Abstract
In the current study, a model of ‘fungus-root’ interaction was designed using host Zea mays inoculated with a metal-tolerant dark septate endophyte (DSE) strain of Exophiala pisciphila H93 both under nutritional- (Zn0) and zinc-stressed (Zn550) conditions. We found that the co-cultured maize benefited from the alleviated excessive Zn toxicity by its root-associated E. pisciphila, through significantly decreasing root Zn under Zn550 conditions, or promoting the nutritional Zn in maize shoots and roots by 34.03% and 22.13% under Zn0 conditions, respectively, compared with non-inoculated controls. Interestingly, H93 further enhanced the maize Zn tolerance by the bioaugmented Zn compartmentation in root cell walls to reduce the proportion of Zn in maize root soluble fractions and cell organelles under Zn550 stress. In exchange for the maize carbon cost, the H93 extraradical hyphae tended to accumulate more toxic Zn, compared with H93 mycelia in the in vitro culture under Zn550 conditions, and vice versa the co-cultured maize also promoted Zn tolerance of H93 via the repartitioning of subcellular Zn. Our results suggest the potentially mutual association of E. pisciphila and its host maize, by trading carbon for the increased abiotic tolerance.
GRAPHICAL ABSTRACT
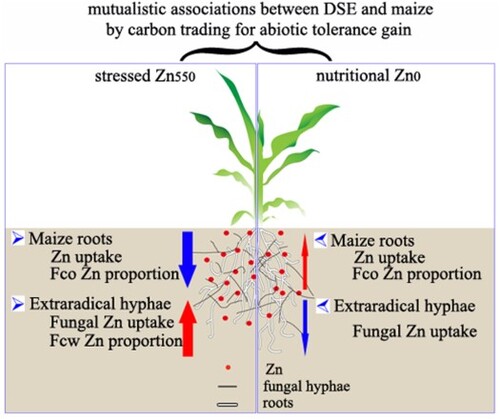
Introduction
Zinc (Zn) is a vital trace element in living things. Zinc acts as a functional, structural, or regulatory cofactor in a large number of enzymes. Zn deficiency is a global concern for human health and causes a decrease in crop production and nutritional characteristics (Zulfiqar et al. Citation2020; Rehman et al. Citation2021). Zinc deficiency also increases membrane leakage by inhibiting the activity of enzymes involved in the detoxification of membrane-damaging oxygen radicles (Brown et al. Citation1993). But Zn may also become toxic when present in excess, and excessive Zn is reported to inhibit plant growth, alter the nutrient balance, induce chlorosis, and affect the germination process (Rasafi et al. Citation2016; Barberis et al. Citation2020; Galati et al. Citation2021).
Dark septate endophytes (DSEs) are a group of multifunctional root endophytic fungi that form an association with numerous plant roots (Yung et al. Citation2021). The distinct morphological characters of DSE in plant roots are the melanized septate intercellular and intracellular running hyphae, and various microsclerotia that are composed of compact masses of thick-walled and pigmented cells (Jumpponen and Trappe Citation1998; Jumpponen Citation2001; Shadmani et al. Citation2021a). The taxonomic status of DSEs is ambiguous, and the interaction with their host plants is not well elucidated. Previous studies have shown that DSEs are one of the most ubiquitous root-associated fungi, and are especially common in various stressful environments, such as heavy metal (HM)-contaminated soils (Zhang et al. Citation2008; Gucwa-Przepióra et al. Citation2016; Ban et al. Citation2017; Shadmani et al. Citation2021a, Citation2021b).
