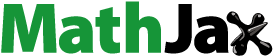
ABSTRACT
Supergene geochemical processes (SGPs) occur ubiquitously in the Earth´s surface, but their effects on the fate and bioavailability of polycyclic aromatic hydrocarbons (PAHs) in soil remain unclear. Study selected phenanthrene and pyrene as representative PAHs and yellow soil (YS) and limestone soil (LS) of karst area in southwest China, to investigate the effects of SGPs on the desorption and bioavailability of PAHs. Two soils were subjected to repeated freeze-thawing and long-term storage for SGPs. The hysteresis index values of pollutants increased with increase cycles of freeze-thawing and storage time. Under the SGPs, the phenanthrene fast desorption fraction of YS and LS decreased by 22.01% and 24.79%, respectively, and that of pyrene were by 23.81% and 28.12%, respectively. The desorption fraction of 24 hours was a better proxy of the fraction of bioavailability than that of 6 hours. The desorption rate of PAHs was negatively correlated with the hydrophobicity of PAHs.
Headings
SGPs significantly affected the desorption and bioavailability of PAHs in soil of karst area
F24h is a better proxy of Fbio than the F6h
A mechanism model was proposed to account for this effect under SGPs
Introduction
Pollution with organic substances is unquestioningly an important issue on a global level. In China, approximately 19.4% of the farmland area (4.66 million hectares) are polluted [Citation1]. According to the 1990 report of the United Nations Environment Program (UNEP), about 30–40 million tons of organic pollutants are released into the environment each year, and most of them entered the soil/sediment ecosystem [Citation2]. PAHs are organic pollutants with strong hydrophobicity. They are mainly derived from chemical production, pesticide emissions, waste incineration, and vehicle exhaust. In general, PAHs had low water solubility and high lipophilic properties and tended to be accumulated in organisms along the food chain [Citation3–8], which resulted in considerable attention from the international community [Citation9–11]. Many organic pollutants, such as PAHs, polychlorinated biphenyls (PCBs), and organo-chlorinated pesticides (OCPs), had been listed in the priority list of toxic and hazardous pollutants by the United States Environmental Protection Agency (EPA) [Citation12]. In the past 100 to 150 years, the concentration of PAHs in soil increased rapidly, especially in urban areas with extensive human activity [Citation13]. Since the 1970s, the international community have been working to reduce anthropogenic PAHs emissions, for example, the United States and European Union began to limit the use of PAHs since the mid-1980s. However, these pollutants can still be detected in various environmental matrices. Based on a previous report, 60% of the total amount of PAHs in China was stored in soils/sediments [Citation14], posing considerable health and ecological risks [Citation15–17].
Hydrophobic organic contaminants may enter the soil/sediment system directly or indirectly through dry and wet depositions, wastewater discharges, or chemical spills. Soil is the primary pooled reservoir of PAH, and as soil is a complex organic polymer, the environmental migration behavior of PAHs is mainly affected by adsorption [Citation18]. Under the influence of several factors, PAHs desorption and release processes in soil/sediment are more complex. In particular, partially ‘aging’ PAHs was difficult to naturally desorb, and thus has some impacts on assessing PAHs migration, transformation and bioavailability [Citation19].
Supergene geochemical processes (SGPs) affected the sorption and desorption behavior and the bioavailability of soil pollutants through change the type and intensity of interactions between soil and pollutants molecules [Citation6,Citation20]. For example, SGPs changes the soil aggregate structure and thus affects the migration and bioavailability of contaminant molecules. . The influence of SGPs on the environmental behavior of PAHs is also called ‘aging’. Long-term interaction between PAHs and soil is the most common aging phenomenon. Citation21,found that the aging of phenanthrene and pyrene in six soil types was mainly related to the content of total organic carbon [TOC) in soil. Citation22, studying the aging of two PAHs in 16 soil types, found that it was associated with TOC, micropores, and cation exchange capacity [CEC]. Natural soil is a dynamic system, and the variation in soil component structure represents a SGP. Citation23,showed that desorption of PAHs on biomass carbon decreased significantly after Humin acid (HA] was encapsulated with biomass charcoal. According to Zhao et al. [Citation20,Citation24,Citation25], the extraction efficiency of PAHs in soil changes after the freeze-thawing cycle. Olu-Owolabi et al (2014, 2015) investigated the effects of five tropical soils with different climatic conditions on adsorption and desorption of fluorene and pyrene, their study shown that pyrene was likely to be more available to biota and reached the aquifer faster in low organic matter soils than those with relatively higher organic matter and more so in warmer climes. Diagboya et al (2021) investigated the effects organic matter and iron oxides in three tropical soil species on PAH adsorption, this result indicated that depletion of soil organic matter (SOM) and soil iron oxides may lead to increased risks of PAHs in the environment, and meanwhile PAHs migrated faster in warm and low OM soil than cold and high OM soil. Although there are several reports about the impacts of SGPs on the interaction between soil matrices and PAHs, quantitative experiments on how SGPs influence PAHs desorption and bioavailability in different soils especially ecology fragile area are scarce.
