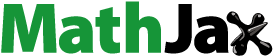
ABSTRACT
Seven potentially toxic elements (PTEs) including Cr, Ni, Cd, Zn, Cu, Pb and As of soil samples from Qinghai-Tibet Plateau (QTP) were measured to assess the degree of contaminations. To have a comprehensive understanding of PTEs’ risks in QTP, the single-step nitric acid extraction and diffusive gradients in thin-films (DGT) technique were employed in this study for the determination of PTEs’ total amount as well as bioavailable content, respectively. Haibei state of Qinghai province was the most contaminated sites by PTEs. Behaviors of PTEs should be highly concerned as they can produce continuous bioavailable effects to ecological systems. High DGT concentrations of Zn and Cr showed their remarkable available risks in soils. In the environment with insufficient organic matter and slight pH variations, phosphorus level was the main contributor to affect the mobility of labile PTEs, especially for Pb and Ni. Concentration ratio between DGT measurement and soil solution and correlation analysis confirmed the high bioavailability of Pb, Zn and Cr, leading to short-term mixture effects on ecological systems, while the long-term release dynamics were found for As and Cu. Results from road-side and river-side soils of QTP evidenced the short-term bioavailable risks were possibly attributed to intensive anthropogenic activities including traveling industries and vehicle driving, whereas long-term bioavailable risks was from the natural transportation and deposition.
1. Introduction
Soil contaminated by the potentially toxic elements (PTEs) has received great attention in recent decades. Their high persistence and low degradability cause the enrichment in terrestrial environments and thereby accumulating in organisms. Soils are major and important terrestrial sink for the storage of various PTE, playing vital roles in maintaining the stability and safety of global ecology [Citation1–3]. PTE at different concentration range in soil can cause either acute or chronic toxicity to ecological systems, and accumulate in food chains and webs to affect human health [Citation4,Citation5]. Chromium (Cr), nickel (Ni), cadmium (Cd), zinc (Zn), copper (Cu), lead (Pb) and arsenic (As) originated from natural and anthropogenic sources are main components of PTEs in soils, attracting considerable public concerns [Citation6,Citation7]. Despite the necessity of low-level PTE ions in physiological and metabolic processes for organisms, exposures to high-content PTE can produce severe damage on enzymes and DNA structure [Citation8]. Therefore, investigating contents and risks of heavy metals in natural environment is required to stabilize ecological systems in soils and protect human health.
Ecological risks of PTEs contamination including metals and metalloids in the soil environment of polar and plateau regions have attracted considerable attentions since these area are vulnerable to highly bioavailable pollutants. The soil environment in the Qinghai-Tibet Plateau (QTP) involves low-level PTEs, the average amounts of different heavy metals including Cr, Pb, As, Zn, Cu and Cd determined in previous reports are 83, 157, 13, 67, 31 and 0.07 mg/kg, but unexpected PTE contamination has been found in this area [Citation9]. PTEs’ ions at high level detected in the biota of QTP were frequently reported. Pb and Hg are dominating PTEs that exhibit 0.079 and 62.1 mg/kg, respectively, in fish samples from this Plateau. In water and soil environments of QTP area, the high concentration of Cr (3429 mg/kg in soil and 2.74 µg/L in water) and Pb (1075.69 mg/kg in soil and 781 µg/L in water) enables them as remarkable pollutants of QTP. Anthropogenic sources for PTEs in QTP are mainly from vehicle exhaust and tire wear [Citation10,Citation11]. Its special geological location causes unique natural landscape, but fragile ecological system, suggesting that mere exposure to high-content pollutants can damage the whole environment of QTP [Citation12,Citation13]. Consequently, PTEs contamination in this area should not be neglected.
Several methods have been developed to risk assessment of PTEs in soils. However, due to technical limitations of current methods, previous studies often focus on the total concentration to evaluate ecological risks of metals and metalloids in soil by using ex-situ methods (e.g. sequential chemical extraction) [Citation14,Citation15]. These techniques involve the active sampling of soil samples followed with laboratory pre-treatment and instrumental analysis, which are complicated and subject to problems of high method detection limits and species instability. The total concentration determined by ex-situ techniques cannot reflect the release ability and mobility of metal/metalloid ions in soils [Citation16,Citation17]. Hence, their bioavailability and labile fraction is not considered in these methods, leading to the inaccurate assessment of PTEs’ biological toxicity and ecological risks [Citation18,Citation19].
To measure the labile fraction, bioavailability and species of PTEs in soils, diffusive gradients in thin-films (DGT) is applied to provide in-situ information from environmental samples. By using DGT for the study of PTEs’ behaviors in soils, a significant positive correlation between DGT concentration of Zn and the phosphorus content was highlighted within the soil matrix. Also, compelling evidence suggested a kinetic interconversion of Cr(VI) and Cr(III), which is primarily driven by the pH, especially the level of hydroxide ions, in soil environments. The As(III) exhibited a propensity for immobilization via interactions with increasing concentration of soil organic matter, thereby reducing its bioavailability [Citation20–22]. The application of the R value revealed the resupply and release ability of labile metals from soil samples. Therefore, this indicator is used to detect the kinetic re-distribution and mobility of PTEs from soil solid particles to porewater [Citation23–26], and can be further performed to distinguish the ‘indigenous’ and ‘exotic’ PTE sources.