Some typical DSE strains isolated from HM-contaminated soils, such as Exophiala pisciphila (Li et al. Citation2011; Zhang et al. Citation2020) and Phialophora/Cadophora complexes (Likar and Regvar Citation2013), have been demonstrated to have a strong tolerance to HM in vitro culture conditions. Increasing reports reveal that various well-known HM-tolerant pathways are responsible for conferring the extreme HM tolerance of the involved diverse fungi. For example, the HM-tolerant pathways of fungi are the altered chemical forms of the metals intracellularly chelated by producing metallothioneins and glutathione (Khullar and Reddy Citation2018). And the Cd tolerant mechanisms of DSE are the enhanced subcellular accumulation in cell walls and integrated with phosphate as the increased Cd in the media (Zhan et al. Citation2015a). Based on transcriptome analysis, the HM tolerance of a DSE involves at least 10 metabolic pathways (Zhao et al. Citation2015a). More importantly, increasing evidence shows that DSE colonization enhances the HM tolerance of their host plants, as DSEs can modify the physiological metabolism of plants, including changes in antioxidant enzyme activities, antioxidant contents, metabolic pathways of plant hormones, and others (Affholder et al. Citation2014; Hui et al. Citation2015; Mani et al. Citation2015; Zhan et al. Citation2015b; Zhu et al. Citation2018; Ban et al. Citation2021). Furthermore, DSEs could regulate the expression of plant genes involved in metal absorption, migration, and accumulation in plants, which would further affect the metal tolerance of plants (Wang et al. Citation2016). Although the mechanisms of metal tolerance of DSEs in vitro and their impacts on the metal tolerance of their host plants under co-culture conditions have been documented (Zhan et al. 2015; Wang et al. Citation2016), little is known about the impacts of the host plants on DSEs under co-culture conditions; for example, the impact of maize on the absorption and accumulation of metals in DSE mycelium under metal-stressed conditions.
E. pisciphila H93 is characterized as one of the dominant DSEs in an old mine smelting site in Yunnan, southwest China, and shows a high tolerance to HMs (Pb, Zn, and Cd) in vitro (Zhang et al. Citation2008). In the greenhouse, H93 alleviated the phytotoxicity of Pb, Zn, and Cd stress and promoted maize growth by restricting the translocation of metals from roots to shoots (Li et al. Citation2011; Wang et al. Citation2016). In the present study, we investigated the effects of H93 colonization on the absorption and subcellular distribution of Zn in maize roots and shoots under nutritional and stressed Zn conditions. We also compared the differences in Zn accumulation and subcellular distribution in the H93 mycelium under in vitro and co-culture conditions. Our results illustrate the interactions between DSE and host maize under Zn nutritional and stressed conditions. The research results are helpful for understanding the relationships of DSEs to their host plants and provide new insights into how DSEs and host plants interact.
Materials and methods
Biological materials and experimental design
E. pisciphila H93 (Agricultural Culture Collection Center of China, accession No.: ACCC32496), one of the dominant DSEs in an old mine smelting site in Yunnan, southwest China, was selected as the DSE inoculant (Zhang et al. Citation2008). Huidan No. 4, one of the main local maize cultivars in Yunnan, China, was selected as the host plant. In an artificial climate chamber, a ‘fungus-root’ interaction model of maize inoculated with E. pisciphila H93 was set up in one-half-strength (1/2 ×) Hoagland’s solution (Abid et al. Citation2019; Szopinski et al. Citation2019). As a negative control of the extraradical mycelia in co-cultured conditions, H93 mycelia were also cultured in vitro in the above nutritional culture broth supplemented with VB1 100 mg/L and 15 g/L glucose. In the present study, two Zn levels were set up via the additional supplementation with 0 (Zn0) or 550 mg/L Zn2+ (Zn550) (ZnSO4·7H2O) (Diao et al. Citation2013). Thus, two experiments were performed in a completely randomized block design with a 2 × 2 factorial scheme with the following variables: four treatments for maize in Experiment 1, i.e. two inoculation treatments (inoculation with H93 or not), with two levels of Zn addition (Zn0 and Zn550); and four treatments of H93 mycelia in Experiment 2, that is, two cultivation conditions (i.e. in vitro and co-cultured with maize) under the above two levels of Zn addition. Four replicates for each treatment were carried out.