SGPs mainly influence the sorption and desorption behavior of PAHs through two ways: 1) changes in soil composition and pore structure; 2) changes in the relative position of PAHs molecules in soil and the distribution among different components in soil [Citation20]. Since the responses of different soil types to different SGPs are different, the effects of SGPs on PAHs release and bioavailability in different soils may not be consistent. In the present study, two soil types (yellow soil (YS) and limestone soil (LS)) were used as representatives of different types of soil in the karst region of southwest China. Two common PAHs, phenanthrene and pyrene, were the target contaminants in the present study. In the laboratory, the two kinds soil samples containing the target compounds were treated with two natural SGPs (freeze-thawing cycles and long-term storage in the dark (aging)) were simulated. Equilibrium desorption and desorption kinetics of PAHs in simulated SGPs were studied and compared with those of treated and untreated soil samples. Hence, the objective of this study was to discuss the effects of SGPs on the transport, transformation, desorption, and release of PAHs in two type of soil in karst ecology fragile area and evaluate the bioavailability of PAHs in soil and the environmental health risk, and provide a scientific basis for the establishment of economically and environmentally viable management programs.
1 Material and Methods
1.1 Soils samples
Two kinds of soil, the yellow soil (YS) (26.673 N, 106.684E) and the limestone soil (LS) (25.307 N, 107.936E), were sampled from karst area of Guizhou Province in southwest China. The YS is rich in iron and characterized by moderate leaching potential, high acidity, heavy texture, and low content of organic matter, while the LS is an inception soil with a low degree of decalcification, slightly alkaline, and relatively high content of organic matter. Both soil samples were collected from the surface layer (0–12 cm) after the removal of the litter layer, and visible plant residues and rocks were manually removed from the samples. The soil was air-dried and slightly compacted to pass through a 2 mm sieve. The main physicochemical properties of the soils were shown in . The pH value was determined with a pH meter (Sartorius Professional Meter pp-50, Germany) after the dry soil was dispersed into deionized water with a solid-to-liquid ratio of 1:2.5 (v/v). The TOC was analyzed using an element analyzer (Vario Macro Cube, Elementary, Germany) as described elsewhere [Citation26]; specific surface area (SSA) and pore volume (PV) were measured by an automatic surface area and pore size analyzer [Autosorb-iQ, Conta Inc., USA) following the method described by Citation27.
Table 1. The pysico-chemical properties of the soil samples
1.2 Chemicals
Phenanthrene (Phen] and pyrene (Pyr) were selected as the target PAHs. They were highly common chemical industrial products and frequently found in natural environments. Both chemicals were purchased from the Sigma Chemical Reagent Company (HPLC degree with purity > 98%). Their main physico-chemical parameters were listed in .
Table 2. Basic physicochemical parameters of PAHs
2 Experimental method
2.1 Preparation of contaminated soil samples
To simulate the release of PAHs from soil samples, soils containing Phen or Pyr were prepared based on preliminary sorption experiments. Briefly, the soil sample (about 10 g) was placed in a Teflon reaction bottle (250 mL) and mixed with 200 mL background solution (0.005 mol/L CaCl2, 5 mg/L NaHCO3, and 100 mg/L NaN3 mixed solution). Subsequently a certain amount of Phen (about 2.0 mg) in methanol was added into the system using a micro-syringe, and the sealed sorption system was placed on a shaker (SY-550B, Taisite Instrument, China) at 125 rpm and 22 ± 1°C for 7 days. After this, another 0.5 mg Phen was added to ensure the equilibrium concentration of Phen (500 g/L), and the system was shaken for 14 days. Then, the reaction bottle was centrifuged and the supernatant was carefully removed. The residual soil slurry was freeze-dried, and the dried soil sample was crushed and mixed well to serve as the freshly contaminated soil sample for later desorption experiments. The soil samples contaminated with Pyr were prepared in the same way, with a total amount of 0.4 mg Pyr, allowing the equilibrium concentration of Pyr in the solution to be about 40 g/L. The co-solving effect was not considered in the present study, since the maximum amount of methanol added to the sorption system was less than 2% of the solution.
The actual contents of Phen and Pyr in the contaminated soil samples were determined by ultra-high-performance-liquid-chromatography (UHPLC, Agilent 1290) after ultrasonic extraction in acetone solution.
2.2 Simulation of SGPs
Two most common SGPs (freeze-thawing and long-term storage), were simulated in the laboratory to investigate their influences on the desorption of PAHs from soil. These two processes were imposed on the contaminated soils obtained above by following additional steps. After 21 days of shaking, instead of being centrifuged and freeze-dried immediately, two groups of the sorption system remained on the shaker, one for another 30 days (1 month) and another one for another 90 days (3 months), under the same conditions, to simulate long-term interaction. Three groups of the sorption system were stored at −8°C for 48 hours to thoroughly freeze, and then to thaw completely in a water bath (22°C) with gentle shaking for 24 hours to simulate freeze-thawing cycles. One group was treated for 3 times (FT3), one group for 15 times (FT15), and one group for 35 times (FT35). After treatment, the sorption systems were centrifuged, freeze-dried, crushed, and mixed to obtain aged contaminated soil samples for later use.
2.3 Experiments for desorption equilibrium and desorption rate
The desorption equilibrium and the desorption rate experiments of Phen and Pyr in the prepared fresh and aged contaminated soils were systematically studied and compared. Details of the desorption equilibrium experiments had been described elsewhere [Citation28]. In short, 1 g of the contaminated soil sample was introduced into a 15 mL glass centrifuge tube, and the tube was filled with as much background solution as possible to minimize the headspace above the liquid level. Subsequently, the glass centrifuge tube was capped tightly with a Teflon septum lined lid and shaken at 125 rpm and 22 ± 1°C for 28 days. After this, the tube was centrifuged (4000 rpm), and a supernatant aliquot (1.5 mL) was transferred into a small glass vial (5 mL) to mix with a known amount of methanol to stabilize the target chemical in the supernatant for later measurements; seven batches of centrifuge tubes containing different equilibrium concentrations of target chemicals in solution phase were prepared by applying seven different amounts of contaminated soils.
