not be manifested in higher original concentration of pollutants. Moreover, when the concentration of ions in the solution is too high, the electrosorption device will not meet the minimum removal requirements of wastewater, so that the final quality of water does not meet the corresponding standard requirements.
Five concentrations of target water were set up for the electrosorption study of ordered mesoporous carbon electrodes, and the results showed that the adsorption effect was poor, when the concentration was low [Citation77]. However, the adsorption saturation state may be reached in a short time when the concentration is high, which affects the desorption effect eventually. The reason for this phenomenon is that in the discharge stage, the cation and anion concentration will increase due to the release of ions. The electromigration of ions will disappear accordingly, and then ions move through free diffusion. However, as the existence of negative difference in the activity coefficient, the free diffusion slows down. So that it is difficult for ions to return from the pore to the solution and resulting in accumulation of ions. And then it finally affects the regeneration process of the electrode.
4.3.4 pH value of the solution
The pH of the solution has a great influence in the adsorption of ionic contaminants onto various adsorbents. Mi et al. [Citation48] used GO aerogel to adsorb Cu2+ in aqueous solution and found that the adsorption capacity of aerogel increased with the increase of the initial pH value. Generally, there were two reasons for this result. One was that the competitiveness decreased between Cu2+ and H3O+ for GO aerogel adsorption sites with the increase of solution pH, and ultimately increased the adsorption capacity. The another one was that, with the increase of solution pH, the oxygen-containing functional groups on the surface of aerogel were deprotonated, resulting in the electronegativity on the surface of aerogel and increasing the electrostatic attraction between aerogel and Cu2+. However, Porada et al. [Citation78] and He et al. [Citation79] found that the pH of solution had little or no influence on the electrosorption, which indirectly indicated that the electrode surface charge might have little influence on the electrosorption.
For the vast majority of ions, the pH of the solution affects the existing state. Zhou et al. [Citation80] found that the wastewater often contained Ca2+ and Mg2+. When the pH of the solution reached the precipitation condition, the precipitation formed on the electrode surface and consequently blocked the microporous structure of the electrode. This further affected the formation of the electrode double electric layer and reduced the ion removal efficiency. In addition, some other studies showed that the pH value of the solution would increase [Citation81] or decrease [Citation82] in the process of electrosorption, and the precipitation of heavy metal ions in the solution performed under a pH range. From an economic point of view, the formation of insoluble hydroxide precipitation damages the device and increase the cost.
4.3.5 Temperature of solution
The influence of temperature on the stability of the electric double layer is mainly manifested in two aspects [Citation83]. Firstly, the increase of temperature will decrease the electrode potential, weaken the electric field force and reduce the adsorption of ions. Secondly, the increase of temperature will reduce the viscosity of the solution, which leads to the acceleration of the ion movement rate in the solution, the decrease of the adhesion force of the electrode surface to ions, and the tendency of ions moving from the electrode surface to the solution. Many researchers believed that the structure of interfacial water alerted from hydrophobic to hydrophilic with the solution temperature decreasing, and a strong affinity was formed between materials and interface hydrated ions, which made it perform a high removal efficiency by an electrosorption at low temperature [Citation84,Citation85]. Later, a study by Předota et al. [Citation86] showed that the number of saturated charges in the double electric layer was also related to the electrolyte temperature to some extent.
4.3.6 Target ion properties
Generally, capacitive electrodes have different electrosorption performance for different ions, which depends on the atomic mass, charge and hydrated ionic radius [Citation87,Citation88]. Li et al. [Citation89] used graphene nano-flakes (GNFs) as electrodes to conduct the batch electrosorption experiments at the working conditions in NaCl, CaCl2, MgCl2 and FeCl3 solution, respectively, when the voltage and flow rate were constant. And they found that the electrosorption capacities of cations on the GNFs followed the order of Fe3+>Ca2+> Mg2+ > Na+. Huang et al. [Citation90] had the same results and obtained the conclusion that ions with high valence and ions with the same valence and small hydration radius had stronger electrostatic force and effective removal rate.
5. Summary and outlook
As a new water treatment technology with the advantages of low cost, high efficiency, safety and stability, green and environmental protection, CDI technology is in line with the strategic requirements of contemporary sustainable development. The review of the up-to-date progress on development can be concluded that CDI has been widely concerned and possesses broad development prospects. However, the researches on CDI are on-going and there are still some deficiencies in the theoretical models, material selection, architecture of the system, operational procedures and detailed systematic energy analysis of the technology where need be further developed.