Experiment 1: DSE-maize co-culture
Maize seeds were surface-sterilized using 75% ethanol for 5 min and 10% sodium hypochlorite for 10 min, then washed three times in sterile water and aseptically germinated at 25 °C for three days. Then, the germinated seeds were transplanted into 250 mL Erlenmeyer flasks (one seed per flask) containing 10 mL sterile water and cultured at 25/22 °C (day/night) and 70% humidity with a 16/8 h photoperiod (18,000 lux). Three days later, each seedling was inoculated with 0.5 g 20-day-old H93 mycelia, and the negative controls received the same amount of the inactivated H93 mycelia (autoclaved at 121 °C for 2 h). Seven days later, both the non-inoculated and DSE-inoculated maize seedlings were randomly divided into two groups, and treated with 200 mL of 1/2× Hoagland's solution (CaNO3·4H2O 472.5 mg/L, KNO3 253 mg/L, NH4NO3 40 mg/L, KH2PO4 68 mg/L, MgSO4 246.5 mg/L, FeSO4·7H2O 0.139 mg/L, Na2-EDTA·2H2O 0.1365 mg/L, KI 0.83 mg/L, H3BO3 6.2 mg/L, MnSO4·4H2O 22.3 mg/L, ZnSO4·7H2O 8.6 mg/L, Na2MoO4·2H2O 0.25 mg/L, CuSO4·5H2O 0.025 mg/L, and CoCl2·6H2O 0.025 mg/L) with the addition of either Zn0 or Zn550 (Hoagland and Arnon Citation1950). During the growth period, the maize seedlings were watered with sterile water every other day and added with one-half-strength Hoagland’s solution without additional Zn supplements every six days to a maximum volume of 200 mL culture medium. Fifteen days later, maize shoots and roots and the extraradical hyphae of H93 were separately collected. The maize samples served as the root and shoot samples of the four maize treatments. The samples of H93 extraradical hyphae were assigned as the fungal hyphal materials in co-cultured conditions in Experiment 2.
Experiment 2: DSE culture in vitro
A 6 mm fungal disc of 20-day-old H93 colony growing on a potato dextrose agar (PDA: potato leachate, 1.0 L; glucose, 20.0 g; agar, 15.0 g, and nature pH) was taken and used to inoculate into a 250 mL Erlenmeyer flask containing 100 mL of the above modified 1/2× Hoagland's solution with either an extra 0 mg/L (Zn0) or 550 mg/L Zn (Zn550) supplementation. The inoculated flasks were cultured for 15 days at 120 rpm 28 °C, and then fungal mycelia in vitro were filtered and collected as described in our previous study (Diao et al. Citation2013). Four treatments of H93 mycelia were collected in total, including two DSE hyphal materials in vitro and the above H93 extraradical hyphae in co-cultured conditions with either Zn0 or Zn550 supplementation. Four replicates were carried out for each treatment.
Sampling and DSE colonization
The extraradical hyphae of H93 and the roots of the co-cultured maize were carefully and separately collected and placed in a pre-cooled 20 mM Na2-EDTA (Ethylenediaminetetraacetic acid disodium salt) solution for 20 min to remove the surface-adhesive metal ions, and then washed twice with deionized water. The samples of maize shoots, roots, and H93 extraradical hyphae were divided into two parts: one part was immediately placed in liquid nitrogen and then stored at −80 °C for the following experiments, and the remaining parts were dried to a constant weight in a 60 °C oven used for the measurement of Zn concentration.
About five segments of fresh maize roots 5 cm in length were cut off and placed into test tubes with 5 mL of a 10% (w/v) potassium hydroxide solution in a water bath at 100 °C for 5–10 min, and then stained with 0.5% acid fuchsin (Berch and Kendrick Citation1982). The fungal colonization intensity was determined using the gridline intersect method with 300 intersects under a compound light microscope (Olympus-BX51) (Mcgonigle et al. Citation1990).