In this study, soil samples were collected from different undeveloped area in Qinghai Province, northwestern China. Evaluation methods including geo-accumulation index (Igeo), pollution load index (PLI) and ecological risk index (ERI) were applied to determine the pollution status of PTEs in QTP soils [Citation24,Citation27,Citation28]. To have deep insights into the potential mobility and bioavailability of PTEs, we used DGT device to collect their available fractions in soluble ions released from soil particles. By analyzing above DGT data and R values, environmental impacts of PTE ions on QTP soils from natural or anthropogenic activities can be differentiated, contributing to visualize and identify the short-term and long-term risk levels of various PTEs in the roof of our world.
2. Materials and methods
2.1. Soil sample collection
A total amount of 21 soil samples were collected from diverse natural environments in Qinghai Province. A previous study indicated that soils in Qinghai province presented low risk from heavy metals and metalloids [Citation29]. PTEs from road-side soils are often subjected to vehicle emissions, posing activate and short-term risks to soil quality, while PTEs in river-side soils are mainly transported through surface runoff and atmospheric transmission and precipitation [Citation30]. Therefore, we selected 5 river-side sites and 16 road-side sites in Qinghai with different functions and administrations. Soil surface samples (0–10 cm in depth) at 300 g were collected from different locations that are shown in .
National highways with intensive human activities were selected as road-side soil sampling sites. Sites 1 and 4 were from a highway on the north side of Qinghai Lake in Haibei state. Sites 5–9 were from the highway in Haixi state, sites 10–16 were from the one in Yushu state, site 17 were from the one in Guoluo state. The banks of Heihe and Yellow rivers that are hardly affected by anthropogenic actions were selected as river-side soil sampling sites. Sites 2 and 3 were from the side of the Heihe river, and sites of 18–21 were from the side of the Yellow River in Hainan state. All soil samples were collected by using a sampling grid, mixed and further stored in labeled polyethylene bags before being transported to the laboratory. Soil samples in the laboratory were freeze-dried, gently crushed and grounded in an agate mortar, passed through 100 mesh sieve and preserved in sealing bags for further analysis.
Maximum water holding capacity: Because measurements for maximum water holding capacity of soils are required to determine the addition volume of water for DGT soil deployment treatments. From Luo et al. [Citation31], soil was moistened with MQ water for 4 h until no waterdrops leaked out to obtain saturated soil. The maximum water holding capacity (MWHC) of the soil sample (5 g) was measured as the weight ratio of the saturated soil to dry soil.
2.2. Chemical analysis
To analyze the total concentrations of trace metals, the acid digestion method was used for all soil samples, and three replicates were performed for each soil sample mentioned by Wei et al. [Citation32]. For each sample, around 0.5 g pulverized soil was digested by adding 9 mL HCl and 3 mL concentrated HF, and then boiled gently for 30 ~ 45 min to remove oxidizable substances prior to adding 2.5 mL 70% HClO4 until the generation of white fumes. After cool down, 5 mL of deionized water was added to dispel white fumes and the solution was pipetted through a filter membrane with the pore size of 0.25 µm for instrumental analysis. Inductively coupled plasma mass spectrometry (ICP-MS, PerkinElmer, USA.) was used to analyze the total concentrations of trace metals. The pH values of the soil samples were measured at a soil/water accurate ratio of 1:4 (g/mL) by a pH meter, which need to debug the instrument before testing. Concentrations of Cd, Cr, Ni, Cu, Pb, As and Zn in soil samples were measured by ICP-MS.
2.3. Pollution risk assessment
2.3.1. Geo-accumulation index
The geo-accumulation index (Igeo) is widely used to quantify the PTE pollution in soils and sediments [Citation33,Citation34]. The Igeo is calculated using EquationEquation (1)(1)
(1) :
where Cn is the measured concentration of PTE n in the soil samples and Bn is the geochemical background value of PTE n in soil of Qinghai province on CNEMC [Citation35], while 1.5 is the correction factor to amend the background matrix due to lithologic variations. The contamination levels corresponding to the obtained values of Igeo are categorized into 7 classes listed in [Citation36].
Table 1. The geo-accumulation index of metals and metalloids pollution.
2.3.2. Pollution load index
The pollution load index (PLI) is versatile to quantify the pollution loads in terms of different PTE species and specific zones. PLI was acquired from EquationEquation (2)(2)
(2) and (Equation3
(3)
(3) ) based on the background value documented in CNEMC.
where contamination factor (CF) for PTE n equals its concentration in soil/base value. Contamination factors are derived for different metal species for each sampling site, and all or five (sampling site > 5 at certain zone) highest contamination factors were taken to address the PLI for specific zone.
2.3.3. Ecological risk index
Different from Igeo and PLI (), the Ecological Risk Index (ERI) is based on the principles of sedimentology and is widely used by researchers to assess pollution and the potential ecological risk of PTE in sediments [Citation37]. Er formula is as follows:
where Tr is toxic response factor for PTEs, and this means the level of toxicity and environmental sensitivity of the PTEs (); Cf is a contamination factor, it is the specific value of the concentration of PTE in sediments (CA) compared to its bottom geochemical value in sediments (CB). Er is classified into five levels [Citation34] in .
Table 4. Status of different risk assessment methods for heavy metals.
Table 2. The Tr and background values of metals and metalloid.
Table 3. Levels and extents of the potential ecological risk assessment of metals and metalloids pollution.