The sacrificial method was applied in the desorption rate experiment. Briefly, procedures similar to those in the equilibrium desorption experiment were conducted. Nine batches of glass centrifuge tubes containing pre-estimated amounts of contaminated soils and background solutions were prepared identically, sealed, and shaken immediately in the dark at room temperature (22 ± 1°C). Subsequently, at nine different time nodes (10 min, 20 min, 30 min, 1 h, 4 h, 1 d, 3 d, 1 wk, and 3 wk), one batch of tubes were randomly selected for centrifugation, and the supernatants were obtained and stabilized for measurements. After this, the concentration of compounds in the supernatant was determined. For all above experiments, triplicates were employed in each batch, and original soils without sorption treatments were involved as comparisons, and centrifuge tubes with solution but no soil samples were used as blanks.
2.4 Chemical analysis
The concentrations of Phen and Pyr in supernatants were determined by UHPLC (1290 Infinity, Agilent), following the method described elsewhere [Citation28]. Briefly, for the separation of Phen and Pyr, we used an ODS–C18, 5 μm, 2.1 × 250 mm back chromatographic column, and the mobile phase was deionized water and acetonitrile, and the column temperature was set to 35°C, and the ratio of the mobile phase was 22:78 (v/v), and the flow rate was 0.4 mL min−1, and the DAD detection wavelength was 254 nm, while the peak time was 2.5 minutes. The parameters for Pyr were deionized water and acetonitrile at a ratio of 32:68 (v/v), the flow rate was 0.6 mL min−1, and the FLD excitation wavelength of the detector was 270 nm, and the emission wavelength was 392 nm, and the peak time was 2.6 minutes.
2.5 Data analysis
The Freundlich model (EquationEq. (1(1)
(1) )) was applied to fit the desorption isotherms of PAHs:
In which, n and Kf were fitting parameters, and Ce and qe were equilibrium concentrations of PAHs in solution and solid phases, respectively. The sorption-desorption hysteresis index (HI) (EquationEq. (2(2)
(2) )), suggested by Citation29, was adopted to evaluate the overall sorption irreversibility of PAHs for the studied soil samples:
Where ns and nd were fitted Freundlich model parameters of sorption and desorption isotherms of PAHs, respectively.
The first-order binomial rate constant model fitted the experimental data well, and this model had been widely used in previous studies [Citation30,Citation31]. The mathematical formula of the first-order binomial rate constant model was as follows (EquationEq. (3)(3)
(3) ):
Where Q0 and Qt were the concentration of PAHs in the solid phase at the beginning and at time t (mg kg−1), respectively; Frap and Fslow were the ratios of fast and slow desorption component s (%), respectively, and Frap + Fslow = 1; krap and kslow were fitted rate constants of fast desorption and slow desorption (h−1), respectively.
3 Results and discussion
3.1 Effects of SGPs on PAHs contents in soils
The effects of SGPs on sorption capacities of PAHs in soils were investigated. According to the experiment, the amounts of Phen or Pyr introduced into all corresponding reaction systems, including 21 days of sorption, long-term interaction, and freeze-thawing cycle experiments, were nearly the same, but the ultimate contents of Phen and Pyr in the two studied soils (YS and LS) varied (). Numerous previous studies had identified soil organic carbon as the main factor affecting soil adsorption of PAHs. It can be seen from the physical and chemical properties of the soil (), the differences of TOC between YS and LS are small, and thus YS samples with higher HMC content which high sorption capacity, SSA and porosity showed higher sorption capacities for both Phen and Pyr in all experiments than LS with lower HMC content, SSA and porosity. Meanwhile, from the mineral content analysis, the clay minerals of YS was 68.91%, while the LS was 22.82%, which was also the reason for yellow soil adsorption of more PAHs than LS. It had been shown that the soil mineral content has a significant influence on the adsorption of organic pollutants [Citation32].
Figure 1. Contents of PAHs in soils subjected to different treatments
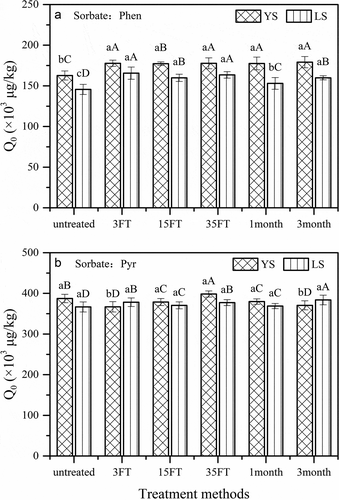
The contents of Phen and Pyr in YS samples after the experiments varied from 162.71 to 179.11 mg/kg (average 166.63 mg/kg) and from 17.39 to 20.59 mg/kg (average 18.60 mg/kg), respectively, and the contents of Phen and Pyr in LS samples after the experiments varied from 145.58 to 165.58 mg/kg and from 16.70 to 20.76 mg/kg, respectively, which showed that the type of soil influenced the sorption capacity for PAHs. The final contents of Phen and Pyr in soils increased consistently with the interaction time increase and freeze-thawing cycles increase, indicating that the effects of different SGPs on the contents of PAHs in soils were different. The deviations of the final contents of PAHs in the two soils after different SGPs were relatively small.