Zn concentrations in DSE mycelia and maize roots and shoots
A subsample of the above-dried samples was collected and then ground using a mini-vegetation disintegrator (FZ102, Tianjin City Test Instrument Co. Ltd., Tianjin, China). Then, 0.5 g of H93 mycelium, maize shoot, or root samples in each treatment were weighed and digested with HNO3/HClO4 (4: 1, v/v) in the 100 mL Erlenmeyer flasks. The Zn concentration was determined using an atomic absorption spectrophotometer (ICE3500, Thermo, Waltham, MA, USA) based on the calibration curve using a series of zinc dilutions (0, 0.1, 0.2, 0.4, 0.8, and 1.2 μg/mL; GSB 04-1761-2004, National Research Center for Certified Reference Materials, China) (Zhu et al. Citation2018).
Subcellular distribution of Zn in DSE mycelia, maize shoots, and roots
The Zn concentrations in the subcellular fractions of H93 mycelia both in co-culture and in vitro conditions, maize shoots, and roots were analyzed according to the previous methods with minor modifications (Teng et al. Citation2018; Zhang et al. Citation2019). Briefly, about 0.5 g of fresh-frozen samples were homogenized in a pre-cooled extraction buffer (50 mM Tris-HCl, 250 mM sucrose, and 1 mM dithioerythritol, pH = 7.5) at a ratio of 1: 30 (w/v) with a chilled mortar and pestle. The above homogenates were then either centrifuged at 45 g for 10 min in the case of fungal materials or at 1000 g for 15 min for maize materials. The collected pellets were assigned as the cell wall fraction (Fcw). The remaining supernatant was subsequently further centrifuged at 31,000 g for 45 min for the H93 mycelia or at 17,500 g for 30 min for maize shoots and roots to yield both the soluble fraction (Fs) in the supernatant or the cell organelle fraction (Fco) in the pellets (Teng et al. Citation2018; Zhang et al. Citation2019). All of the steps were performed at 4 °C and the Zn concentrations in different subcellular fractions were determined as described above.
Statistical analysis
All data are presented as means ± standard errors (SE) for all four replicates. The data were submitted for the analysis of the normality and homogeneity of variances using the Shapiro-Wilkson test and Levene statistics, respectively. Data on the concentration and proportion of Zn in different subcellular fractions in maize or E. pisciphila mycelia were subjected to a two-way analysis of variance (ANOVA). Differences in the Zn proportion and concentration in different subcellular fractions between the maize inoculated with E. pisciphila and the non-inoculated treatments, and also in the DSE mycelia between the co-culture and in vitro culture were compared using an independent samples t-test. Statistical analyses were conducted using SPSS 22.0 (SPSS Inc., Chicago, IL, USA), and the graphs were produced using Origin Pro 9.0.0 (OriginLab Corporation, Northampton, USA).
Results
DSE colonization
No DSE structures were observed in the maize roots of the non-inoculated controls. The roots of H93-inoculated maize both under Zn0 and Zn550 treatments were intensively colonized by typical DSE structures e.g. microsclerotia and melanized septate hyphae, with an average of 28.3 ± 0.01% and 45.8 ± 0.01% colonization intensity after 15 days’ growth, respectively (Figure ). Interestingly, the H93 colonization intensity was significantly higher under Zn550 stress treatment than that under the nutritional Zn0 supply (p < 0.05, t-test).
