ABSTRACT
Pollutants in aquatic environment tends to alter the community composition and activities of biofilm cells. The stress induced by these pollutants leads to the production of excessive reactive oxygen species (ROS) which play a key role in the degradation of pollutants in aquatic environment. This mini-review is aimed at forecasting the efficiency of the use of natural freshwater microalgae biofilm in the treatment of water resulting from mining activities. Moreover, it summarises the concept of mining by concisely stating the stages involved in mining and how the pollutants produced during mining can cause deleterious effects to aquatic flora and fauna. Environmental issues resulting from mining activities and how they hindered the progress of biodiversity were concisely discussed. Freshwater microalgae play an important role in the mitigation of the impact of pollution resulting from mining activities. The process of pollutants removal in freshwater by microalgae biofilm is by degradation and biosorption. However, it is an unestablished fact that the extracellular polymeric substances (EPS) were responsible for the biosorption of heavy metals from mining wastewater. It is also important to know the kinetic model that best explains the chemical and physical reactions of heavy metal absorption by the surface of microalgae biofilm.
Introduction
Mining is the main economic activity of many developing countries. Mining was among the major source of export in Nigeria before 1970 (Eludoyin et al. Citation2017). The overdependent on oil resources have intensified artisanal mining practice in the country due to lack of government will, intervention and implementation of available regulations. Mining in Nigeria is done without considering the impacts on the environment, and in most cases, reclamation is never a stage in mining process (Stewart Citation2020). Mining operation be it small or large scale is inherently disruptive to the environment, producing enormous quantities of waste that can have a deleterious impact for decades. The risk of pollution from mining sites lasts even after the cessation of mining activities. In all sites where mining activities exist, environmental problems are present.
These problems manifest as degradation of forest and loss of biodiversity, soil contamination, air pollution, surface and groundwater pollution, noise and vibrations, deterioration of natural drainage system, natural land degradation, negative impact on terrestrial and aquatic ecosystems, socio-economic and human health. The worsening environment caused by mining occurs mainly as a result of unsuitable and wasteful working practices and rehabilitation measures. Hence, protecting water quality is an urgent environmental challenge at mining and mineral processing sites (Ugya et al. Citation2018).
Many technologies have been employed in the treatment of mining wastewater, among which include desalination, clarification, evaporation and crystallization, disinfection, filtration, ion exchange, membrane separation, package plants and biological techniques (Puyol et al. Citation2017). These technologies are cost-ineffective and very difficult to use under field conditions, hence in such a situation, there is an urgent need to study natural, simple and cost-effective techniques for control (Guo et al. Citation2019). The use of natural freshwater microalgae biofilm for the clean-up of water resulting from mining activities is an alternative to conventional and other biological methods due to its cost-effectiveness, the requirement of low technological know-how and high efficiency of freshwater microalgae biofilm to remove nutrients, heavy metals, organic and inorganic wastewater from wastewater (Abdel-Raouf et al. Citation2012).
Natural freshwater microalgae biofilm encompasses several syntrophic groups of microorganisms in association with the inorganic surfaces. The member cells forming a freshwater biofilm become surrounded by a slimy extracellular matrix which is composed of extracellular polymeric substances (EPS) (Arunasri and Mohan Citation2019). The components of the EPS signify the community routine of all the member microorganism in the freshwater microalgae biofilm and have a three-dimensional structure because the EPS is produced by the accumulation of polysaccharide, proteins, lipid and deoxyribonucleic acid (DNA) of each of the members’ microorganism forming the natural freshwater microalgae biofilm (López et al. Citation2010).
The microorganisms developing freshwater microalgae biofilm are physiologically different from other planktonic cells of a similar organism floating in a freshwater environment (Pikoli et al. Citation2019). Natural biofilm consists of different types of microorganisms which could either feed autotrophically or heterotrophically. The autotrophic organisms present in natural biofilms consist of microalgae and cyanobacteria, whereas heterotrophic organisms include bacteria, fungi and protist (Xuemei et al. Citation2010). These organisms form a natural biofilm and accumulate on the surface of an artificial or natural substrate which are surrounded by EPS (Ugya et al. Citation2020). The EPS of natural biofilm is the reason why biofilm is able to resist environmental stress, thus causing the degradation of pollutants in aquatic environment (Han et al. Citation2017). This mini-review is thereby aimed at forecasting the efficiency of the use of natural freshwater microalgae biofilm in the treatment of water resulting from mining activities.
The concept of mining
Mining can be defined as the process by which minerals resulting from geological processes are extracted from the lithosphere (Ugya et al. Citation2018, Ugya et al. Citation2019). These economically valuable ores are a combination of different materials including metals, coal, oil shale, gemstones, limestone, chalk, dimension stone, rock salt, potash, gravel and clay (Ugya et al. Citation2018). The process of mining involves the extraction of non-renewable resources such as coal, crude oil, water and natural gas and is independent of material resulting from agronomic activities or materials created in the laboratory (Emmanuel et al. Citation2018). The unanswered question about mining operations is its negative environmental impact which has led to the creation of different laws aimed at decreasing the negative aspect of mining on the environment (Ngole-Jeme and Fantke Citation2017). The stages involved in mining activities include prospecting, exploration, development, exploitation and reclamation as illustrated in Figure .
The prospecting stage of modern-day mining activities is aimed at discovering and searching for valuable mineral deposits or ore. This stage is important because the minerals may be located beneath or in the surface of the earth (Alavi Citation2020). The methods adapted in prospecting of mining site can either be direct prospecting method or the indirect prospecting method.
The direct prospecting method is used in the exploration of valuable minerals or ores found on the surface of the earth. This method involves the use of visual examination and geologic techniques such as aerial photography, geologic mapping, structural assessment of an area and microscopic studies of sample (Griffith Citation1960). The indirect prospecting method is a tool that is used for the discovery of valuable ores and materials deposited beneath the earth. The indirect prospecting method used for the detection of materials is geophysical techniques including physical measurement of gravitational, seismic, magnetic, electrical, electromagnetic and radiometric variables of the earth (Day-Lewis et al. Citation2017).
Exploration is the second stage of mining which involves the estimation of the size and value of mineral deposits. This important stage is aimed at determining the supposed economics of the ore deposit in order to know if there is the need for further investment (Sonter et al. Citation2018). This stage of mining activity involves the measurement of the fiscal viability, technical and monetary danger, as well as the strength of the project. This is the stage where decision is made on continuity of the mining activity. This stage also involves the planning to evaluate the retrievable portion of the deposit (the metallurgy and ore), its economic and marketability potential, and engineering concerns, as well as an investigation of the proposed mine from the initial excavation all the way through to the recovery of land. Another issue deserving consideration is that more waste than ore is mined during the life of a mine, so waste elimination and placement are key burden to the mining operator, and as such, comprehensive characterization of the waste material formed is a crucial part of the geological exploration program for a mining operation (Considine et al. Citation2017). The techniques used in exploration during mining include chemical, metallurgical, X-ray, spectrographic or radiometric evaluation techniques which are meant to enhance the investigator’s knowledge of the mineral deposit (Singer and Kouda Citation1999).