Firstly, the theory of CDI is not perfect and mature, and the theoretical model of electric double layer needs to be improved and standardized. Secondly, there are current few moderate materials, so it is necessary to develop more electrode materials with better performance, better adsorption effect and lower cost. Thirdly, CDI technology is mostly suitable for the treatment of low concentration solution but not suitable for industrial application. Thus, it is necessary to break the environmental limitation and to ameliorate the practical application of CDI technology. The combination with other green energy technologies (wind energy, water energy, solar energy, etc.) will be developed for reducing cost and energy consumption. Therefore, the CDI would be comprehensively applied in various fields and then bring out the significant contribution in the friendly environment.
Acknowledgments
This work was supported by the National Natural Science Foundation of China under Grant [number 21377074].
Disclosure statement
No potential conflict of interest was reported by the authors.
Additional information
Funding
References
- Shiklomanov IA. Appraisal and assessment of world water resources. Water Int. 2000;25(1):11–32.
- He ML, Wang L, Lv YT, et al. Novel polydopamine/metal organic framework thin film nanocomposite forward osmosis membrane for salt rejection and heavy metal removal. Chem Eng J. 2020;389:124452.
- Santos CSLD, Reis MHM, Cardoso VL, et al. Electrodialysis for removal of chromium (VI) from effluent: analysis of concentrated solution saturation. J Environ Chem Eng. 2019;7(5):103380.
- Chitpong N, Husson SM. High-capacity, nanofiber-based ion-exchange membranes for the selective recovery of heavy metals from impaired waters. Sep Purif Technol. 2017;179:94–103.
- Xu ZT, Gu SW, Rana D, et al. Chemical precipitation enabled UF and MF filtration for lead removal. Journal of Water Process Engineering. 2021;41:101987.
- Wu SH, Shen LY, Lin Y, et al. Sulfite-based advanced oxidation and reduction processes for water treatment. Chem Eng J. 2021;414:128872.
- Luptakova A, Ubaldini S, Macingova E, et al. Application of physical–chemical and biological–chemical methods for heavy metals removal from acid mine drainage. Process Biochem. 2012;47(11):1633–1639.
- Ahmed MA, Tewari S. Capacitive deionization: processes, materials and state of the technology. J Electroanal Chem. 2018;813:178–192.
- Maheshwari K, Agarwal M, Solanki YS. Electrode material effect on electrochemical characterization, properties and operational parameters in capacitive deionization. Mater Today Proc. 2021;43:1204–1209.
- Chen FM. A new method for removing ions from water-the preliminary study of charge concentration. J Chem Indus Eng. 1999;50(1):114–117.
- Zou LD, Li LX, Song HH, et al. Using mesoporous carbon electrodes for brackish water desalination. Water Res. 2008;42(8–9):2340–2348.
- Oladunni J, Zain JH, Hai A, et al. A comprehensive review on recently developed carbon based nanocomposites for capacitive deionization: from theory to practice. Sep Purif Technol. 2018;207:291–320.
- Farmer JC, Bahowick SM, Harrar JE, et al. Electrosorption of chromium ions on carbon aerogel electrodes as a means of remediating ground water. Energy Fuels. 1997;11(2):337–347.
- Gaikwad MS, Balomajumder C. Simultaneous electrosorptive removal of chromium(VI) and fluoride ions by capacitive deionization (CDI): multicomponent isotherm modeling and kinetic study. Sep Purif Technol. 2017;186:272–281.
- Dai M, Xia L, Song SX, et al. Electrosorption of As(III) in aqueous solutions with activated carbon as the electrode. Appl Surf Sci. 2018;434:816–821.
- Shi Z, Wang XX, Bu J, et al. Study on electrosorption of Cd2+ in aqueous solution with CNT /MnO2 composite electrodes. J Safety Environ. 2018;18(3):1089–1094.
- Liu Y, Xia JJ, Lin JY, et al. Preparation of biomass-based carbon membrane and its adsorptive performance for Pb (II). Chin J Environ Eng. 2016;10(11):6171–6178.
- Shi Z, Jin ZQ, Deng L, et al. Electrosorption of Cu2+ in aqueous solution using CNT/PANI electrodes. China Environ Sci. 2016;36(12):3650–3656.
- Zafra MC, Lavela P, Macías C, et al. Electrosorption of environmental concerning anions on a highly porous carbon aerogel. J Electroanal Chem. 2013;708:80–86.
- Xing WL, Liang J, Tang WW, et al. Perchlorate removal from brackish water by capacitive deionization: experimental and theoretical investigations. Chem Eng J. 2019;361:209–218.