Zn concentration and subcellular distribution in maize shoots and roots
In all maize treatments, Zn was accumulated mainly in maize roots compared with the shoots, regardless of the inoculation treatments (Figure ). Two-way ANOVA results showed that the Zn concentration in maize roots was significantly affected by both Zn and DSE inoculation, as well as a significant interaction between Zn and DSE inoculation. In addition, the Zn concentration in maize shoots was significantly affected by Zn supplementation (Table S1). Compared with their respective Zn0 controls, there was a significant increase of Zn accumulated in maize shoots and roots under Zn550 supplementation for both the inoculated and non-inoculated treatments (Figure ). In contrast to the increase of Zn accumulation in maize shoots (34.03%) and roots (22.13%) under Zn0 treatments, DSE inoculation significantly reduced the root Zn concentration (40.34%) compared with the non-inoculated treatments under Zn550 supplementation (p < 0.05) (Figure ).
Figure 2. Zn concentration in the shoots and roots of maize inoculated with (+DSE) or without (−DSE) E. pisciphila H93 under Zn0 and Zn550 treatments. Data represent means ± SE (n = 4), (* p < 0.05, ** p < 0.01, *** p < 0.001, t-test).
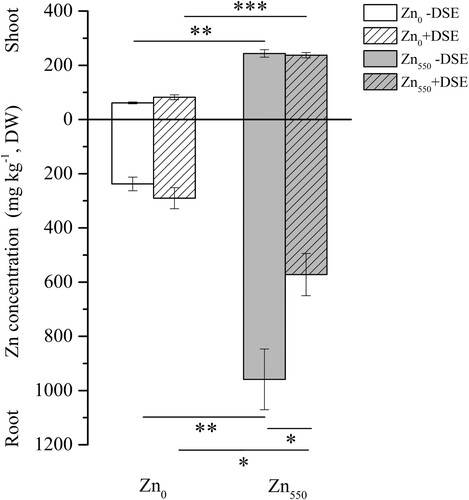
We noted that the majority of Zn (proximately 85.6%–93.9%) was accumulated in the cell walls and soluble fractions of maize shoots and roots, while only a small proportion of Zn was deposited in the cell organelles, regardless of DSE inoculation (Table ). Two-way ANOVA results showed that the Zn subcellular distribution in all three subcellular fractions in maize shoots and roots was significantly affected by the Zn supplementation. The Zn accumulated in both shoot Fcw and root Fs was significantly affected by DSE inoculation, as well as by a significant interaction between Zn and DSE inoculation. There was a significant effect of two-factor interaction on the proportion of Zn in the root Fcw and Fco subfractions, and also a significant effect of Zn and DSE-inoculation on the Zn proportions of Fco in maize shoots and roots (Table S2). Compared with their respective Zn0 controls, the Zn concentration increased significantly in each subcellular fraction of maize shoots and roots for both the DSE- and non-inoculated treatments under Zn550 supplementation (p < 0.05) (Table S3). Additionally, compared with non-inoculated treatments, DSE inoculation significantly increased Zn proportions in the root Fco (121.54%) under Zn0 treatments and in the shoot Fs (9.83%) under Zn550 treatments (Table ). Interestingly, DSE inoculation promoted the nutritional Zn accumulation in the cell organelles of maize shoots (58.06%) and roots (48.28%) (Table S3), with a significantly increased Zn proportion in root Fco (121.54%), compared with the non-inoculated controls under Zn0 treatments. Conversely, under excessive Zn550 stress, DSE inoculation significantly altered the Zn subcellular distribution of maize, including the markedly increased proportion in shoot Fs (9.83%) and the reduced proportion in shoot Fcw (9.80%) (Table ).
Table 1. The proportion of Zn in different subcellular fractions of maize shoots and roots with (+DSE) or without (−DSE) H93 inoculation, under Zn0 and Zn550 supplementation after 15 days’ culture.
Zn concentration, subcellular distribution in H93 mycelia
Two-way ANOVA analysis showed significant effects due to the DSE culture methods and Zn supplementation as well as the two-factor interaction on the Zn concentration accumulated in fungal mycelia (Table S4). Compared with their respective controls under nutritional Zn0 supply, the Zn concentrations in the H93 mycelia both in vitro and in co-culture were obviously increased under Zn550 conditions. It was found that there was a marked increase of Zn concentration in the H93 mycelia (40.87%) when it was co-cultured with maize compared with in vitro culture under Zn550 supplementation (Figure ).