Development is the third stage of mining activities which involves the preparation for the exploitation of mineral deposits. Before exploitation of surface mining can occur, the mineral deposits are accessed by stripping the soil or rock covering the mineral deposits while deep soil excavation techniques are used to expose the mineral deposits during sub-surface mining. The most common method of mining in Nigeria is surface mining (without petroleum and natural gas) (Obiadi et al. Citation2016). The developmental work that must be done before the stripping of soil/rock or the process of excavation include acquiring water and mineral rights, buying surface lands, arranging for financing, and preparing permit applications and an environmental impact statement (EIS). Other developmental work pertaining to the mining process include the provision of access roads, power sources, mineral transportation systems, mineral processing facilities, waste disposal areas, offices and other support facilities (Maier et al. Citation2014).
Exploitation is the fourth stage of mining involving the actual exploration of valuable minerals in quantity from the earth. This stage is initiated by the movement of mining equipment to the site and commencement of mining activities until the mineral mining is no longer economically viable (Considine et al. Citation2017). The two categories of materials targeted during the exploitation stage include the placer and lode deposits. The placer deposits are valuable minerals that is formed as a result of hydrologic weathering and surface weathering, e.g. alluvial, colluvial, eluvial, beach and paleo placers (Haldar Citation2013), while lode deposits are metalliferous ore that are found embedded in the cracks or layer of rocks (Groves and Foster Citation1991).
Both the placer or lode deposits are mined by both surface and underground methods. Rare earth element (cerium (Ce), dysprosium (Dy), erbium (Er), europium (Eu), gadolinium (Gd), holmium (Ho), lanthanum (La), lutetium (Lu), neodymium (Nd), praseodymium (Pr), promethium (Pm), samarium (Sm), scandium (Sc), terbium (Tb), thulium (Tm), ytterbium (Yb) and yttrium (Y)) and uranium can be mined using in-situ leaching method, which is a method that do not depend on surface or underground digging (Xie et al. Citation2014). The requirement for extracting target minerals by this technique is that they should be soluble, for instance, potash, potassium chloride, sodium chloride, and sodium sulfate, are soluble in water, but minerals, such as copper minerals and uranium oxide, will require acid or carbonate solutions to dissolve (Mhlongo et al. Citation2018).
Surface mining is performed by stripping surface vegetation, dirt, and, layers of bedrock for the purpose of getting the ore deposits. This is achieved by different methods such as open-pit method, quarrying, strip mining, and mountaintop removal. Open-pit method is the type of surface mining commonly called open cast mining, and this type of surface mining involves the extraction of valuable minerals from the borrow without the use of the tunnel because the kind of ores (sand, cinder and gravel) extracted using this method are always near the surface (Zendehboudi and Bahadori Citation2017). Quarrying is similar to open-pit mining but it deals with sand, stone and clay. Strip mining is a type of surface mining usually called the overburden removal that is used for the mining of coal and lignite.
This mining technique involves the removal of long strip of overlying soil or rock covering the ore/seams underneath (Cole Citation1999). The mountaintop removal is a type of surface coal mining technique that occurs at the summit ridge of a mountain (Hendryx et al. Citation2019). The final stage of mining activities is reclamation which involves the recontouring, revegetation and restoring of water and land values. The process of restoration should begin after the excavation process is initiated (Ezeokoli et al. Citation2020). Since the concern of mining industry is to maintain sustainability, it is important to address some issues during reclamation in order to sustain the economic and environmental needs of the present and future generation (Virgone et al. Citation2018). These issues include safety of mine site, restoration of land surface and water and change of land use after mining (Virgone et al. Citation2018).
Environmental issues resulting from mining activities
Environmental issues resulting from mining activities are as a result of the direct or indirect impact of mining practices. These issues are of local, regional and global concern and arose from the emission during mining activities (Navarro et al. Citation2004). These emissions led to environmental issues such as sinkholes, erosion, soil contamination, water contamination (surface and ground water) (Figure ), loss of biodiversity and deterioration of air quality (Haddaway et al. Citation2019). The process of mining is associated with soil degradation and erosion leading to the destruction of large area of land. Erosion and degradation are as a result of mining activities such as open pit and overburden mining, construction, etc. (Ćwieląg-Drabek et al. Citation2020). Soil degradation is as a result of the contamination of soil by the chemical pollutants produced during mining activities (Eludoyin et al. Citation2017). One major effect of soil erosion resulting from mining activities is the link with water resources contamination, sinkhole and decline in biodiversity (Harfoot et al. Citation2018). The pollutants produced during mining activities can seep into aquifers and pollute underground water or erode into nearby surface water leading to increase in pollution, stream sedimentation, clogging of water ways and loss of biodiversity (Tarras-Wahlberg et al. Citation2001). During mining activities such as blasting, excavation and transportation, particulate and gases matter are dispersed by wind to the atmosphere causing air pollution (Yu et al. Citation2017). This air pollution tends to affect human health, global climate and the environment (water, soil and wildlife) (Almalki Citation2019).
The impact of mining activities on biodiversity
The modification of habitat resulting from mining activities is associated with disturbance and persistence adverse health effects on both aquatic and terrestrial organisms (Taylor-Brown et al. Citation2019). Damaging of the original site is more detrimental to biodiversity because the pollutants produced during mining activities are toxic and can directly or indirectly affect biodiversity (Prach and Tolvanen Citation2016).
The modification of the habitat is associated with change in pH and temperature which in turn causes disturbances to the surrounding community. Cosmopolitan species are likely to adapt to the change in pH and temperature while endemic species are negatively affected due to slight variation in the condition of their habitat which increase the risk of extinction (Davidson et al. Citation2017).
The mobility and bioavailability of pollutants produced during mining activities determine the effect of the pollutants on biodiversity (Wuana and Okieimen Citation2011). The less-mobile pollutants remain in the mining site, whereas highly mobile pollutants move into nearby environment or are been taken up by organism (Sharma and Bhattacharya Citation2017).