- Cohen I, Shapira B, Avraham E, et al. Bromide ions specific removal and recovery by electrochemical desalination. Environ Sci Technol. 2018;52(11):6275–6281.
- Hou CH, Huang CY. A comparative study of electrosorption selectivity of ions by activated carbon electrodes in capacitive deionization. Desalination. 2013;314:124–129.
- Bai Y, Huang ZH, Yu XL, et al. Graphene oxide-embedded porous carbon nanofiber webs by electrospinning for capacitive deionization. Colloids Surf A Physicochem Eng Asp. 2014;444(4):153–158.
- Lado JJ, Wouters JJ, Tejedor-Tejedor MI, et al. Asymmetric capacitive deionization utilizing low surface area carbon electrodes coated with nanoporous thin-films of Al2O3and SiO2. J Electrochem Soc. 2013;160(8):E71–E78.
- Linnartz CJ, Rommerskirchen A, Wessling M, et al. Flow-electrode capacitive deionization for double displacement reactions. ACS Sustain Chem Eng. 2017;5(5):3906–3912.
- Mossad M, Zhang W, Zou L. Using capacitive deionisation for inland brackish groundwater desalination in a remote location. Desalination. 2013;308:154–160.
- Yoon H, Lee J, Kim S-R, et al. Capacitive deionization with Ca-alginate coated-carbon electrode for hardness control. Desalination. 2016;392:46–53.
- Liu J, Xie FL, Zhang P. Experimental research on the application of electrosorption technique to wastewater treatment in power plants. Ind Water Treat. 2015;35(4):68–71.
- Xu YQ, Zhou BH, Zhang HT, et al. Pilot study on desalination of coke wastewater by electro-absorption technology. Res Environ Sci. 2014;27(6):663–669.
- Chen ZL, Song CY, Sun XW, et al. Progress in the research on the electrosorption desalination technology and its application. Ind Water Treat. 2011;31(4):11–14.
- Yang ZY, Jin LJ, Lu GQ, et al. Sponge-templated preparation of high surface area graphene with ultrahigh capacitive deionization performance. Adv Funct Mater. 2014;24(25):3917–3925.
- Elisadiki J, Jande YAC, Machunda RL, et al. Porous carbon derived from Artocarpus heterophyllus peels for capacitive deionization electrodes. Carbon. 2019;147:582–593.
- Liu Y, Chen TQ, Lu T, et al. Nitrogen-doped porous carbon spheres for highly efficient capacitive deionization. Electrochim Acta. 2015;158:403–409.
- Zhang Y, Chen L, Mao SD, et al. Fabrication of porous graphene electrodes via CO2 activation for the enhancement of capacitive deionization. J Colloid Interface Sci. 2019;536:252–260.
- Chen YZ, Yue MB, Huang ZH, et al. Electrospun carbon nanofiber networks from phenolic resin for capacitive deionization. Chem Eng J. 2014;252:30–37.
- Xu D, Tong Y, Yan TT, et al. N,P-codoped Meso-/microporous carbon derived from biomass materials via a dual-activation strategy as high-performance electrodes for deionization capacitors. ACS Sustain Chem Eng. 2017;5(7):5810–5819.
- Luo GM, Gao LX, Zhang DQ, et al. Highly efficient capacitive deionization electrodes from electrospun carbon nanofiber membrane containing reduced graphene oxide and carbon nanotubes. Desalin Water Treat. 2018;135:157–166.
- Luo GM, Wang YZ, Gao LX, et al. Graphene bonded carbon nanofiber aerogels with high capacitive deionization capability. Electrochim Acta. 2018;260:656–663.
- Huang W, Zhang YM, Bao SX, et al. Desalination by capacitive deionization process using nitric acid-modified activated carbon as the electrodes. Desalination. 2014;340:67–72.
- Wang WB, Zhang R, Liu XM, et al. Electrosorption of NaCl solution by phenolicfurfural based carbon aerogels. New Carbon Mater. 2007;22(1):65–69.
- Han LC, Karthikeyan KG, Anderson MA, et al. Exploring the impact of pore size distribution on the performance of carbon electrodes for capacitive deionization. J Colloid Interface Sci. 2014;430:93–99.
- Porada S, Zhao R, Van Der Wal A, et al. Review on the science and technology of water desalination by capacitive deionization. Pro Mater Sci. 2013;58(8):1388–1442.
- Li Z, Zhang YX. Study on electroadsorption desalt technology of carbon aerogel electrode water & wastewater engineering. 2008;34(5):177–180.