2.4. DGT measurements and soil extractions
The DGT piston devices and all resin gels were produced by DGT Research Co., and each resin gel was assembled individually in a DGT device for heavy metal collection, with Chelex-100, N-Methyl-D-glucamine (NMDG), Fe2O3 and ZrO2 resin gels in DGT device, 0.78 mm thick agarose diffusive gels and polyether sulfone (PES) filter membrane were used for the measurements of Cr(III), Cr(VI), As(III), As(V), Cd, Ni, Zn and Cu.
Before DGT deployment, soil samples were mixed well with MQ water at the weight of 70% MWHC, and left in room temperature for 12 h [Citation23]. The wet soil paste was covered on the window of DGT device, and the device was gently pressed into the soil to ensure the sufficient contact between two phases. DGT device was deployed at 24.9 ± 0.1°C for 12 h and three replicates were carried out for each treatment. After deployment, the DGT device was jet washed with MQ water and dried with clean tissue paper. Chelex-100 and NMDG resin gels were collected from the device and placed into 1.5 mL centrifuge tubes with 1 mL 1.0 M HCl for overnight elution. Fe2O3 and ZrO2 resin gels were collected from the device and placed into 1.5 mL centrifuge tubes with 1 mL 1.0 M NaOH for overnight elution. The eluent was diluted 10 times by MQ water to a 1 mL volume system before ICP-MS measurements. The labile concentration of PTEs in soil (CDGT) can be calculated using EquationEquation (7)(7)
(7) and (Equation8
(8)
(8) ):
where Ce (µg/L) is the concentration of the PTEs in the eluent; Vgel (L) is the volume of the binding gel; Vacid (L) is the volume of the eluent; fe is the elution factor for the PTE; M (µg) is the mass accumulated on the binding gel; Δg (0.15 cm) is the thickness of the diffusive gel layer and filter membrane; A (cm2) is the surface area of the gel layer; t (s) is the deployment time for the DGT devices; and D is the diffusion coefficient of the PTEs at 24.9 ± 0.1°C.
The devices were equipped with a 2.54 cm2 exposure window, facilitates are easy to solid-liquid ion exchange. The pistons included a binding gel layer (including Chelex-100 and NMDG), a diffusive gel layer, and a filter membrane.
The ratio (R) of CDGT to Csol (EquationEquation (9)(9)
(9) ) of PTEs indicates the potential resupply ability from the soil solid to the soil solution. The equation is as follows [Citation38]:
The correlation analyses in this study were performed using the software package SPSS statistics 26.0 to do principal component analysis. Pearson’s correlation tests were performed clearly for the independent variables using SPSS statistics 26.0 at a significance level of p < 0.05 and p > 0.01.
3. Results and discussion
3.1. Physicochemical properties of soils in QTP
In Table S1, the physicochemical properties of 21 soil samples are provided. The pH ranging from 7.94 to 9.76 illustrated the neutral or weak alkaline soil conditions in QTP. It is reported that alkaline soil can reduce the mobility of metal ions, leading to large amounts of metals immobilized in the soil of QTP, thereby potentially increasing their environmental risks [Citation39]. Though interactions between PTE ions and soil pH were well-documented in previous studies, small variations in pH value suggested they were not the main driver to affect the mobility of PTEs in QTP soils. In contrary, the level of soil organic matter from 2.97 to 176 g/kg among each soil sample were believed as important influencer on PTEs’ behaviors in QTP. The non-detectable polyaromatic hydrocarbons showed its negligible contributions to the formation of soil organic matters (OMs), while total petroleum hydrocarbons were identified as key constituents for soil OMs to affect the mobility and bioavailability of PTEs. The majority of high-level petroleum hydrocarbons (concentration >6 mg/kg) was found in the road-side soil samples due to the exhaust emissions of vehicles, possibly resulting in mixture contamination and ecological risks of hydrocarbons and PTEs.
Concentrations of 7 different PTEs from all sampling sites were measured in this study. The average concentrations of Cr, Cd, As, Cu, Pb, Zn and Ni were 48.3, 0.11, 14.6, 20.0, 17.9, 61.6 and 27.6 mg/kg, respectively, in the ranges of 22–111, 0–0.3, 7.1–30.2, 11.3–39.1, 9.1–28.2, 31–128 and 14.7–79.5 mg/kg, respectively (Table S1, and S1).
Figure 2. Total (black line) and DGT concentration (red line) patterns of 5 metals and 1 metalloid in soil at each sampling site. Blue line is the DGT concentration of trivalent Cr and red line is the DGT concentration of hexavalent Cr.
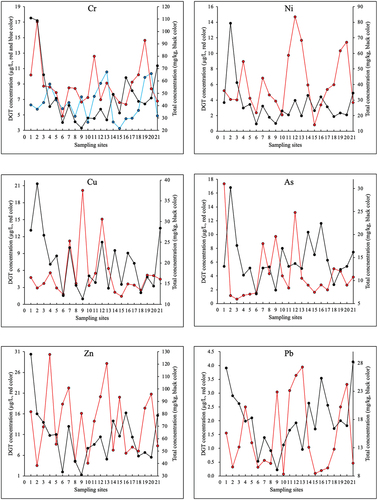
Total contents of these 7 PTEs in surface soils of QTP documented in China National Environmental Monitoring Centre (CNEMC) were compared to those in our study [Citation35], showing that values of Cr and Ni from several sampling sites were higher, while the concentration of Cd were at low level. Highest concentrations of Cr and Ni were found around Qinghai Lake in Haibei state due to the intensive and rapid development of tourism industries. The population located around Qinghai Lake in 2021 was over 12% of Qinghai Province, and the number of tourists was over 6 million per year, which facilitates the transport and content growing of PTEs in this area [Citation40].