Some pollutants produced during mining activities tend to be bioavailable while others evolve in the environment. This difference in bioavailability led to variation in the level of toxicity to organisms (Tchounwou et al. Citation2012). The ability of some pollutants (heavy metals) produced during mining activities to bioaccumulate also determines the effect of these pollutants on biodiversity. This is because heavy metals tend to bioaccumulate across the food chain to the level that it causes toxic effects to organisms. On the other hand, contaminants that do not bioaccumulate tend to degrade and completely disappear from the contaminated zone especially with low concentration (Jain and Das Citation2017).
Impact of mining activities on aquatic organisms
Aquatic biodiversity is impacted by mining in different ways. One of which is direct poisoning; this happens when contaminants are bioavailable in the water and/or freely mobile in the sediment. Contaminants can physically affect aquatic organisms. Example including high concentrations of suspended sediment in a stream tends to limit penetration of light which may diminish algal biomass. Metal oxide deposition limits biomass by coating algae or their substrate, thus preventing colonization. Also, temperature, rainfall, pH, salinity and metal concentrations in a stream can in the long run affect aquatic communities. pH or temperature changes affect metal solubility making it more bioavailable for uptake by organisms.
It is noteworthy that metal contaminants persist in the habitat for a very long time up to 90 years after pyrite mine closure. Mine drainages cause distress in algal communities leading to decreased primary productivity. Both physical and chemical changes in aquatic environment tend to affect diatoms population. In the extreme, the diatom population may become extinct. Some macroinvertebrates like aquatic insect and crustacean communities are altered due to mining resulting in a low trophic completeness and may become unbalanced with predators dominating their community. Macroinvertebrate diversity may persist if sensitive species are replaced with tolerant ones (Huang et al. Citation2010).
Pollution in freshwater environment resulting from mining activities
The pollutants produced during mining activities are in the form of organic and inorganic. Biological pollutants are divided into two classes based on polarity as hydrophilic and hydrophobic organic pollutants (Schweitzer and Noblet Citation2018). The polar organic contaminant that is soluble in water is referred to as hydrophilic pollutants while the non-polar and insoluble organic contaminant is known as hydrophobic organic contaminant (Nanny and Ratasuk Citation2002). Hydrophilic contaminants are organic pollutants that do not readily affect freshwater ecosystem owing to their non-persistence nature and ability to dissolve in water, common example of hydrophilic contaminant includes organic pollutants with relatively small alkyl group such as alcohol and carboxylic acids (CH3, C2H5, C3H7 and C4H5). Another important example is methyl tertiary butyl ether (MTBE) (Qin et al. Citation2010). Hydrophobic contaminants are the most worrisome organic contaminants due to their persistence’s nature and inability to dissolve in water, example of these pollutants includes dichlorodiphenyltrichloroethane (DDT), polychlorinated biphenyls (PCBs), polybrominated diphenyl ethers (PBDEs), polychlorinated dibenzodioxins (PCDDs), polychlorinated dibenzofurans (PCDFs), polyaromatic hydrocarbons (PAHs), benzene toluene, ethylbenzene and xylene (BTEX) (Jaffé Citation1991) (Table ). Inorganic pollutants include heavy metals, phosphorus compounds, nitrogen compounds, halide compound, sulphide compounds, etc. (Baykoucheva Citation2015) (Table ). Heavy metals are the most persistent inorganic contaminants due to their non-biodegradable capacity (Tchounwou et al. Citation2012). Nevertheless, these elements might be found in a very low concentration in the environment but they tend to be detrimental to aquatic flora and fauna due to their ability to bioaccumulate along the food chain. Phosphorus and nitrogen containing compounds are also worrisome in the environment due to their contribution in eutrophication which leads to the growth of harmful algae (Heisler et al. Citation2008).
Table 1. The effect of organic and inorganic contaminant resulting from mining activities.
Harmful microalgae can be very dangerous to freshwater habitat due to the formation of algae blooms (Mohamed Citation2018). Microalgae blooms vary in colour from green, yellow or brown colour depending on the species of microalgae involved (Wells et al. Citation2015). This bloom is caused as a result of excessive utilization of nutrients by harmful microalgae particularly in slow moving water bodies such as lake and ponds (Mohamed Citation2018).
This bloom produced by freshwater microalgae is extremely toxic to freshwater biota because they tend to block the gills of fishes and other freshwater micro invertebrate and also depletes the available dissolve oxygen in freshwater habitat leading to the death of freshwater organisms (Schmale et al. Citation2019).
Blooms can also make an ecosystem to be unsustainable because they tend to be poisonous to the smaller fishes and other filter feeder organisms that feed on them and this toxicity can move across the food web resulting into the death of macro plants in freshwater environment (Paerl Citation2018).
Harmful microalgae can also affect aquatic productivity because they prevent sunlight energy from penetrating deep into other freshwater zones leading to the reduction in the diversity of organisms in the freshwater habitat (Heisler et al. Citation2008). The prevention of sunlight by harmful microalgae could be based on the decolouration of freshwater habitat by harmful microalgae or due to the formation of thick film on the surface of freshwater by freshwater microalgae (Wang et al. Citation2017).
Harmful microalgae bloom also affects the mobility of freshwater fauna such as coral and can also affect other freshwater flora from having access to sunlight for photosynthesis because they submerged this freshwater flora and also serve as a shade, thereby preventing sunlight energy from reaching the organisms. Harmful microalgae are able to produce micro toxins that have detrimental effects on freshwater biota particularly fishes and other micro- and macroinvertebrates (Stauffer et al. Citation2019).
Formation and composition of natural freshwater microalgae biofilm
Freshwater microalgae are microorganisms that are adapted to freshwater environment. They are unicellular and free living organism that ranges in size from 4 to 178 µm (Ugya et al. Citation2020). Microalgae are able to utilize light, carbon dioxide and water for the process of photosynthesis to generate the energy needed for metabolic activities. The growth of microalgae also depends on major nutrients such as nitrogen and phosphorus (Zullaikah Citation2019).
Natural freshwater microalgae biofilm is formed by the attachment of free-floating microalgae to organic or inorganic substrate (Donlan Citation2002). The initial freshwater microalgae that form the biofilm are able to attach to the substrate at first by the help of weak Van der Waals force of attraction and hydrophobic effect before being attached permanently with increasing density of the freshwater microalgae (Davey and O'Toole Citation2000). The process of biofilm formation by freshwater microalgae can be divided into five stages. The stages of freshwater microalgae biofilm formation include initial attachment, permanent attachment, initial maturation, permanent maturation and dispersion (Muhammad Citation2020).