Figure 3. Zn concentration in H93 mycelia both in vitro and co-cultured with maize under Zn0 and Zn550 conditions after 15-days’ growth. Data represent means ± SE, (n = 4) (* p < 0.05, ** p < 0.01, t-test).
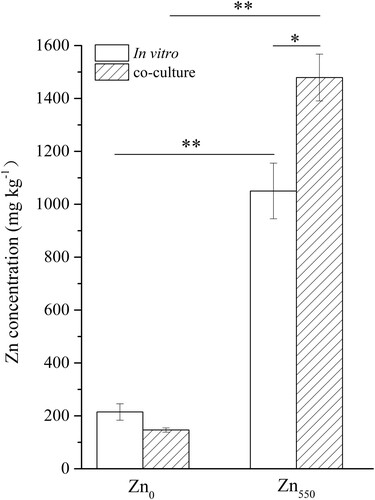
A large percentage of Zn (approximately 85.3%–92.9%) accumulated in the fungal cell walls and soluble fractions, while only a small portion of Zn accumulated in the cell organelles both in vitro and in co-culture (Table ). Two-way ANOVA results showed that the Zn concentration and proportion in all three subcellular fractions of E. pisciphila mycelia were significantly affected by Zn supplementation. The proportions of Zn in fungal Fcw and Fs were significantly affected by DSE-culture methods, and the Zn concentration in Fcw and Zn proportion in Fco were significantly affected by an interaction between Zn and DSE culture methods (Table S5). Compared with their respective Zn0 treatments, the Zn concentration in each subcellular fraction of the H93 mycelia increased significantly under Zn550 treatment both in co-cultured and in vitro conditions (Table S6). Although there was a significantly higher Zn concentration accumulated in mycelia (40.87%) in co-culture with maize compared to that in vitro, no obvious change in the concentration and proportion of toxic Zn in cell organelles under Zn550 supplementation was found. Conversely, more toxic Zn was accumulated in the cell walls of mycelia in co-culture than in the in vitro conditions (Table and S6). Compared with in vitro culture, the co-cultured mycelia had a significantly higher proportion of Zn (44.48%) in cell organelles under Zn0 supplementation, as well as a significantly higher proportion of Zn in fungal Fcw (38.25%), but a significantly lower proportion of fungal Fs (24.55%) under Zn0 supplementation (Table ).
Table 2. Zn proportion in subcellular fractions of H93 mycelium both in vitro and in co-cultured with maize under Zn0 and Zn550 conditions.
Discussion
In the present study, the fungus-root association between E. pisciphila and maize roots was set up, and obviously confirmed by the colonization of typical DSE structures of melanized septate hyphae and microsclerotia in the inoculation treatments, which occurred to a greater degree than in the original host plant of Arundinella bengalensis (Poaceae) (Zhang et al. Citation2008). Our results suggest the root colonization of H93 without host specificity. Recently, Li et al. (Citation2019) also reported a similar phenomenon in which three DSE strains isolated from Hedysarum scoparium not only successfully colonized the roots of both Glycyrrhiza uralensis and Z. mays, but also functioned as they did in their isolated host plant. Similarly, five fungal strains of DSE isolated from non-hyperaccumulator poplar roots also successfully colonized in the roots and promoted phytoextraction of Cd and Zn by the hyperaccumulator Noccaea caerulescens (Yung et al. Citation2021). Furthermore, in our study, Zn550 stress treatments significantly increased the fungal colonization intensity. This result was in accordance with the previous reports that the elevated metal supplementations in the culture medium resulted in the increased DSE colonization in plant roots (Wang et al. Citation2016; Zhu et al. Citation2018; Haruma et al. Citation2021).