3.2. Pollution assessment by total concentration of PTEs
Though general concentrations of the metals and metalloid measured in all sampling sites did not exceed the threshold value in national standards of China, EU and U.S.A. [Citation41], it cannot prove their pollution and risk status were at safe level because soil properties in different area of China were in great variations. Therefore, pollution assessment tools derived from PTE’s total concentration including geo-accumulation index (Igeo), pollution load index (PLI) and ecological risk index (ERI) were utilized to illustrate PTEs’ contamination status and potential risks in QTP. In , the average Igeo values collected for each metal(−loid) followed the order: Cd > As > Ni > Cu > Pb > Zn > Cr. Although the mean value showed that all tested PTEs were at ‘uncontaminated’ level, the standard deviation higher than 0.4 suggested the significant differences in PTEs’ concentrations from different sampling sites.
Table 5. The Igeo values of each metal(−loid) in QTP surface soils.
Except for Pb, Igeo values greater than 0 were found in several soils for other PTEs. Because Igeo can only provide the contamination information of individual PTE, the pollution level and potential risks from PTEs mixture still remained unknown. Additionally, the geochemical background values of metals and metalloid in soils acquired ~30 years ago was too stale to accurately determine the contamination status for present studies.
To investigate PTE mixture’s contamination in soil, PLI was performed for all soil samples and all trace metals. From the results in , soil samples were at moderate contamination degree (where 1 < PLI ≦ 2), while other sites were at uncontaminated degree. Compared to Igeo, the highest value from PLI of 1.02 for As showed its moderate pollution level in QTP soils. PLI values of all tested PTEs higher than 1.0 in Haibei state demonstrated that the metal mixture contamination caused by increasing anthropogenic activities should be taken into consideration. Different from Igeo, PLI method can reduce the effects of extreme results to provide more precise pollution status. However, these two assessment tools depending on PTEs’ total concentration were unable to illustrate their bioavailable and toxic impacts on ecological systems and/or human health.
Table 6. Average PLI values of each metal(−loid) in different states and types of QTP.
Yushu 1; sampling sites from 10 to 13 in Yushu state. Yushu 2; sampling sites from 14 to 16 in Yushu states. Road; sampling sites along the road. River; sampling sites by the Heihe and the Yellow river. PTEs; average PLI of each trace element throughout QTP.
As a consequence, ERI has been widely used to evaluate the ecological risks of metals in soil environments. For each sampling site, the ERI ranging from 13 to 98 showed the low ecological risks from metal mixture (). In contrary, effects from individual Cd and As illustrated high risks throughout QTP area, following the sequence shown as: Cd > As > Ni > Cu > Pb > Cr > Zn, which is similar to the pattern found from Igeo except for the order of Cr and Zn. From previous studies, the high content of As in soils of QTP could be attributed to the As-enriched rocks in this area [Citation42], while Cd in soil was likely to be atmospheric deposition transported from Southern regions of Eastern Asia [Citation43,Citation44].
Table 7. The ERI values for each metal(-loid).
Results from three assessment tools shown above were in a similar pattern, demonstrating that the surface soil of QTP were slightly polluted by Cd and As, and the contamination of both individual and PTE mixture in Haibei state was remarkable. Because the above assessment tools were derived from total concentration, they possessed virtually unanimous conclusions in spite of the difference in calculating method. Although these methods can provide the information about the level of PTEs presented in soils, they failed in evaluating their mobility and release risks of PTEs, leading to the superficial assessments for their ecological risks. Hence, the labile and bioavailable fraction of PTEs should be taken into consideration when evaluating their risks in soil environments.
3.3. Bioavailable versus total concentration of PTEs in soil
PTEs’ total concentrations were wide-applied to assess their pollution and risk status in real environments, but cannot show their dynamic patterns and possible interactions with other chemicals in soils. Consequently, to have a comprehensive view on the PTEs’ mobility and bioavailability in soil environments, their labile concentrations (CDGT) were collected by deployments of DGT devices in all soil samples [Citation45–47].
Speciation measurements of DGT determined the chemical forms of chromium, and gave the labile concentration range of trivalent (3.2 ~ 10.6 µg/L) and hexavalent Cr (4.8 ~ 17.1 µg/L) in QTP soils. The total concentration is the amount of heavy metals in all chemical forms in the soil, and the DGT concentration refers to their bioavailable fraction in the soil. Results from Table S2 showed that the average CDGT of other 6 PTEs were 14.0 ± 7.9 µg/L for Zn, 6.2 ± 3.6 µg/L for Ni, for 5.5 ± 4.6 µg/L for Cu, 4.5 ± 4.3 µg/L for As, 1.5 ± 1.3 µg/L for Pb and 0.2 ± 0.6 µg/L for Cd. In and S2, the distinct patterns of DGT and total concentrations for each PTE at all sampling sites suggested that its bioavailability was insignificantly related to its gross quantity. The highest average labile concentrations of Zn and Cr (including trivalent and hexavalent forms), compared to other PTEs, illustrated their strong mobility and bioavailable contents in surface soils throughout QTP. The difference between spatial patterns of PTEs’ bioavailable fractions and contamination distributions proved that their ecological risks in soil environments were not heavily regulated by their background values, but could be related to particular physicochemical properties at local environments.