The stages of natural freshwater biofilm represented in Figure depict that during the initial and permanent attachment stages, the EPS was not formed due to the visibility of the substrate, though the number of freshwater microalgae on the substrate during the initial attachment stage is fewer than the permanent attachment stage (Miranda et al. Citation2017). The EPS was formed during the initial maturation, permanent maturation and dispersion stage, despite the thickness of the natural freshwater biofilm of the permanent maturation and dispersion stages is thicker than the initial maturation stage (Roostaei et al. Citation2018). The reason why higher biomass of the freshwater microalgae were found during the maturation and dispersion stages when compared to the attachment stage is owing to the fact that during the attachment stage, some freshwater microalgae were not able to anchor to a substrate instead it attached to the EPS during the maturation and dispersion stages (Roostaei et al. Citation2018). The dispersion stage is the final stage of freshwater biofilm microalgae formation and is the stage in which the freshwater microalgae biofilm is established and may undergo alteration in form and size. Enzymes such as dispersin B and deoxyribonuclease play important role in the dispersal of freshwater microalgae biofilm because they have the ability to degrade the EPS (López et al. Citation2010).
Figure 3. The process of natural freshwater microalgae biofilm formation (a) initial attachment (b) permanent attachment (c) initial maturation (d) permanent maturation (e) dispersion.
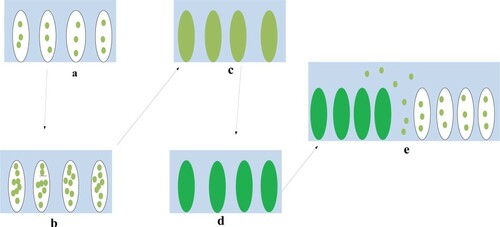
Freshwater microalgae biofilms are usually found naturally on solid substrates submerged in aquatic environment, although they can sometimes form a floating mat on the surfaces of aquatic habitat. Microalgae biofilm often grow to macroscopic level but tend to contain diverse kinds of microorganisms including bacteria, archaea, protozoa and fungi with each group of organism playing a particular metabolic role (Besemer Citation2015).
Factors influencing natural microalgae biofilm formation
There are no much information on the factors that influences the formation and structure of natural freshwater microalgae biofilms, this is because the formation of natural freshwater microalgae biofilm is depended on different parameters and interactions among the microbes involved in the biofilm formation (Mantzorou and Ververidis Citation2019). In order to determine the mechanism involved in the regulation of pollutants in natural environment by freshwater natural biofilm, it is crucial to know the most influential parameter for the formation of natural freshwater microalgae biofilm (López and Soto Citation2019). This mini-review is structured specifically to consider the role of nutrients, light intensity, temperature, pH, inorganic carbon, the role of other microbes, growth substrate, water flow and nature of EPS formed. This mini-review will not consider the selection of microalgae strain as part of the factors that influences the formation of natural microalgae biofilm because the study is aimed at a natural system where the ecological significance is dependent on the structure of microbe forming the biofilm, as such, it is paramount to determine the community of microalgae forming the biofilm using relevant microbiological and molecular techniques (Rutherford and Bassler Citation2012).
Nutrient availability
Fresh water microalgae utilized the nutrients such as nitrate and phosphorus present in aquatic environment for natural biofilm formation (Delgadillo-Mirquez Citation2016). Although, it is a well-established fact that during the early growth stage of freshwater microalgae, the cells are metabolically active and tend to utilize more nutrient than the late growth stage when the cell is less active metabolically (Moreno Osorio et al. Citation2019).
This principle tends to be insignificant for natural freshwater biofilm formation because at the early growth stage, the biofilm is not yet settled based on its low density and absence of EPS, thereby using up lesser amount of nutrients compared to that at the late growth stage when the microalgae is fully matured, which has higher density and settle comfortably on the organic substrate (Zhu et al. Citation2016). The formation of EPS also contributes to the high usability of nutrient because the EPS aids the attachment of other non-microalgae organism which also need nutrient to survive (Pierre et al. Citation2019). Studies have shown that elevated nutrients concentration in aquatic environment tends to promote EPS production while nutrient starvation leads to the degradation of EPS resulting to the detachment of the contributing organism in the biofilm formation (Kesaano and Sims Citation2014).
The large amount of nutrient used up by the freshwater microalgae biofilm at the late stage of formation is the reason why the biomass of freshwater microalgae cannot be used for lipid production. Furthermore, it buttresses the reason why any aquatic bodies consisting of freshwater microalgae biofilm do not permit algae blooms (Zhu et al. Citation2016).
Availability and duration of light
Natural freshwater biofilm depends on light for formation, because freshwater microalgae are autotrophic organisms that rely on light for the production of food via photosynthesis and also EPS production (Pierre et al. Citation2019). The availability and duration of light tend to play a significant role in the formation of freshwater microalgae biofilm in natural environment (Shen Citation2014). This presence of light led to the increase in nutrient utilization, thus enhancing the production of EPS which in turn attracts more organisms into the biofilm (Moreno Osorio et al. Citation2019). Whereas the increase in duration of light could be detrimental to the freshwater microalgae formation because the freshwater microalgae could undergo oxidative stress (Diaz and Plummer Citation2018), consequently, affecting the formation of the biofilm. Moreover, this can be attributed to the reason why some freshwater environment never form natural freshwater microalgae but rather algae blooms (López et al. Citation2010).
Natural freshwater microalgae biofilm extracellular matrix (EPS)
The EPS matrix of freshwater microalgae is a three-dimensional network consisting of exopolysaccharides, proteins and nucleic acids that gives biochemical and structural supports to the cells (that make up the freshwater biofilm) (López et al. Citation2010). The role of the EPS in the biofilm association is to encase the cells and also aid in the communications, biochemical signals and gene exchange (Costa et al. Citation2018). The EPS also plays a role in the external digestion of pollutants because the matrix traps extracellular enzymes and keep them in close proximity to the participant cells (Decho and Gutierrez Citation2017). Freshwater biofilms contain water channels that help in the distribution of nutrients and signalling molecules (Decho and Gutierrez Citation2017).
Freshwater microalgae in a biofilm association are likely to possess different characteristics to free-floating freshwater microalgae by reason of the interaction occurring between the freshwater microalgae and organisms within the dense and protected environment of the film. The reason why freshwater microalgae are highly resistant to detergents and antibiotics is because the dense extracellular matrix and the outer layer of cells protect the interior of the community (Stoodley et al. Citation2002).
The role of natural freshwater microalgae biofilm in pollutant degradation from mining wastewater
Mining activities is associated with the production of wastewater which is detrimental to the flora and fauna of freshwater environment. The utilization of freshwater microalgae biofilm in the treatment of mining wastewater will help to minimize the menace associated with the production of this wastewater due to the natural principle behind the production of freshwater microalgae biofilm, making it easily accessible and cost effective (Escapa et al. Citation2017).