3.2 Effects of SGPs on PAHs desorption behavior
3.2.1 Effects of SGPs on desorption equilibrium of PAHs in soil
The desorption equilibrium of Phen and Pyr for the contaminated soils were systematically studied and compared to investigate the effects of different simulated SGPs. . The ns values had been reported previously [Citation28]; ns of Phen in YS and LS were 0.81 and 0.68, respectively, and ns of Pyr in YS and LS were 0.67 and 0.61, respectively. The nd values (n) were shown in . The main results of the equilibrium desorption experiments were illustrated in ; fitting parameters of the Freundlich model and the calculated single-point partition coefficient (KOC) and HI values were shown in .
Table 3. Parameters of Phen and Pyr equilibrium desorption for soils subjected to different supergene geochemical processes
In general, the Kf and the Koc values of the desorption isotherm of Pyr were significantly higher than those for Phen. So we infered that the logKow value of Pyr was larger and hydrophobicity was stronger than that of Phen, so desorption of Pyr was more difficult. This result agreed with previous findings. The higher the hydrophobicity of the PAHs, the more likely it was that they combine with solid media such as soil [Citation33,Citation34]. The desorption Kf values of the Phen and Pyr elevated with increase contact time and freeze-thawing cycle times. In general, the Kf of long time contact in YS and LS was larger than that of the freeze-thaw cycle. This indicated that time was the main factor to affect PAH desorption. The Kf values of Phen in YS and LS varied from 3434.82 to 11,915.20 and from 2651.51 to 9145.34, respectively; similarly, Pyr varied from 7707.33 to 13,655.22 and from 9272.51 to 13,902.72, respectively.
Generally, the isotherm n value of the Freundlich model showed the sorption energy distribution of the sorption sites. The smaller the difference in sorption point energy and the more linear the sorption, the closer the n value was to 1 [Citation35,Citation36]. The treatment also significantly affected the desorption equilibrium parameter n values of Phen and Pyr in soil, and the n value gradually decreased with an increasing intensity of the SGPs. The n value of Phen in YS and LS decreased from 0.65 to 0.52 and from 0.67 to 0.52, respectively;The n value of Pyr in YS and LS decreased from 0.60 to 0.32 and from 0.54 to 0.35, respectively. The simulated SGPs also showed a consistent effect on HI of Phen and Pyr in soil. With increase interaction time and freeze-thawing cycle times, the HI value of the two types soil samples increased gradually, which showed that under the same desorption experimental conditions, the desorption capacity of Phen and Pyr in both soil types decreased with an increase in the intensity of SGPs. The HI values of Phen and Pyr in untreated YS samples were 1.26 and 1.13, respectively, increasing to 1.56 and 2.08 for 3 months of storage in the dark and to 1.49 and 1.78 for 35 freeze-thawing cycles. The HI values of Phen and Pyr in untreated LS samples were 1.02 and 1.13, respectively, and increased to 1.23 and 1.76 for 3 months of storage in the dark and to 1.32 and 1.41 for 35 times freeze-thawing cycles, respectively. These trends indicated that the desorption and release ability of PAHs in soil decreases with increasing transformation degree of SGPs. The researchers thought that this was related to the decrease of cationic concentration in solution. With the increase of freeze-thawing cycles, the Ca2+ concentration in solution will be crystallized and precipitated, and the ion concentration in solution decreased. However, previous studies had suggested that Ca2+ was acidic cations, which was helpful to the desorption of phenanthrene and fluorescence [Citation37]. Therefore, when freeze-thawing increases, Ca2+ concentration decreases, desorption intensity decreases and desorption hysteresis increases.
3.2.2 Effects of SGPs on PAHs desorption kinetics in soil
showed the results of the desorption experiment. The first-order binomial rate constant model fitted the experimental data well. The residual concentrations of Phen and Pyr in soil decreased with increasing desorption time. The desorption of Phen in all soils was close to equilibrium after about 1 day, while that of Pyr was close to equilibrium after 3 days. Hydrophobicity was an important factor to controll the desorption rates of Phen and Pyr, and the simulated SGPs had a significant effect on these rates. With increasing freeze-thawing cycle times and the dark storage time, the residual amounts of Phen and Pyr increased gradually when desorption reached an equilibrium. The data fitting results () showed that, in general, with the increase in freeze-thawing cycle times and interaction time, the rapidly desorbing fractions (Frap) decreased gradually, while the slowly desorption fractions [Fslow) increased correspondingly. The change of Frap was shown that the Phen and Pyr in YS after the SGPs were reduced by 22.01% and 24.79%, respectively; and that the Phen and Pyr in LS after the SGPs were reduced by 23.81% and 28.12%, respectively. Compared with freeze-thawing, Pyr desorption was more strongly influenced than Phen desorption after long-term storage in the dark, but the difference between the two types of PAHs was not significant, and there was no consistent tendency between the two soil types.