Interestingly, we noted that fungal inoculated maize roots significantly reduced the uptake of toxic Zn compared to in the non-inoculated treatments under Zn550 supplementation. Increasing data showed the toxicity of excessive heavy metals to organisms was not only dependent on the total concentrations, but also on their subcellular distribution (Pourrut et al. Citation2011; Zhao et al. Citation2015b; Ban et al. Citation2021). In the present study, the proportion of Zn in the cell organelles of H93 was also increased under co-culture compared with in vitro culture under Zn0 supplementation, which was beneficial to the growth of H93 as Zn is an essential element. Meanwhile, under Zn550 treatment, a large percentage of Zn was accumulated in the cell wall and soluble fractions (including the vacuoles) of H93 mycelia, and the proportion of Zn in the fungal cell organelles was reduced in the co-cultured conditions compared with the in vitro culture. Therefore, compared with in vitro culture, co-culture with maize decreased the toxicity of excessive Zn to H93 growth under Zn550 treatment, which provided support for our hypothesis that a mutual association between the E. pisciphila H93 and its host plants may occur under toxic Zn stress. Researches have argued that the binding of metals to fungal cell walls and compartmentalization in vacuoles (both in spores and hyphae) reduced the toxicity of excessive ions (Zn, Cu, and Cd) to diverse cell organelles (Li et al. Citation2021; Priyadarshini et al. Citation2021). And Gonzalez-Guerrero et al. (Citation2008) suggested these may be the essential mechanisms of the extraradical mycelia and spores of Glomus intraradices for metal detoxification. We also demonstrated that the compartmentalization of metals (Pb, Zn, and Cd) in vacuoles via the functions of EpABC transporters was an important metal detoxification pathway for H93 (Cao et al. Citation2019). Therefore, the altered accumulation of heavy metals in the cell walls and vacuoles may lower the entry of metal ions into the fungal protoplast, thereby conferring the tolerance that allows species to survive and thrive in metal-contaminated environments.
In the present study, we found that under Zn0 treatment, there was no difference between H93 mycelia Zn concentration in co-culture and in vitro culture. In the Zn550 stress treatments, the concentration of Zn in H93 mycelia was significantly higher in co-culture compared with in vitro culture. Obviously, the sources of carbon of H93 greatly varied between the two culture conditions, i.e. in vitro by saprophytic nutrition, and in co-culture with plants by biotrophic nutrition, which resulted in the altered Zn accumulation in E. pisciphila hyphae. Kim et al. (Citation2003) also found that glucose addition increased the growth of the three ectomycorrhizal fungi, and they argued that detoxification/repair mechanisms of fungi for heavy metals may be associated with a significant metabolic C cost. In addition, such root-associated fungi, e.g. arbuscular mycorrhizal fungus Rhizophagus intraradices could significantly minimize hazards of As contamination via the restored As-mediated alteration in sugar metabolism by modulating the activities of starch phosphorylase, α-amylase, and etc. (Gupta et al. Citation2021). In addition, plant roots secrete numerous exudates including sugars, proteins, and organic acids, which are responsible for the high concentrations of metal stresses (Cai et al. Citation2013). Presumably, these varied exudates may have also functioned as carbon sources and thus altered the metabolic pathways linked to metal metabolism and the absorption and accumulation of Zn in the H93 extraradical hyphae. Interestingly, the increased Zn accumulation in the extraradical hyphae co-cultured with maize roots further contributed to the reduced Zn accumulation in its host maize, thus alleviating the Zn stress and promoting the host plant growth. This association therefore enabled both the fungus and its host plant to grow in the metal-stressed and unfavorable growth conditions, providing a strong ecological advantage compared to the plant alone or fungus alone.