The mechanisms involved in pollutant degradation by microalgae biofilm are a natural process involving biosorption and interaction. Since thousands of microalgae cell are involved in microalgae biofilm formation, these biofilm can biodegrade or biotransform pollutants resulting from mining activities by either degradation or accumulation (Wang et al. Citation2019). The ability of microalgae biofilm to cause the degradation of pollutants present in mining wastewater could be as a result of the enzyme activities produced by the individual microalgae cell present in the biofilm (Baghour Citation2019) or due to the increased level of reactive oxygen species (ROS) produced as a result of the high amount of microalgae cells forming the biofilm (Ugya et al. Citation2020).
Many studies have shown that freshwater microalgae biofilm is able to produce reactive oxygen species (ROS) which is capable of oxidizing persistent organic compound, thereby bringing about the degradation of these compounds into CO2, CO32- and H2O with the products being utilized by the freshwater microalgae for biomass production (Shi and Xu Citation2019). Studies by Ugya et al. (Citation2020) show that freshwater microalgae biofilm is able to produce ROS of high concentration, which is capable of degrading persistent organic compounds such as trichlorobenzene, tetrachlorobenzene, alkyl halides, and some heavy organic compounds such as PCBs. These studies also reveal that the ROS produced by freshwater microalgae is capable of degrading sulphur containing pollutants because of the potentiality of ROS to desulfurize thiocarbonyl compounds such as thiouracils, thioamides and thioureas to carbonyl compounds (Shi and Xu Citation2019).
Freshwater microalgae have cellular organization similar to bacteria but also different in their ability to perform photosynthesis. The ability of freshwater microalgae to perform photosynthesis is very important to life on water and land because during the photosynthetic process, they are most likely to exhaust the greenhouse gases particularly CO2 produced during mining activities leading to the production and release of more oxygen (Benedetti et al. Citation2018).
Microalgae biofilm is able to remove inorganic substances such as phosphorus, ammonia and nitrogen compounds present in freshwater habitat by utilizing these substances for biomass production, thereby preventing the substance from accumulating to a toxic level in freshwater habitat (Naidoo Citation2019).
Freshwater microalgae are also able to oxidize or reduce toxic metals in freshwater environment, thus preventing the toxicity to freshwater biota (Cakmak et al. Citation1987). This is also possible via the production and release of ROS by freshwater microalgae which oxidized these toxic metals to usable elements by the freshwater microalgae. Studies by Cakmak et al. (Citation1987) in marine environment show that the ROS produced by marine microalgae is capable of increasing the amount of iron present in the environment via the reduction of ferric compounds (Cakmak et al. Citation1987).
Conclusion and future direction
Mining activities negatively alter both aquatic and terrestrial ecosystems in enormous ways. The water generated tend to affect the biota of the receiving surface water and also pose a critical threat to the public health of terrestrial organism. The incessant artisanal mining activities in developing countries are worrisome due to the menace associated with it. Most artisanal mining is carried out without environmental impact assessment (EIA), and as a result, the fate of the environmental pollutants produced during mining activities is not considered. These unofficial mining activities are the rationale behind lead poisoning in three local government areas of Zamfara State in Nigeria, which was recorded in March 2010 (Udiba et al. Citation2019).
Microalgae biofilm can effectively be used as a tool in the remediation of water pollution resulting from mining activities. This is because microalgae biofilm can occur naturally in aquatic environment, so its usage under field conditions will never be a difficult task. The enhancement of environmental factors such as nutrients and light will help freshwater microalgae biofilm formation. Microalgae biofilm tends to prevent the occurrences of algae blooms which is the severe threat to freshwater environment. The stimulations of environmental factors can also make freshwater microalgae biofilm to release higher amount of reactive oxygen species (ROS) that can regulate the growth of bacteria, thereby minimizing the loss of dissolved oxygen (Udiba et al. Citation2019).
Although, microalgae biofilm removes pollutants from mining wastewater by degradation and biosorption. More research needs are required to clarify if the enzymes released by microalgae biofilm during degradation process are the sole obligation of the entire cells partaking in the biofilm or that of the extracellular matrix. There is also a need to explore the role of EPS in the biosorption process of heavy metal removal from mining wastewater and explain its removal mechanism. It is also important to comprehensively understand the kinetic model that best explains the chemical and physical reactions of heavy metal absorption by the surface of microalgae biofilm.
Acknowledgment
The first author appreciates the support rendered by the PTDF 2018 PhD scholars of Jilin University Changchun, China
Disclosure statement
No potential conflict of interest was reported by the authors.
Additional information
Funding
References
- Abdel-Raouf N, Al-Homaidan AA, Ibraheem IBM. 2012. Microalgae and wastewater treatment. Saudi J Biol Sci. 19(3):257–275.
- Ajibade FO, Adelodun B, Lasisi KH, Fadare OO, Ajibade TF, Nwogwu NA, Sulaymon ID, Ugya AY, Wang HC, Wang A. 2020. Environmental pollution and their socioeconomic impacts. In: Kumar A., Singh V.K., Singh P., Mishra V.K., editor. Microbe mediated remediation of environmental contaminants. Elsevier: Woodhead Publishing. doi:https://doi.org/10.1016/B978-0-12-821199-1.00025-0.
- Alavi, S., Rafieyan, S., Yavari-Bafghi, M., Amoozegar, M. A. 2020. Extremophiles: A powerful choice for bioremediation of toxic oxyanions. In M. P. Shah, editor. Microbial bioremediation & biodegradation (pp. 203–249). Singapore: Springer Singapore.
- Almalki AM, et al. 2019. Effects of mining activities on Gerbillus nanus in Saudi Arabia: A biochemical and histological study. Animals: an Open Access Journal from MDPI. 9(9):664.
- Arunasri K, Mohan SV. 2019. Chapter 2.3 - biofilms: Microbial life on the Electrode surface. In: S.V. Mohan, S. Varjani, A. Pandey, editor. Microbial Electrochemical Technology. Elsevier; p. 295–313.
- Baghour M. 2019. Algal degradation of organic pollutants. In: L.M.T. Martínez, O.V. Kharissova, B.I. Kharisov, editor. Handbook of ecomaterials. Cham: Springer International Publishing; p. 565–586.
- Baykoucheva S. 2015. 5 - Finding and managing scientific information. In: S. Baykoucheva, editor. Managing scientific information and research data. USA: Chandos Publishing; p. 33–41.
- Benedetti M, Vecchi V, Barera S, Dall’Osto L. 2018. Biomass from microalgae: the potential of domestication towards sustainable biofactories. Microb Cell Fact. 17(1):173.
- Besemer K. 2015. Biodiversity, community structure and function of biofilms in stream ecosystems. Res Microbiol. 166(10):774–781.