The total organic carbon (TOC), phosphorus level and pH were considered in the measurements on labile fraction of PTEs. The range of pH values of sampling sites were less than 2.0, showing weak correlation with the total and labile concentrations of PTEs ( and S3). Phosphorus level in the range of 415–844 mg/kg, showing distinct effects on total and DGT concentrations of PTEs ( and S4). The correlation r2 values higher than 0.47 suggested that phosphorus was positively related to the total concentration of Cr, Ni and Cu. The utilization of chemical fertilizers containing phosphorus and PTEs in QTP was the possible reason to strengthen their correlations [Citation48]. In contrary to the positive correlations of phosphorus-total PTE in soil, its negative impacts on the CDGT of Pb, Ni, Cu and Cr(III) indicated the reduced mobility of these PTEs with high phosphorus level. In soil environments, phosphorus is often found in the form of phosphates that can precipitate with PTE ions to produce insoluble substances, thereby limiting the mobility and available fractions of PTEs [Citation49–51]. Hence, risks assessment for PTEs is required to involve the information about their labile concentrations. Compared to phosphorus content, in spite of the correlation between organic matter (OM) and PTEs’ total concentration, effects of OM were not strong to influence their labile fractions and mobilities ( and S5) [Citation52,Citation53]. Analysis from the results in suggested that current assessment methods derived from pollutant’s total concentration were failed in describing the effects of OM and phosphorus on PTEs’ bioavailability and mobility.
3.4. Short-term and long-term kinetic risk assessment for PTEs by DGT
It is reported that metals’ contents in soil solution are their extractable fraction, while DGT concentration indicates their bioavailable fraction. Therefore, relationship among these three definitions for heavy metals’ concentration often aligns with the following order: total > soil solution > DGT concentration. Since this method is pertinent to bioavailability studies, the ecological risks and dynamic mechanisms of PTE’s mobility in the soil of QTP can be assessed by this tool. The R value is the ratio of available and accessible concentration is the depletion extent of soil solution concentrations at the interface of DGT [Citation54]. So, in this study, R value was performed to determine the short-term and long-term risks of trace elements. It is reported in previous study that R value derived from DGT-based model greater than 0.5 was caused by the large bioavailable fractions, while R < 0.5 indicated the relatively stable state of PTEs [Citation21]. Therefore, PTE’s R value higher than 0.5 is often along with short-term releasing risks. Table S3 presented metals were in the order of Pb > Zn > Cr > Cd > Ni > As > Cu by R values, which is consistent with previous study [Citation55]. The R values of Pb (1.8) and Zn (1.2) confirmed their serious short-term ecological risks. It is documented that the resupply time of Zn is much higher than that of Cd and Ni, and Cu exhibited the weak release ability from solid phase to liquid phase in soil environments [Citation56]. Similar to Zn, our study also evidenced the strong resupply of Pb ions in surface soils, making them two hazardous metals that can be easily accumulated in cells or organisms.
Different from shot-term risk that often exhibit acute effects, long-term risk metals are more likely to be immobilized by soil particles and sensitive to the changes in soil properties such as pH and OM to generate chronic toxicities to soil ecosystems. To understand the difference of short-term and long-term risks, we studied the releasing dynamics of road-side and river-side metals (). Road-side soils in QTP area are main sources of fresh metal ions (R > 0.5) due to intensive human activities and vehicle emissions, whereas metals in river-side soils of QTP are usually long-term naturally deposited (R < 0.5). The significant correlations of Cr, Ni, Cu and Zn indicated the road-side soils were easily affected by PTE mixtures as simultaneous increases were found for their mobility and bioavailability. In contrary, the low significance among 7 PTEs in river-side soils suggested their individual impacts on natural environments. It is noticeable that the presence of Cu and Zn was synergistic in river-side soil, but antagonistic in road-side soils. This is possibly caused by the ageing of Zn ions to produce long-term risks with Cu [Citation57].
Figure 4. Correlations of R value of each PTE from total sampling sites (a), roadside (b) and riverside (c). Blue colors indicate positive correlations and red colors indicate negative correlations. The intensity of the color is proportional to the strength of the correlation. The white asterisk (*) represents significant level: double asterisk (**) indicates P-value < 0.05; single asterisk (*) indicates 0.05 < P-value < 0.1.
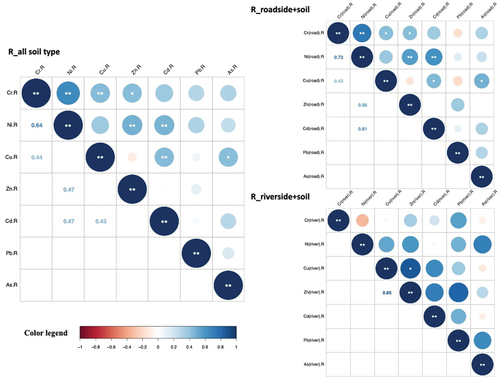
4. Conclusion
Ecological risks from toxic trace elements including heavy metals and metalloids in the soil of Qinghai-Tibet Plateau has attracted considerable attentions in recent years. Although PTE contamination status in QTP area were recently studied, the comprehensive view on their kinetic risks remained uncovered. Therefore, in this study, DGT and relevant model tool was performed to determine the short-term and long-term risks of PTEs by measuring their mobility and bioavailability. Different from conventional assessment methods showing Cd and As were at high contamination level, labile fractions determined by DGT device indicated the strong bioavailability of Cr and Zn in soils of QTP. Due to the slight changes in pH of QTP soils, the effects of this property were not remarkable on the mobility and bioavailability of other tested PTEs. However, from the analysis of CDGT and soil properties, organic matter and phosphorus concentration were two key contributors to affect the behavior of PTEs. Releasing dynamics of PTEs from soil particles in QTP were different, R values indicated the short-term risks of Pb, Zn and Cr due to anthropogenic activities such as traveling activities and vehicles emissions, while As and Cu from natural deposition and transportation in natural environments exhibited long-term risks in QTP soils. This new insight was also confirmed by the correlation analysis of PTEs from road-side and river-side soil, suggesting that the PTEs collected from road-side sampling sites often produced mixture toxicity and short-term risks. Herein, in this study, we confirmed DGT is a promising risk assessment tool based on PTEs’ mobility and bioavailability to facilitate current methods for the regulation of soil contamination and remediation.