- Peng Z, Zhang DS, Shi LY, et al. Comparative electroadsorption study of mesoporous carbon electrodes with various pore structures. J Phys Chem C. 2011;115(34):17068–17076.
- Canal-Rodríguez M, Menéndez JA, Arenillas A. Performance of carbon xerogel-graphene hybrids as electrodes in aqueous supercapacitors. Electrochim Acta. 2018;276:28–36.
- Yamamoto T, Nishimura T, Suzuki T, et al. Control of mesoporosity of carbon gels prepared by Sol–Gel polycondensation and freeze drying. J NonCryst Solids. 2001;288(1–3):46–55.
- Lee J-H, Park S-J. Recent advances in preparations and applications of carbon aerogels: a review. Carbon. 2020;163:1–18.
- Mi X, Huang GB, Xie WS, et al. Preparation of graphene oxide aerogel and its adsorption for Cu2+ ions. Carbon. 2012;50(13):4856–4864.
- Zhao R, Biesheuvel PM, Miedema H, et al. Charge efficiency: a functional tool to probe the double-layer structure inside of porous electrodes and application in the modeling of capacitive deionization. J Phys Chem Lett. 2009;1(1):205–210.
- Huang ZH, Wang M, Wang L, et al. Relation between the charge efficiency of activated carbon fiber and its desalination performance. Langmuir. 2012;28(11):5079–5084.
- Shen JM, Li Y, Wang CH, et al. Hollow ZIFs-derived nanoporous carbon for efficient capacitive deionization. Electrochim Acta. 2018;273:34–42.
- Shaker M, Salahinejad E. A combined criterion of surface free energy and roughness to predict the wettability of non-ideal low-energy surfaces. Prog Org Coat. 2018;119:123–126.
- Miwa M, Nakajima A, Fujishima A, et al. Effects of the surface roughness onsliding angles of water droplets on superhydrophobic surfaces. Langmuir. 2000;16(13):5754–5760.
- Ye SB, Liu Y, Feng JC. Low-density, mechanical compressible, water-induced self-recoverable graphene aerogels for water treatment. ACS Appl Mater Interfaces. 2017;9(27):22456–22464.
- Chang LM, Duan XY, Liu W. Preparation and electrosorption desalination performance of activated carbon electrode with titania. Desalination. 2011;270(1–3):285–290.
- Li HB, Liang S, Li J, et al. The capacitive deionization behaviour of a carbon nanotube and reduced graphene oxide composite. ?J Mater Chem A. 2013;1(21):6335–6341.
- Kim N, Lee J, Hong SP, et al. Performance analysis of the multi-channel membrane capacitive deionization with porous carbon electrode stacks. Desalination. 2020;479:114315.
- Remillard Marielle E, Shocron AN, Rahill J, et al. A direct comparison of flow-by and flow-through capacitive deionization. Desalination. 2018;444:169–177.
- Dahiya S, Mishra BK. Enhancing understandability and performance of flow electrode capacitive deionisation by optimizing configurational and operational parameters: a review on recent progress. Sep Purif Technol. 2020;240:116660.
- Tang KX, Yiacoumi S, Li YP, et al. Optimal conditions for efficient flow-electrode capacitive deionization. Sep Purif Technol. 2020;240:116626.
- Porada S, Weingarth D, Hamelers HVM, et al. Carbon flow electrodes for continuous operation of capacitive deionization and capacitive mixing energy generation. ?J Mater Chem A. 2014;2(24):9313.
- Zhao Y, Hu XM, Jiang BH, et al. Optimization of the operational parameters for desalination with response surface methodology during a capacitive deionization process. Desalination. 2014;336:64–71.
- Bao SX, Chen Q, Zhang YM, et al. Optimization of preparation conditions of composite electrodes for selective adsorption of vanadium in CDI by response surface methodology. Chem Eng Res Des. 2021;168:37–45.
- Hou CH, Huang CY, Hu CY. Application of capacitive deionization technology to the removal of sodium chloride from aqueous solutions. Int J Environ Sci Technol. 2013;10(4):753–760.
- Sun SQ, Zhang WF, Cheng J, et al. Studies of desalination techniques and properties with activated carbon electrodes by capacitive desalination technology. Technol Water Treat. 2008;34(3):31–34.
- AlMarzooqi FA, Al Ghaferi AA, Saadat I, et al. Application of capacitive deionisation in water desalination: a review. Desalination. 2014;342:3–15.
- Wang C, Chen L, Liu S. Activated carbon fiber for adsorption/electrodeposition of Cu (II) and the recovery of Cu (0) by controlling the applied voltage during membrane capacitive deionization. J Colloid Interface Sci. 2019;548:160–169.