Table 4. Fit parameters for PAHs desorption from soils subjected to different supergene geochemical processes
Under the action of different SGPs, rapid and slow desorption rates of Phen and Pyr did not show the same tendency, irrespective of the soil type. With increasing storage time, the slow desorption rate decreased. The rapid desorption rate was 3 to 4 orders of magnitude higher than the slow desorption rate, which was agreement with previous findings. Citation31showed that the desorption rates values of krap were in the order of 10−1/h, and those of kslow were in the order of 10−4 /h; and Citation33,stated that the rapid desorption rate constant of soil sample was > 10−1 /h, and the slow desorption rate ranged from 10−3 to 10−4 /h. In our study, the desorption rate was related to the increase in PAHs with increasing degree of binding of the adsorbent. The PAHs would slowly diffuse into the regions and sites that could not be reached in the initial stage and were thus more difficult to desorb Citation38. In freeze-thawing experiments, the fast desorption components of Phen and Pyr gradually decreased over time, but the corresponding slow desorption components increased. This was not only related to the various minerals and the organic matter, but also the precipitation of inorganic ions in the soil [Citation39,Citation40]. During freeze-thawing, precipitation-solution-precipitation may take place repeatedly, and the spatial position of mineral precipitation was also changed, which may cover the surface of adsorbing PAHs, resulting in the amount of isolated pollutants increasing. In addition, the thermal expansion and contraction of pore water during freeze-thawing may result in increased pores and connected pores among, leading to the decrease in the fast desorption component [Citation41]. Previous studies had shown that the migration behavior of PAHs in soil was closely related to their hydrophobicity. The fast and slow desorption rates of PAHs decreased with increasing hydrophobicity of PAHs, as shown in , which was consistent with previous findings [Citation38].
In general, the desorption behavior of Pyr was changed more significantly after simulated SGPs compared with Phen, with a variation range between 3.08%and 24.79% in LS samples of Phen and between 10.72% and 28.12% in LS samples of Pyr. The rapid desorption fraction of LS was slightly smaller than that of YS, which was related to the physical and chemical properties of the two soils. The specific surface area and the micropore volume of YS was larger, but the organic carbon content was lower. The YS was a typical iron-rich soil, high in iron and aluminum oxides, facilitating the iron hydroxide precipitation. In contrast, LS had smaller specific surface area and micropore volume, a higher organic carbon content, and was more calcareous, facilitating the formation of calcium carbonate precipitation.
3.2.3 Effects of soil properties on PAHs desorption under SGPs
Previous studies showed that the content of soil organic matter was the main factor to determined soil sorption/desorption of PAHs [Citation9,Citation21,Citation42], along with the physical and chemical properties of soil organic matter [Citation42–45]. However, the response of sorption/desorption of PAHs to SGPs in different soils with varying properties had hardly been investigated [Citation22]. One exception was the study of Citation24, who investiated the effects of simulated freeze-thawing cycles on the extractability of Pyr in different soils, albeit without a consistent correlation. The authors assumed that the soil samples in their experiment had gone through several freeze-thawing cycles, resulting in contrary results. In addition, these authors also reported that the efficiency of using anionic surfactant (SDBS) to extract PAHs in soil did not follow a consistent pattern after long-term interaction between PAHs and different soils; and they attributed this result to the different contents and characteristics of organic matter in different soils [Citation20].
Our experiments showed that the sorption capacity for Phen and Pyr was higher in YS than that in LS, and the Kf and HI values of YS were higher than those of LS. This was consistent with the large specific surface area and the highly condensed matter content in YS. In another study, the sorption ability of condensed organic matter to PAHs in soil was significantly higher than that of indefinite morphology organic matter [Citation46,Citation47]. The ratio of humin carbon to total organic carbon in YS was 32.95, which was significantly higher than that in LS (26.21%); In YS, the ratio of HA/FA was higher than that in LS (). With the increase of simulated freeze-thawing cycle times and long-term storage in the dark, the values of the desorption isotherm parameters n, Kf, and HI changed in both soil types; the n values gradually decreased, and the Kf and HI values gradually increased. However, the effects of different simulated SGPs on the changes in equilibrium desorption state of the two soils were different. Taking single Point Organic carbon Correction Distribution coefficient (KOC) of Phen as example, the KOC value of YS increased by 131.08% and 85.92% after storage in the dark for 3 months and 35 times freeze-thawing compared with that of untreated YS. And the KOC value of LS increased by 56.38% and 115.28% after storage in the dark for 3 months and 35 times freeze-thawing compared with that of untreated YS. Freeze-drying had a more significant influence on the desorption equilibrium state of LS, leading us to infer that the influences of different SGPs on PAHs desorption behavior in soil was also closely related to the soil properties. This was verified by the PAHs desorption rate in soil; the fast and sow desorption rates of PAHs in LS decreased significantly after the simulated supergene geochemical process treatment. However, the fast and slow desorption rates of PAHs in YS were not significantly changed (). The LS contained more secondary carbonate minerals, mainly calcium carbonate [Citation48]. The soil minerals dissolved or precipitated with variation of water content in the soil, resulting in the exposure or coverage of PAHs sorption surface and in a temporary blockage of the contact between adsorbed PAHs and the water phase. In the desorption experiment, particularly under short contact times, the PAHs desorption rate was affected by the dissolution of carbonate in soil, which resulted in decreased PAHs desorption in LS (). The conceptual model built by Citation20, also believes that after long adsorption, freeze-thaw cycle will reduce the extraction efficiency of SDBS, the reason is the long adsorption of the pollutant molecules into the organic matter inner and deeper pores, after freeze-thaw soil glass domain expaned increases the high active adsorption site, which is consistent with the decline of the apparent geochemical process under the soil and soil system, soil organic matter component [glass and rubber state) changes. Previous studies had reported similar results. For example, Citation49, found that with the increase in Ca ion intensity, the sorption rate and sorption capacity of PAHs in soil both decreased. Citation37, found differences in the effects of different metal ion types on PAHs desorption in soil. Therefore, we thought that the variation in PAHs desorption behavior of iron- and aluminum-rich YS and calcium-rich LS during the supergene geochemical process was related to the main ion types and ionic intensity in the soil. The dynamic variation of soil minerals significantly affected the desorption and release of PAHs in soil. However, we only investigated two soil types, and further studies with more soil types were needed.