Supplemental Material
Download MS Word (750.8 KB)Acknowledgments
The authors would like to thank Beijing University of Technology for technical and laboratory support and thank Lancaster University for providing academic support. HL acknowledges the support from National Natural Science Foundation of China (NSFC). SC acknowledges the support from Environmental Defense Fund, USA.
Disclosure statement
No potential conflict of interest was reported by the author(s).
Supplementary material
Supplemental data for this article can be accessed online at https://doi.org/10.1080/26395940.2024.2357297
Additional information
Funding
References
- Choppala G, Kunhikrishnan A, Seshadri B, et al. Comparative sorption of chromium species as influenced by pH, surface charge and organic matter content in contaminated soils. J Geochem Explor. 2018;184:255–12. doi: 10.1016/j.gexplo.2016.07.012
- Dhal B, Thatoi HN, Das NN, et al. Chemical and microbial remediation of hexavalent chromium from contaminated soil and mining/metallurgical solid waste: a review. J Hazard Mater. 2013;250–251:272–291. doi: 10.1016/j.jhazmat.2013.01.048
- Gao L, Li R, Liang Z, et al. Mobilization mechanisms and toxicity risk of sediment trace metals (Cu, Zn, Ni, and Pb) based on diffusive gradients in thin films: a case study in the Xizhi River basin. South China J Hazard Mater. 2021;410:124590. doi: 10.1016/j.jhazmat.2020.124590
- Campillay Llanos W, Córdova Lepe FD, Moreno Gómez FN. Coexistence, energy, and trophic cascade in a three-level food chain integrating body sizes. Front Ecol Evol. 2022;10. doi: 10.3389/fevo.2022.821176
- Mattas K, Tsakiridou E, Karelakis C, et al. Strengthening the sustainability of European food chains through quality and procurement policies. Trends Food Sci Technol. 2022;120:248–253. doi: 10.1016/j.tifs.2021.11.021
- Yan W, Hamid N, Deng S, et al. Individual and combined toxicogenetic effects of microplastics and heavy metals (Cd, Pb, and Zn) perturb gut microbiota homeostasis and gonadal development in marine medaka (Oryzias melastigma). J Hazard Mater. 2020;397:397. doi: 10.1016/j.jhazmat.2020.122795
- Choppala G, Bolan N, Lamb D, et al. Comparative sorption and mobility of Cr(III) and Cr(VI) species in a range of soils: implications to bioavailability. Water Air Soil Pollut. 2013;224(12):1699. doi: 10.1007/s11270-013-1699-6
- Huang Y, Zheng J, Wang L, et al. Sensitive detection of chloramphenicol based on Ag-DNAzyme-mediated signal amplification modulated by DNA/Metal ion interaction. Biosens Bioelectron. 2018;127:45–49. doi: 10.1016/j.bios.2018.12.016
- Deyong L, Xiaodian J, Wei G, et al. Tectonic uplift along the northeastern margin of the Qinghai–Tibetan Plateau: Constraints from the lithofacies sequence and deposition rate of the Qaidam Basin. Tectonophysics. 2022;827:229279. doi: 10.1016/j.tecto.2022.229279
- Windom Zachary W, Ajith P, Bartlett Rodney J. Benchmarking isotropic hyperfine coupling constants using (QTP) DFT functionals and coupled cluster theory. J Chem Phys. 2022;156(9). doi: 10.1063/5.0069928
- Wang S, Che Y, Xinggang M. Integrated risk assessment of glacier lake outburst flood (GLOF) disaster over the Qinghai–Tibetan Plateau (QTP). Landslides. 2020;17(12):2849–2863. doi: 10.1007/s10346-020-01443-1
- Bitna K. Ecological system levels of risk factors for intimate partner homicide perpetration and victimization: a three-level meta-analysis. Trauma Violence Abuse. 2022;24(4):2082–2096. doi: 10.1177/15248380221082154
- Hao Z, Sanyi T. Bifurcation dynamics on the sliding vector field of a Filippov ecological system. Appl Math Comput. 2022;424:424. doi: 10.1016/j.amc.2022.127052
- Tiancheng N, Changchun Z, Jinhe P, et al. Study on the occurrence of rare earth elements in coal refuse based on sequential chemical extraction and Pearson correlation analysis. Min Metall Explor. 2022;39(2). doi: 10.1007/s42461-022-00542-y
- Yinjiao S, Xuan L, Yang T, et al. Mercury speciation in various coals based on sequential chemical extraction and thermal analysis methods. Energies. 2021;14(9):2361. doi: 10.3390/en14092361
- Diana A, Thomas R, Alves Vítor D, et al. Chitin-glucan complex hydrogels: optimization of gel formation and demonstration of drug loading and release ability. Polymers. 2022;14(4):785. doi: 10.3390/polym14040785