- Brewster C, et al. 2017. Occurrence, distribution and composition of aliphatic and polycyclic aromatic hydrocarbons in sediment cores from the Lower Fox River, Wisconsin, US. Environ Sci Pollution Res. 25:1–15.
- Cakmak I, van de Wetering DAM, Marschner H, Bienfait HF. 1987. Involvement of superoxide radical in extracellular ferric reduction by iron-deficient bean roots. Plant Physiol. 85(1):310–314.
- Chen Z, et al. 2012. Distribution patterns of polychlorinated dibenzo-p-dioxins and polychlorinated dibenzofurans in sediments of the Xiangjiang River, China. Environ Monit Assess. 184(12):7083–7092. doi: https://doi.org/10.1007/s10661-011-2481-0.
- Cole CA. 1999. Surface mining, strip mining, quarries. In: Environmental Geology. Alexander, D.E., Fairbridge, Rhodes W, Dordrecht: Springer Netherlands, p. 586-587.
- Considine R, Tynan R, James C, Wiggers J, Lewin T, Inder K, Perkins D, Handley T, Kelly B, Behrens T. 2017. The contribution of individual, social and work characteristics to employee mental health in a coal mining industry population. PLOS ONE. 12(1):e0168445.
- Costa OYA, Raaijmakers JM, Kuramae EE. 2018. Microbial extracellular polymeric substances: ecological function and impact on soil aggregation. Front Microbiol. 9:1636–1636.
- Cui X, Dong J, Huang Z, Liu C, Qiao X, Wang X, Zhao X, Zheng B, Shen J. 2020. Polychlorinated biphenyls in the drinking water source of the Yangtze River: characteristics and risk assessment. Environ Sci Europe. 32(1):29.
- Ćwieląg-Drabek M, Piekut A, Gut K, Grabowski M. 2020. Risk of cadmium, lead and zinc exposure from consumption of vegetables produced in areas with mining and smelting past. Sci Rep. 10(1):3363.
- Davey ME, O'Toole GA. 2000. Microbial biofilms: from ecology to molecular genetics. Microbiol Mol Biol Rev MMBR. 64(4):847–867.
- Davidson AD, Shoemaker KT, Weinstein B, Costa GC, Brooks TM, Ceballos G, Radeloff VC, Rondinini C, Graham CH, Kamilar JM. 2017. Geography of current and future global mammal extinction risk. PLOS ONE. 12(11):e0186934.
- Day-Lewis FD, Slater LD, Robinson J, Johnson CD, Terry N, Werkema D. 2017. An overview of geophysical technologies appropriate for characterization and monitoring at fractured-rock sites. J Environ Manag. 204(Pt 2):709–720.
- Decho AW, Gutierrez T. 2017. Microbial extracellular polymeric substances (EPSs) in ocean systems. Front Microbiol. 8(922.
- Delgadillo-Mirquez L, et al. 2016. Nitrogen and phosphate removal from wastewater with a mixed microalgae and bacteria culture. Biotechnology Reports (Amsterdam, Netherlands). 11:18–26.
- Diaz JM, Plummer S. 2018. Production of extracellular reactive oxygen species by phytoplankton: past and future directions. J Plankton Res. 40(6):655–666.
- Donlan RM. 2002. Biofilms: microbial life on surfaces. Emerg Infect Dis. 8(9):881–890.
- Duncan AE, de Vries N, Nyarko KB. 2018. Assessment of heavy metal pollution in the sediments of the River Pra and its tributaries. Water Air Soil Pollut. 229(8):272.
- Eludoyin AO, Ojo AT, Ojo TO, Awotoye OO, Nzeadibe T. 2017. Effects of artisanal gold mining activities on soil properties in a part of southwestern Nigeria. Cogent Environ Sci. 3(1):1305650.
- Emmanuel AY, Jerry CS, Dzigbodi DA. 2018. Review of environmental and health impacts of mining in Ghana. J Health Pollution. 8(17):43–52.
- Escapa C, Coimbra RN, Paniagua S, García AI, Otero M. 2017. Comparison of the culture and harvesting of Chlorella vulgaris and Tetradesmus obliquus for the removal of pharmaceuticals from water. J Appl Phycol. 29(3):1179–1193.
- Ezeokoli OT, Bezuidenhout CC, Maboeta MS, Khasa DP, Adeleke RA. 2020. Structural and functional differentiation of bacterial communities in post-coal mining reclamation soils of South Africa: bioindicators of soil ecosystem restoration. Sci Rep. 10(1):1759.
- Fernandes AN, Gouveia CD, Grassi MT, da Silva Crespo J, Giovanela M. 2014. Determination of monoaromatic hydrocarbons (BTEX) in surface waters from a Brazilian Subtropical Hydrographic Basin. Bull Environ Contam Toxicol. 92(4):455–459.
- Ghani AC, Sutherland CJ, Riley EM, Drakeley CJ, Griffin JT, Gosling RD, Filipe JAN, Riley S. 2009. Loss of population levels of immunity to malaria as a result of exposure-reducing interventions: consequences for interpretation of disease trends. PLOS ONE. 4(2):e4383.
- Griffith SV. 1960. CHAPTER II - PROSPECTING METHODS. In: S.V. Griffith, editor. Alluvial prospecting and mining (second edition). Pergamon; p. 7–32.
- Groves DI, Foster RP. 1991. Archaean lode gold deposits. In: R.P. Foster, editor. Gold metallogeny and exploration. Boston, MA: Springer US; p. 63–103.
- Guo Z, Sun Y, Pan S-Y, Chiang P-C. 2019. Integration of green energy and advanced energy-efficient technologies for municipal wastewater treatment plants. Int J Environ Res Public Health. 16(7):1282.
- Haddaway NR, Cooke SJ, Lesser P, Macura B, Nilsson AE, Taylor JJ, Raito K. 2019. Evidence of the impacts of metal mining and the effectiveness of mining mitigation measures on social–ecological systems in Arctic and boreal regions: a systematic map protocol. Environmental Evidence. 8(1):9.
- Haldar SK. 2013. Chapter 2 - economic mineral deposits and Host rocks. In: S.K. Haldar, editor. Mineral exploration. Boston: Elsevier; p. 23–39.
- Han X, Wang Z, Chen M, Zhang X, Tang CY, Wu Z. 2017. Acute responses of microorganisms from membrane bioreactors in the presence of NaOCl: Protective mechanisms of extracellular polymeric substances. Environ Sci Technol. 51(6):3233–3241.
- Harfoot MBJ, Tittensor DP, Knight S, Arnell AP, Blyth S, Brooks S, Butchart SHM, Hutton J, Jones MI, Kapos V, et al. 2018. Present and future biodiversity risks from fossil fuel exploitation. Conserv Lett. 11(4):e12448.