Figure 5. The mechanism of SGP Changing the behavior of Organic Pollutants in Soil
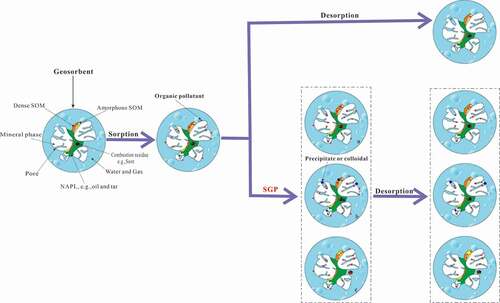
3.3 Effects of SGPs on PAHs bioavailability in soil
The distribution, migration, and fate of PAHs in the natural environment were the core problems in accurately evaluating the bioavailability of PAHs in the environment. The bioavailability directly affected human health and the natural environment. Accurate evaluation of the bioavailability of PAHs in soil could provide a scientific basis for the remediation and treatment of contaminated areas, enable the selection of specific microorganisms to degrade PAHs in soil, and facilitate the development of adequate policies. Therefore, the rapid evaluation of PAHs bioavailability in the natural environment was of paramount importance. In previous studies, the fast desorption component (Frap) corresponded to the bioavailability of PAHs [Citation33,Citation50]. Using Tenax and HPCD to study the bioavailability of PAHs molecules, it was considered that F6h had a good linear correlation with Frap [Citation51–53], while some scholars showed that F24h had a good linear relationship with Frap [Citation54]. These studies did, however, not consider the dynamic effects of SGPs, and the conclusions were subject of debate. Our study showed that under the condition of simulated SGPs, the correlation between the 24-hour fast desorption fraction ratio (F24h) and the substitute fraction of bioavailability (Fbio) was significantly better than that obtained by the 6-hour desorption experiment fitting the fast desorption fraction ratio (F6h) (). The bioavailability values of Phen and Pyr were Fbio = 0.899 × F24h and Fbio = 0.905 × F24h, respectively. Therefore, we suggest that under natural conditions, the fast desorption components of PAHs in natural environment can be rapidly determined by using the fast desorption fraction F24h, enabling an accurate evaluation of the bioavailability of PAHs. The two soil types with different properties showed significant differences in fast desorption components and corresponding bioavailability components after being subjected to SGPs. After three cycles of freeze- thawing, the bioavailability of Pyr in soil decreased by about 10%, while that of Phen decreased only by about 2%. When subjected to the same SGPs, the bioavailability of PAHs with higher ring number and stronger hydrophobicity rapidly decreased, with small environmental risks. The stronger the hydrophobicity of PAHs and the greater the intensity and more complex the interaction types of interaction with environmental media were, and the more difficult the PAHs to desorb were . However, under different SGPs, these corresponding bioavailability reduction components were likely to be re-exposed and released into the environment as a result of the variations in the sorption-desorption system. In this sense, further studies should be needed to focus on the PAHs isolated in environmental media.
4. Conclusion
The migration, transformation, and fate of PAHs were closely related to the physical and chemical properties of the soil-PAHs system, and the influence of SGPs on PAHs could not be ignored. The results were as follows: (i) the desorption behavior and bioavailability of different types of soils were different under different SGPs which was closely related to soil properties and external environmental factors. Under the SGPs, the Phen fast desorption fraction of YS and LS decreased by 22.01% and 24.79%, respectively, and that of Pyr were by 23.81% and 28.12%, respectively. (ii) The bioavailability of PAHs was linearly correlated with the desorption of F24h under SGPs. Hence, the desorption of F24h could be used as a indicators of bioavailability of PAHs in soil. (iii) Under the condition of SGPs, the desorption rate of PAHs in soil was negatively correlated with the hydrophobicity of PAHs.Our results provide a theoretical basis for the migration, transformation, and fate of PAHs in real environments. Especially in areas with complex SGPs, such as the karst region in southeast China, the effect of this action on the desorption behavior of PAHs should be considered.
Ethics approval and consent to participate
Not applicable.
Consent for publication
Not applicable.
Availability of data and materials
All data generated or analysed during this study are included in this published article [and its supplementary information files].
Authors’ contributions
All authors contributed to the study conception and design. Material preparation, data collection and analysis were performed by [An Xianjin, Li Wei, Di Xinyue], [An Xianjin, Li Wei] and [An Xianjin, Li Wei, Di Xinyue, Xiao Baohua]. The first draft of the manuscript was written by [An Xianjin and Li Wei], and all authors commented on previous versions of the manuscript. All authors read and approved the final manuscript.
Disclosure statement
The authors declare that they have no competing interests.
Correction Statement
This article has been republished with minor changes. These changes do not impact the academic content of the article.
Additional information
Funding
References
- Chen R, De Sherbinin A, Ye C, et al. China’s soil pollution: farms on the Frontline. Science. 2014;344(6185):691.
- Peng SW, Zhou QX. Research advances in phytoremediation and its mechanisms of POPs-contaminated soils Chinese J Ecol(in chinese).2008;27(3):469–475
- Diagboya PN, Olu-Owolabi BI, Dikio ED, et al. Concentration-dependent and simultaneous sorption and desorption of pyrene and fluorene on major soil minerals in sub-Saharan Africa. Appl Clay Sci. 2018;153:257–264.