- Snehal Ashokrao H, Sekhar Kanaparedu PC, Deepak Kumar S, et al. Supramolecular glycolipid-based hydro-/organogels with enzymatic bioactive release ability by tuning the chain length and headgroup size. ACS Biomater Sci Eng. 2022;8(3):1103–1114. doi: 10.1021/acsbiomaterials.1c01510
- Ning S, Qiang L, Jinhu W, et al. Liu Rutao, Gao Canzhu. Probing the biological toxicity of pyrene to the earthworm Eisenia fetida and the toxicity pathways of oxidative damage: A systematic study at the animal and molecular levels. Environ Pollut. 2021;289:289. doi: 10.1016/j.envpol.2021.117936
- Peng G, Qi L, Weizhen Z, et al. Biological toxicity of fresh and rotten algae on freshwater fish: LC50, organ damage and antioxidant response. J Hazard Mater. 2021;407:407. doi: 10.1016/j.jhazmat.2020.124620
- Qiuyun X, Li G, Wenqi P, et al. Assessment of labile Zn in reservoir riparian soils using DGT, DIFS, and sequential extraction. Ecotoxicol Environ Saf. 2018;160:184–190, 0147–6513. doi: 10.1016/j.ecoenv.2018.05.039
- Bo G, Li G, Dongyu X. New insight for the diffusion–resupply kinetics of Cr(VI) in contaminated soil using DGT/DIFS. Ecotoxicol Environ Saf. 2022;242:242. doi: 10.1016/j.ecoenv.2022.113946
- Alberto P, Beatriz P, Del Pino P, et al. Monodisperse superparamagnetic nanoparticles separation adsorbents for high-yield removal of arsenic and/or mercury metals in aqueous media. J Mol Liq. 2021;335:335. doi: 10.1016/j.molliq.2021.116485
- Chenxu L, Yu T, Khan Zulfiqar A, et al. Mitigation of tribocorrosion of metals in aqueous solutions by potential-enhanced adsorption of surfactants. Friction. 2023;11(5):801–819. doi: 10.1007/s40544-022-0692-8
- Rui C, Tao G, Nuo C, et al. Application of DGT/DIFS to assess bioavailable Cd to maize and its release in agricultural soils. J Hazard Mater. 2020;411:411. doi: 10.1016/j.jhazmat.2020.124837
- Min Z, Cai L, Liyuan Y, et al. Application of DGT/DIFS combined with BCR to assess the mobility and release risk of heavy metals in the sediments of Nansi Lake, China. Environ Geochem Health. 2020;42(11):3765–3778. doi: 10.1007/s10653-020-00638-8
- Xu D, Gao B, Peng W, et al. Application of DGT/DIFS and geochemical baseline to assess Cd release risk in reservoir riparian soils, China. Sci Total Environ. 2019;646:1546–1553. doi: 10.1016/j.scitotenv.2018.07.262
- YangGuang G, HongHui H, ShiJun J, et al. Appraising ecotoxicological risk of mercury species and their mixtures in sediments to aquatic biota using diffusive gradients in thin films (DGT). Sci Total Environ. 2022;825:825. doi: 10.1016/j.scitotenv.2022.154069
- Yuanyuan Y, Sisi L, Runmei W, et al. Diffusive gradients in thin films (DGT) probe for effectively sampling of per- and polyfluoroalkyl substances in waters and sediments. J Environ Sci. 2022;121:90–97. doi: 10.1016/j.jes.2021.09.003
- Yang D, Chen H, Sun H, et al. Validation and assessment of diffusive gradients in thin-films (DGT) technique for measuring nutrients in Taihu Lake water with algae bloom. Bull Environ Contam Toxicol. 2022;108(5):943–948. doi: 10.1007/s00128-022-03470-1
- Zu C, Song X, Li Y, et al. The accumulation of heavy metals in agricultural land in Qinghai province, China. Environ Sci Pollut Res. 2018;23:1135–1139.
- Luo J, Cheng H, Ren JH, et al. Mechanistic insights from DGT and soil solution measurements on the uptake of Ni and Cd by radish. Environ Sci Technol. 2014;48(13):7305–7313. doi: 10.1021/es500173e
- Wei X, Gao B, Wang P, et al. Pollution characteristics and health risk assessment of heavy metals in street dusts from different functional areas in Beijing, China. Ecotoxicol Environ Saf. 2015;112:186–192. doi: 10.1016/j.ecoenv.2014.11.005
- Tang R, Ma K, Zhang Y, et al. The spatial characteristics and pollution levels of metals in urban street dust of Beijing. China Appl Geochem. 2013;35(4):88–98. doi: 10.1016/j.apgeochem.2013.03.016
- Li F, Zhang J, Jiang W, et al. Spatial health risk assessment and hierarchical risk management of mercury in soils from a typical contaminated site, China. Environ Geochem Health. 2017;39(4):923–934. doi: 10.1007/s10653-016-9864-7
- CNEMC (China National Environmental Monitoring Centre). The Background Values of Chinese Soils. Beijing. China: Environmental Science Press of China; 1990.