- Kim N, E-a L, Su X, et al. Parametric investigation of the desalination performance in multichannel membrane capacitive deionization (MC-MCDI). Desalination. 2021;503:114950.
- Agartan L, Hayes-Oberst B, Byles BW, et al. Influence of operating conditions and cathode parameters on desalination performance of hybrid CDI systems. Desalination. 2019;452:1–8.
- Liu LJ, Guo XR, Tallon R, et al. Highly porous N-doped graphene nanosheets for rapid removal of heavy metals from water by capacitive deionization. Chem Comm. 2017;53(5):881–884.
- Wang Z, Dou BJ, Zheng L, et al. Effective desalination by capacitive deionization with functional graphene nanocomposite as novel electrode material. Desalination. 2012;299:96–102.
- Oren Y. Capacitive deionization (CDI) for desalination and water treatment — past, present and future (a review). Desalination. 2008;228:10–29.
- Hawks SA, Ramachandran A, Porada S, et al. Performance metrics for the objective assessment of capacitive deionization systems. Water Res. 2019;152:126–137.
- Sun XW, Zhu FG. Principle and makeup of electrosorb technology in water treatment. Indus Water Wastewater. 2002;33(4):18–20.
- Jiang SJ, Wang HW, Xiong GQ, et al. Removal of nitrate using activated carbon-based electrodes for capacitive deionization. Water Supply. 2018;18(6):2028–2034.
- Liu XJ, Wang JL. Electro-adsorption characteristics and mechanism of Sr2+ ions by capacitive deionization and CFD analysis study. Prog Nucl Energy. 2021;133:103628.
- Huang -C-C, He J-C. Electrosorptive removal of copper ions from wastewater by using ordered mesoporous carbon electrodes. Chem Eng J. 2013;221:469–475.
- Porada S, Bryjak M, Van Der Wal A, et al. Effect of electrode thickness variation on operation of capacitive deionization. Electrochim Acta. 2012;75:148–156.
- He C, Ma JX, Zhang CY, et al. Short-circuited closed-cycle operation of flow-electrode CDI for brackish water softening. Environ Sci Technol. 2018;52(16):9350–9360.
- Zhou GZ, Wang ZF, Wang X, et al. Research on treatment of high salt wastewater by the graphite and activated carbon fiber composite electrodes. Environ Sci. 2014;35(5):1832–1837.
- Lee J-H, Bae W-S, Choi J-H. Electrode reactions and adsorption/desorption performance related to the applied potential in a capacitive deionization process. Desalination. 2010;258(1–3):159–163.
- Job N, Théry A, Pirard R, et al. Carbon aerogels, cryogels and xerogels: influence of the drying method on the textural properties of porous carbon materials. Carbon. 2005;43(12):2481–2494.
- Rao W, Li DG. Dynamics research on ion adsorption of electric double layer. J Naval Univ Eng. 2009;21(4):108–112.
- Mossad M, Zou L. A study of the capacitive deionization performance under various operational conditions. J Hazard Mater. 2012;213-214:491–497.
- Wang HJ, Xi XK, Kleinhammes A, et al. Temperature-induced hydrophobic-hydrophilic transition observed by water adsorption. Science. 2008;322(5898):80–83.
- Předota M, Machesky ML, Wesolowski DJ, et al. Electric double layer at the rutile (110) surface. 4. Effect of temperature and pH on the adsorption and dynamics of ions. J Phys Chem C. 2013;117(44):22852–22866.
- Christopher JG, DT T, Mel S. Electrosorption of inorganic salts from aqueous solution using carbon aerogels. Environ Sci Technol. 2002;36(13):3013–3019.
- Gao Y, Pan LK, Li HB, et al. Electrosorption behavior of cations with carbon nanotubes and carbon nanofibres composite film electrodes. Thin Solid Films. 2009;517(5):1616–1619.
- Li H, Zou L, Pan L, et al. Using graphene nano-flakes as electrodes to remove ferric ions by capacitive deionization. Sep Purif Technol. 2010;75(1):8–14.
- Huang Z, Lu L, Cai ZX, et al. Individual and competitive removal of heavy metals using capacitive deionization. J Hazard Mater. 2016;302:323–331.
- Ziegler C, Wolf A, Liu W, et al. Modern inorganic aerogels. Angew Chem. 2017;56(43):13200–13221.
- Luciano MA, Ribeiro H, Bruch GE, et al. Efficiency of capacitive deionization using carbon materials based electrodes for water desalination. J Electroanal Chem. 2020;859:113840.