- Diagboya PN, Mtunzi FM, Adebowale KO, et al. Assessment of the effects of soil organic matter and iron oxides on the individual sorption of two polycyclic aromatic hydrocarbons. Environ Earth Sci. 2021;80(6):227.
- Hofman J, Hovorkova I, Semple KT. The variability of standard artificial soils: behaviour, extractability and bioavailability of organic pollutants. J Hazard Mater. 2014;264:514–520.
- Olu-Owolabi BI, Diagboya PN, Adebowale KO. Sorption and desorption of fluorene on five tropical soils from different climes. Geoderma. 2015;239:179–185.
- Olu-Owolabi BI, Diagboya PN, Adebowale KO. Evaluation of pyrene sorption–desorption on tropical soils.J Environ Manage. 2014;137: 1–9
- Teixeira SCG, Ziolli RL, Da Costa Marques MR, et al. Study of pyrene adsorption on two brazilian soils. Water Air Soil Pollut. 2011;219:297–301.
- Chiou CT, Peters LJ, Freed VH. Physical concept of soil-water equilibria for non-ionic organic-compounds. Science. 1979;206(4420):831–832.
- Ren XY, Zeng GM, Tang L, et al. Sorption, transport and biodegradation–an insight into bioavailability of persistent organic pollutants in soil. Sci Total Environ. 2018;610:1154–1163.
- Zhu QH, Wu YC, Zeng J, et al. Influence of organic amendments used for benz[a]anthracene remediation in a farmland soil: pollutant distribution and bacterial changes. J Soil Sediment. 2020;20(1):32–41.
- Carmichael LM, Christman RF, Pfaender FK. Desorption and mineralization kinetics of phenanthrene and chrysene in contaminated soils. Environ Sci Technol. 1997;31(1):126–132.
- Krauss M, Wilcke W, Zech W. Availability of polycyclic aromatic hydrocarbons (PAHs) and polychlorinated biphenyls (PCBs) to earthworms in urban soils. Environ Sci Technol. 2000;34(20):4335–4340.
- Wang JZ, Zhu CZ, Chen TH. PAHs in the Chinese environment: levels, inventory mass, source and toxic potency assessment. Environ Sci-Proc Imp. 2013;15:1104–1112.
- Sun L, Zang S. Relationship between polycyclic aromatic hydrocarbons (PAHs) and particle size in dated core sediments in Lake Lianhuan, Northeast China. Sci Total Environ. 2013;461:180–187.
- Wei R, Ni J, Guo L, et al. The effect of aging time on the distribution of pyrene in soil particle-size fractions. Geoderma. 2014;232:19–23.
- Li Z. Health risk characterization of maximum legal exposures for persistent organic pollutant (POP) pesticides in residential soil: an analysis. J Environ Manage. 2018;205:163–173.
- Lamichhane S, Krishna KCB, Sarukkalige R. Polycyclic aromatic hydrocarbons (PAHs) removal by sorption: a review. Chemosphere. 2016;148:336–353.
- Tan WB, Liu NK, Dang QL, et al. Insights into the removal efficiencies of aged polycyclic aromatic hydrocarbons in humic acids of different soil aggregate fractions by various oxidants. Environ Pollut. 2020;264:114678.
- Zhao Q, Xing BS, Tai PD, et al. Effect of freeze-thawing cycles on soil aging behavior of individually spiked phenanthrene and pyrene at different concentrations. Sci Total Environ. 2013a;444:311–319.
- Bogan BW, Sullivan WR. Physicochemical soil parameters affecting sequestration and mycobacterial biodegradation of polycyclic aromatic hydrocarbons in soil. Chemosphere. 2003;52(10):1717–1726.
- Chung N, Alexander M. Effect of soil properties on bioavailability and extractability of phenanthrene and atrazine sequestered in soil. Chemosphere. 2002;48(1):109–115.
- Zhou ZL, Sun HW, Zhang W. Desorption of polycyclic aromatic hydrocarbons from aged and unaged charcoals with and without modification of humic acids. Environ Pollut. 2010;158(5):1916–1921.
- Zhao Q, Li PJ, Stagnitti F, et al. Effects of aging and freeze-thawing on extractability of pyrene in soil. Chemosphere. 2009;76(4):447–452.
- Zhao Q, Xing BS, Tai PD, et al. Effect of freeze-thawing cycles on aging behavior of phenanthrene, pyrene and their mixture in soil. Sci Total Environ. 2013b;452-453:246–252.
- Di XY, Xiao BH, Dong H, et al. Implication of different humic acid fractions in soils under karst rocky desertification. Catena. 2019;174:308–315.
- Aschermann G, Zietzschmann F, Jekel M. Influence of dissolved organic matter and activated carbon pore characteristics on organic micropollutant desorption. Water Res. 2018;133:123–131.
- An XJ, Xiao BH, Di XY, et al. Effect of inorganic ion precipitation on hydrophobic organic pollutant adsorption by non-extracted soil organic matters. Earth and Environ (in chinese). 2016;44:572–580.
- Sanchez-Camazano M, Sanchez-Martin MJ, Rodriguez-Cruz MS. Sodium dodecyl sulphate-enhanced desorption of atrazine: effect of surfactant concentration and of organic matter content of soils. Chemosphere. 2000;41(8):1301–1305.
- He Y, Liu ZZ, Zhang J, et al. Can assessing for potential contribution of soil organic and inorganic components for butachlor sorption be improved? J Environ Qual. 2011;40(6):1705–1713.