- Heisler J, Glibert PM, Burkholder JM, Anderson DM, Cochlan W, Dennison WC, Dortch Q, Gobler CJ, Heil CA, Humphries E, et al. 2008. Eutrophication and harmful algal blooms: A scientific consensus. Harmful Algae. 8(1):3–13.
- Hendryx M, Yonts SD, Li Y, Luo J. 2019. Mountaintop removal mining and multiple illness symptoms: A latent class analysis. Sci Total Environ. 657:764–769.
- Huang X, Sillanpää M, Gjessing ET, Peräniemi S, Vogt RD. 2010. Environmental impact of mining activities on the surface water quality in Tibet: Gyama valley. Sci Total Environ. 408(19):4177–4184.
- Jaffé R. 1991. Fate of hydrophobic organic pollutants in the aquatic environment: A review. Environ Pollut. 69(2):237–257.
- Jain MK, Das A. 2017. Impact of mine waste Leachates on aquatic environment: A review. Current Pollution Reports. 3(1):31–37.
- Kannan K, Yun SH, Ostaszewski A, McCabe JM, Mackenzie-Taylor D, Taylor AB. 2008. Dioxin-like toxicity in the Saginaw River Watershed: polychlorinated dibenzo-p-dioxins, dibenzofurans, and biphenyls in sediments and floodplain soils from the Saginaw and Shiawassee Rivers and Saginaw Bay, Michigan, USA. Arch Environ Contam Toxicol. 54:9–19.
- Kesaano M, Sims R. 2014. Algal biofilm based technology for wastewater treatment. Algal Res. 5. 231–240.
- Li D, Chan K-S, Schilling KE. 2013. Nitrate concentration trends in Iowa's Rivers, 1998 to 2012: What challenges await nutrient reduction initiatives? J Environ Qual. 42(6):1822–1828.
- Liu J, Lu G, Zhang F, Nkoom M, Yan Z, Wu D. 2018. Polybrominated diphenyl ethers (PBDEs) in a large, highly polluted freshwater lake, China: Occurrence, fate, and risk assessment. Int J Environ Res Public Health. 15:1529.
- López D, Vlamakis H, Kolter R. 2010. Biofilms. Cold Spring Harbor Perspect Biol. 2(7):a000398–a000398.
- López Y, Soto SM. 2019. The usefulness of microalgae compounds for preventing biofilm infections. Antibiotics (Basel, Switzerland). 9(1):9.
- Maier RM, Díaz-Barriga F, Field JA, Hopkins J, Klein B, Poulton MM. 2014. Socially responsible mining: the relationship between mining and poverty, human health and the environment. Rev Environ Health. 29(1-2):83–89.
- Mantzorou A, Ververidis F. 2019. Microalgal biofilms: A further step over current microalgal cultivation techniques. Sci Total Environ. 651:3187–3201.
- Mhlongo SP, Mativenga PT, Marnewick A. 2018. Water quality in a mining and water-stressed region. J Cleaner Prod. 171:446–456.
- Miranda AF, Ramkumar N, Andriotis C, Höltkemeier T, Yasmin A, Rochfort S, Wlodkowic D, Morrison P, Roddick F, Spangenberg G, et al. 2017. Applications of microalgal biofilms for wastewater treatment and bioenergy production. Biotechnol Biofuels. 10:120–120.
- Mohamed ZA. 2018. Potentially harmful microalgae and algal blooms in the Red Sea: Current knowledge and research needs. Mar Environ Res. 140:234–242.
- Moreno Osorio JH, Pinto G, Pollio A, Frunzo L, Lens PNL, Esposito G. 2019. Start-up of a nutrient removal system using Scenedesmus vacuolatus and Chlorella vulgaris biofilms. Bioresour Bioprocess. 6(1):27.
- Muhammad MH, et al. 2020. Beyond risk: Bacterial biofilms and their regulating approaches. Front Microbiol. 11(928).
- Naidoo RK, et al. 2019. Nutrient exchange of carbon and nitrogen promotes the formation of stable mutualisms between Chlorella sorokiniana and Saccharomyces cerevisiae under engineered synthetic growth conditions. Front Microbiol. 10(609).
- Nanny MA, Ratasuk N. 2002. Characterization and comparison of hydrophobic neutral and hydrophobic acid dissolved organic carbon isolated from three municipal landfill leachates. Water Res. 36(6):1572–1584.
- Navarro A, Collado D, Carbonell M, Sanchez JA. 2004. Impact of mining activities on soils in a semi-arid environment: Sierra Almagrera district, SE Spain. Environ Geochem Health. 26(3):383–393.
- Ngole-Jeme VM, Fantke P. 2017. Ecological and human health risks associated with abandoned gold mine tailings contaminated soil. PLOS ONE. 12(2):e0172517.
- Obiadi II, Obiadi CM, Akudinobi BEB, Maduewesi UV, Ezim EO. 2016. Effects of coal mining on the water resources in the communities hosting the Iva Valley and Okpara Coal Mines in Enugu State, Southeast Nigeria. Sustain Water Res Manage. 2(3):207–216.
- Paerl HW. 2018. Mitigating toxic planktonic cyanobacterial blooms in aquatic ecosystems facing increasing anthropogenic and climatic pressures. Toxins (Basel). 10(2):76.
- Pierre G, Delattre C, Dubessay P, Jubeau S, Vialleix C, Cadoret J-P, Probert I, Michaud P. 2019. What is in store for EPS microalgae in the next decade? Molecules. 24(23):4296.
- Pikoli MR, Sari AF, Solihat NA, Permana AH. 2019. Characteristics of tropical freshwater microalgae Micractinium conductrix, Monoraphidium sp. and Choricystis parasitica, and their potency as biodiesel feedstock. Heliyon. 5(12):e02922–e02922.
- Prach K, Tolvanen A. 2016. How can we restore biodiversity and ecosystem services in mining and industrial sites? Environ Sci Pollution Res. 23(14):13587–13590.
- Puyol D, Batstone DJ, Hülsen T, Astals S, Peces M, Krömer JO. 2017. Resource recovery from wastewater by biological technologies: Opportunities, challenges, and prospects. Front Microbiol. 7:2106–2106.
- Qin WC, Su LM, Zhang XJ, Qin HW, Wen Y, Guo Z, Sun FT, Sheng LX, Zhao YH, Abraham MH. 2010. Toxicity of organic pollutants to seven aquatic organisms: effect of polarity and ionization. SAR QSAR Environ Res. 21(5-6):389–401.
- Roostaei J, Zhang Y, Gopalakrishnan K, Ochocki AJ. 2018. Mixotrophic microalgae biofilm: A novel algae cultivation strategy for improved productivity and cost-efficiency of biofuel feedstock production. Sci Rep. 8(1):12528.