- Li F, Xiao M, Zhang J, et al. Spatial distribution, chemical fraction and fuzzy comprehensive risk assessment of heavy metals in surface sediments from the Honghu Lake, China. Int J Environ Res Public Health. 2018;15(2):207. doi: 10.3390/ijerph15020207
- Lin Q, Liu E, Zhang E, et al. Spatial distribution, contamination and ecological risk assessment of heavy metals in surface sediments of Erhai Lake, a large eutrophic plateau lake in southwest China. Catena. 2016;145:193–203. doi: 10.1016/j.catena.2016.06.003
- Harper MP, Davison W, Tych W. DIFS—a modelling and simulation tool for DGT induced trace metal remobilisation in sediments and soils. Environ Model Softw. 2000;15(1):55–66. doi: 10.1016/S1364-8152(99)00027-4
- Guan DX, Zheng JL, Luo J, et al. A diffusive gradient in thin films technique for the assessment of bisphenols desorption from soils. J Hazard Mater. 2017;331:321–328. doi: 10.1016/j.jhazmat.2017.02.053
- Xu DY, Gao B, Peng WQ, et al. Application of DGT/DIFS and geochemical baseline to assess Cd release risk in reservoir riparian soils, China. Sci Total Environ. 2019;646:1546–1553. doi: 10.1016/j.scitotenv.2018.07.262
- Shui Qiu J, Lie Yi X, Yun Liu B. Comparison research on standards of foreign country and national standard of China for marine fire extinguisher. Adv Mater Res. 2013;2606(781–784):2803–2808.
- Mai Duc D, Tran Thi Viet N, Nguyen Thi L, et al. Adsorption behavior and mechanism of As(V) on magnetic Fe3O4–graphene oxide (GO) nanohybrid composite material. Anal Sci Int J Jpn Soc Anal Chem. 2022;38(2):427–436. doi: 10.1007/s44211-022-00064-z
- Jiang L, Meng Z, Yujie Z, et al. Mechanism of mercapto-modified palygorskite in reducing soil Cd activity. Sci Total Environ. 2022;857(2):159372. doi: 10.1016/j.scitotenv.2022.159372
- Elisa A, Isvett Josefina F-S, Dennis B, et al. Sublethal and lethal Cd toxicity in soybean roots specifically affects the metabolome, Cd binding to proteins and cellular distribution of Cd. J Hazard Mater. 2023;442:442. doi: 10.1016/j.jhazmat.2022.130062
- Burkitt LL, Mason SD, Dougherty WJ, et al. The ability of the DGT soil phosphorus test to predict pasture response in Australian pasture soils – a preliminary assessment. Soil Use Manage. 2016;32(1):27–35. doi: 10.1111/sum.12230
- Hooda PS, Zhang H, Davison W, et al. Measuring bioavailable trace metals by diffusive gradients in thin films (DGT): soil moisture effects on its performance in soils. Eur J Soil Sci. 1999;50(2):285–294. doi: 10.1046/j.1365-2389.1999.00226.x
- Gao Y, Leermakers M, Pede A, et al. Response of diffusive equilibrium in thin films (DET) and diffusive gradients in thin films (DGT) trace metal profiles in sediments to phytodetritus mineralisation. Environ Chem. 2012;9(1):41. doi: 10.1071/EN11075
- Bould C, Thomas WDE, Tolhurst JAH. Cover crops in relation to soil fertility and fruit tree nutrition: the effect of grass on the uptake of total and fertilizer phosphate by malling i rootstocks. J Hortic Sci. 2015;29(4):301–309. doi: 10.1080/00221589.1954.11513822
- Miguel N, Gonzalo D, Ana L. Comparative study on bubbling and shearing techniques for the crystallization of xylitol in TES systems. Results In Eng. 2023;17:17. doi: 10.1016/j.rineng.2023.100909
- India’s National Hydroelectric Power Corporation. OM metals to equip 520-mw parbati 3, 231-mw chamera 3. Hydro Review Worldwide: HRW. 2007;15(5).
- Saeedi M, Li LY, Grace JR. Desorption and mobility mechanisms of co-existing polycyclic aromatic hydrocarbons and heavy metals in clays and clay minerals. J Environ Manage. 2018;214:204–214. doi: 10.1016/j.jenvman.2018.02.065
- Shankar E, Stalin John MR, Devanathan C, et al. Burnishing of Al (SiC) p metal matrix composites using TiAlN coated roller. J Mech Sci Technol. 2017;31(7):3475–3479. doi: 10.1007/s12206-017-0636-9
- Sidorenko DA, Zaitsev AA, Kirichenko AN, et al. Modification of Fe–Cu–Co–Sn–P metal matrix with various forms of carbon nanomaterials. Izv vuzov, Porošk metall funkc pokryt (Proc Higher Schools Powder Metall Funct Coat). 2015;0(3):61. doi: 10.17073/1997-308X-2013-3-61-66
- Zhang H, Davison W, Knight B. In situ measurements of solution concentrations and fluxes of trace metals in soils using DGT. Environ Sci Technol: ES&T. 1998;32(5):704–710. doi: 10.1021/es9704388
- Wu Z, Wang S, Ji N. Phosphorus (P) release risk in lake sediment evaluated by DIFS model and sediment properties: a new sediment P release risk index (SPRRI). Environ Pollut. 2019;255(Pt 2):113279. doi: 10.1016/j.envpol.2019.113279
- Laurence P, David S, Anne B, et al. Photo-reversible solid to liquid transition of azobenzene containing polymers: Impact of the chemical structure and chain length. Eur Polym J. 2022;174:174. doi: 10.1016/j.eurpolymj.2022.111297
- Chaowei L, Jingjing L, Xiaojuan S, et al. Constructing protective layer on electrode for ultra-enduring Zn ions batteries. Appl Surface Sci. 2022;605:605. doi: 10.1016/j.apsusc.2022.154660