- Nichols EG, Gregory ST, Musella JS. The impact of vegetation on sedimentary organic matter composition and PAH desorption. Environ Pollut. 2008;156(3):928–935.
- Cheng J, Ye Q, Lu ZJ, et al. Quantification of the sorption of organic pollutants to minerals via an improved mathematical model accounting for associations between minerals and soil organic matter. Environ Pollut. 2021;280:116991.
- Cornelissen G, Pcm V-N, Govers HAJ. Mechanism of slow desorption of organic compounds from sediments: a study using model sorbents. Environ Sci Technol. 1998;32(20):3124–3131.
- Crampon M, Bureau F, Akpa-Vinceslas M, et al. Correlations between PAH bioavailability, degrading bacteria, and soil characteristics during PAH biodegradation in five diffusely contaminated dissimilar soils. Environ Sci Poll Res. 2014;21:8133–8145.
- Huang WL, Weber WJ. A distributed reactivity model for sorption by soils and sediments .10. Relationships between desorption, hysteresis, and the chemical characteristics of organic domains. Environ Sci Technol. 1997;31(9):2562–2569.
- Pignatello JJ. Soil organic matter as a nanoporous sorbent of organic pollutants. Adv Colloid Interface Sci. 1998;76:445–467.
- Chen G, Cheng L, Ding A, et al. Effects of metallic cations on desorption of phenanthrene and fluoranthene from long-term contaminated soil particles. Procedia Eng. 2011;18:411–416.
- Pcm V-N, Poot A, Koelmans AA. Analysis of organic contaminant desorption kinetic data for sediments and soils: implications for the Tenax extraction time for the determination of bioavailable concentrations. Sci Total Environ. 2014;490:235–238.
- Joseph SD, Arbestain MC, Lin Y, et al. An investigation into the reactions of biochar in soil. Aust. J Soil Res. 2010;48(7):501–515
- Wang C, Li FC, Shi HZ, et al. The significant role of inorganic matters in preservation and stability of soil organic carbon in the baoji and luochuan loess/paleosol profiles, central China. Catena. 2013;109:186–194.
- Ma RM, Yu J, Liu B, et al. Effects of pore structure characterized by synchrotron-based micro-computed tomography on aggregate stability of black soil under freeze-thaw cycles. Soil Till Res. 2020;207:104855.
- Luo L, Lin S, Huang H, et al. Relationships between aging of PAHs and soil properties. Environ Pollut. 2012;170:177–182.
- Adeyinka GC, Moodley B. Effect of aqueous concentration of humic acid on the sorption of polychlorinated biphenyls onto soil particle grain sizes. J Soil Sediment. 2018;19(3):1543–1553.
- Barriuso E, Benoit P, Dubus IG. Formation of pesticide nonextractable (bound) residues in soil: magnitude, controlling factors and reversibility. Environ Sci Technol. 2008;42(6):1845–1854.
- Zhang J, He M. Effect of structural variations on sorption and desorption of phenanthrene by sediment organic matter. J Hazard Mater. 2010;184(1–3):432–438.
- Xiao BH, Yu ZQ, Huang WL, et al. Black carbon and kerogen in soils and sediments. 2. Their roles in equilibrium sorption of less-polar organic pollutants. Environ Sci Technol. 2004;38(22):5842–5852.
- Xing BS. Sorption of naphthalene and phenanthrene by soil humic acids. Environ Pollut. 2001;111(2):303–309.
- Di XY, Dong H, An XJ, et al. The effects of soil sand contents on characteristics of humic acids along soil profiles. Acta Geochim. 2016;35(3):251–261
- Luo XM, Liu CM. Effects of Ca2+ ionic strength on sorption of polycyclic aromatic hydrocarbons (PAHs) on soils and sediments in Yellow River Delta. Ecol Environ(in chinese). 2006;15:983–987.
- Kraaij R, Seinen W, Tolls J. Direct evidence of sequestration in sediments affecting the bioavailability of hydrophobic organic chemicals to benthic deposit-feeders. Environ Sci Technol. 2002;36(16):3525–3529.
- Cornelissen G, Rigterink H, Ten- Hulscher DEM, et al. A simple Tenax (R) extraction method to determine the availability of sediment-sorbed organic compounds. Environ Toxicol Chem. 2001;20(4):706–711.
- Doick KJ, Clasper PJ, Urmann K, et al. Further validation of the HPCD-technique for the evaluation of PAH microbial availability in soil. Environ Pollut. 2006;144(1):345–354.
- Xu YP, Gan J, Wang Z, et al. Effect of aging on desorption kinetics of sediment-associated pyrethroids. Environ Toxicol Chem. 2008;27(6):1293–1301.
- Shor LM, Rockne KJ, Taghon GL, et al. Desorption kinetics for field-aged polycyclic aromatic hydrocarbons from sediments. Environ Sci Technol. 2003;37(8):1535–1544.
- Yang K, Zhu LZ, Xing BS. Adsorption of polycyclic aromatic hydrocarbons by carbon nanomaterials. Environ Sci Technol. 2006;40(6):1855–1861.
- Ling W, Zeng Y, Gao Y, et al. Availability of polycyclic aromatic hydrocarbons in aging soils. J Soil Sediment. 2010;10(5):799–807.
- Karickhoff SW, Brown DS, Scott TA. Sorption of hydrophobic pollutants on natural sediments. Water Res. 1979;13(3):241–248.