- Rutherford ST, Bassler BL. 2012. Bacterial quorum sensing: its role in virulence and possibilities for its control. Cold Spring Harbor Perspect Med. 2(11):a012427.
- Schmale DG, Ault AP, Saad W, Scott DT, Westrick JA. 2019. Perspectives on harmful algal blooms (HABs) and the cyberbiosecurity of freshwater systems. Front Bioeng Biotechnol. 7(128).
- Schweitzer L, Noblet J. 2018. Chapter 3.6 - water contamination and pollution. In: B. Török, T. Dransfield, editor. Green Chemistry. Elsevier; p. 261–290.
- Sharma S, Bhattacharya A. 2017. Drinking water contamination and treatment techniques. Appl Water Sci. 7(3):1043–1067.
- Shen Y, et al. 2014. An auto-flocculation strategy for Chlorella vulgaris. Biotechnol Lett. 37(1):75-80.
- Shi S, Xu G. 2019. Identification of phosphorus fractions of biofilm sludge and phosphorus release, transformation and modeling in biofilm sludge treatment related to pH. Chem Eng J. 369:694–704.
- Singer DA, Kouda R. 1999. Examining risk in mineral exploration. Nat Resour Res. 8(2):111–122.
- Sonter LJ, Ali SH, Watson JEM. 2018. Mining and biodiversity: key issues and research needs in conservation science. Proceedings. Biological Sciences. 285(1892):20181926.
- Sonthiphand P, Cejudo E, Schiff SL, Neufeld JD. 2013. Wastewater Effluent impacts ammonia-oxidizing Prokaryotes of the Grand River, Canada. Appl Environ Microbiol. 79(23):7454.
- Stauffer BA, Bowers HA, Buckley E, Davis TW, Johengen TH, Kudela R, McManus MA, Purcell H, Smith GJ, Vander Woude A, Tamburri MN. 2019. Considerations in harmful algal bloom research and Monitoring: Perspectives from a Consensus-Building Workshop and Technology Testing. Frontiers in Marine Science. 6:399.
- Stewart AG. 2020. Mining is bad for health: a voyage of discovery. Environ Geochem Health. 42(4):1153–1165.
- Stoodley P, Sauer K, Davies DG, Costerton JW. 2002. Biofilms as complex differentiated communities. Annu Rev Microbiol. 56:187–209.
- Tarras-Wahlberg NH, Flachier A, Lane SN, Sangfors O. 2001. Environmental impacts and metal exposure of aquatic ecosystems in rivers contaminated by small scale gold mining: the Puyango River basin, southern Ecuador. Sci Total Environ. 278(1):239–261.
- Taylor-Brown A, Booth R, Gillett A, Mealy E, Ogbourne SM, Polkinghorne A, Conroy GC, Bhadauria T. 2019. The impact of human activities on Australian wildlife. PLOS ONE. 14(1):e0206958.
- Tchounwou PB, Yedjou CG, Patlolla AK, Sutton DJ. 2012. Heavy metal toxicity and the environment. Experientia Supplementum. 101:133–164.
- Udiba UU, Akpan ER, Antai EE. 2019. Soil lead concentrations in Dareta Village, Zamfara, Nigeria. Journal of Health & Pollution. 9(23):190910–190910.
- Ugya AY, Ajibade FO, Ajibade TF. 2018. Water pollution resulting from mining activity: An Overview. Proceedings of the 2018 Annual Conference of the School of Engineering & Engineering Technology (SEET), The Federal University of Technology, Akure, Nigeria.
- Ugya AY, Imam TS, Li A, Ma J, Hua X. 2020. Antioxidant response mechanism of freshwater microalgae species to reactive oxygen species production: a mini review. Chemistry and Ecology. 36(2):174–193.
- Ugya AY, Imam TS, Ma J. 2019. Mini-review on the efficiency of Aqutic Macrophytes as Mosquito Larvicide. Journal of Applied Botany and Food Quality. 92:320–326.
- Virgone KM, Ramirez-Andreotta M, Mainhagu J, Brusseau ML. 2018. Effective integrated frameworks for assessing mining sustainability. Environ Geochem Health. 40(6):2635–2655.
- Wang L, Liang T. 2015. Distribution characteristics of phosphorus in the Sediments and overlying water of Poyang lake. PLOS ONE. 10(5):e0125859.
- Wang L, Wang X, Jin X, Xu J, Zhang H, Yu J, Sun Q, Gao C, Wang L. 2017. Analysis of algae growth mechanism and water bloom prediction under the effect of multi-affecting factor. Saudi J Biol Sci. 24(3):556–562.
- Wang L, Xiao H, He N, Sun D, Duan S. 2019. Biosorption and Biodegradation of the environmental Hormone Nonylphenol By Four marine microalgae. Sci Rep. 9(1):5277–5277.
- Wells ML, Trainer VL, Smayda TJ, Karlson BSO, Trick CG, Kudela RM, Ishikawa A, Bernard S, Wulff A, Anderson DM, Cochlan WP. 2015. Harmful algal blooms and climate change: Learning from the past and present to forecast the future. Harmful Algae. 49:68–93.
- Wuana RA, Okieimen FE. 2011. Heavy metals in contaminated Soils: A review of sources, Chemistry, Risks and best available Strategies for remediation. ISRN Ecology. 2011:402647.
- Xie F, Zhang TA, Dreisinger D, Doyle F. 2014. A critical review on solvent extraction of rare earths from aqueous solutions. Miner Eng. 56:10–28.
- Xuemei W, Jingling L, Muyuan M, Zhifeng Y. 2010. Response of freshwater biofilm to pollution and ecosystem in Baiyangdian lake of China. Proc Environ Sci. 2:1759–1769.
- Yu R, He L, Cai R, Li B, Li Z, Yang K. 2017. Heavy metal pollution and health risk in China. Global Health Journal. 1(1):47–55.
- Zendehboudi S, Bahadori A. 2017. Chapter Eight - production methods in shale Oil Reservoirs. In: S. Zendehboudi, A. Bahadori, editor. Shale Oil and Gas Handbook. Gulf Professional Publishing; p. 285–319. Texas, USA.
- Zhu LD, Li ZH, Hiltunen E. 2016. Strategies for lipid production Improvement in microalgae as a Biodiesel Feedstock. BioMed Res Int. 2016:8792548–8792548.
- Zullaikah S, et al. 2019. 9 - Ecofuel conversion technology of inedible lipid feedstocks to renewable fuel. In: K. Azad, editor. Advances in Eco-Fuels for a Sustainable environment. Woodhead Publishing; p. 237–276.