Our previous studies have found that the inoculation of H93 enhanced the tolerance of maize to Pb, Zn, and Cd by enhancing the activity of maize antioxidant enzymes (Li et al. Citation2011; Wang et al. Citation2016). H93 also reduced the absorption of Cd and changed its subcellular distribution in maize roots by modulating the expression of metal metabolism-associated genes and metabolic pathways, thus enhancing the tolerance of maize to Cd (Shen et al. Citation2020). In the present study, we further found that under nutritional Zn supplementation (Zn0), H93 inoculation increased the Zn content in the maize shoots and roots compared with the non-inoculated controls, while under Zn550 stress treatment, H93 inoculation decreased Zn accumulation in maize shoots and roots. Furthermore, under Zn0 treatment, the proportion of Zn in the cell organelles and soluble fraction of maize shoots and roots was increased by H93 inoculation compared to non- inoculation. Meanwhile, the reversed trends were observed in maize roots under Zn-stressed conditions, which further supported the potential mutual association between the plants and E. pisciphila H93 under toxic Zn stress. Additionally, DSE could also form a mutualistic symbiosis by nutrient exchange, e.g. Humicola sp. providing numerous amino acids, e.g. Val, Leu and Phe and auxin-like compound in exchange for the photosynthetic carbon of host plant Chinese cabbage (Khastini and Jannah Citation2021).
Conclusions
DSEs are a group of multifunctional fungi that ubiquitously colonize numerous plant root systems. Their relationship with their host plants is still ambiguous. We reported a case study of an HM-tolerant DSE strain (H93) with its host plant maize to gain insight into their relationships under both nutritional Zn0 and stressed Zn550 conditions. Our results showed that the mutualistic relationships occurred between E. pisciphila and its host maize both under the nutritional Zn0 and stressed Zn550 conditions. In exchange for photosynthetic products, DSE bioaugmented the Zn tolerance of maize under Zn550 treatments via the reduced Zn accumulation in maize, and the enhanced Zn compartmentation both in cell walls and vacuoles (Haruma et al. Citation2021). Likewise, under the nutritional Zn0 conditions, DSE conversely increased the accumulation of nutritional Zn and promoted the availability of active Zn in numerous cell organelles in maize. Meantime, the co-cultured maize also enhanced the Zn tolerance of H93 via the accumulation of a high percentage of Zn in the cell walls and soluble fractions of H93 mycelia, though more toxic Zn in the culture broth was accumulated in the extraradical hyphae of E. pisciphila. Our results suggest the potentially mutual association of E. pisciphila and its host plants, by trading carbon for increased abiotic stress tolerance, which is beyond a carbon economy: nutrient trade in a mycorrhizal symbiosis (Smith et al. Citation2009). Understanding the optimum plant-DSE association will help shed light on new perspectives for increasing the accumulation of Zn in crops in non-polluted soil and reducing the accumulation of a specific metal pollutant in crops, even when grown in HM-contaminated soils. However, we must keep in mind that more research is required to validate the interactions of the various crop species, metal species, and the abundant DSE resources both in the greenhouse and field trials (Yin et al. Citation2021).
Authors’ contributions
Conceptualization, T.L. and Z.Z.; methodology, T.L. and Z.Z.; investigation, L.Z. and D.L.; writing (original draft preparation), D.L.; writing (review and editing), T.L. and Z.Z.; funding acquisition, T.L. and Z.Z.
Ethical approval
This article is original and has not been published elsewhere. All of the data generated or analyzed during this study are included in this published article, and all of the authors have read and agreed to the published version of the manuscript. The authors confirm that there are no ethical issues in the publication of the manuscript.
Acknowledgments
The authors gratefully acknowledge Fangdong Zhan (College of Resources and Environment, Yunnan Agricultural University, China) for his help in the metal analysis.
Disclosure statement
No potential conflict of interest was reported by the author(s).
Data availability statement
Data for the manuscript have been deposited in the generalist repositories of Mendeley Data (https://data.mendeley.com/datasets/wg4wjhdjmv/1).
Additional information
Funding
References
- Abid R, Manzoor M, De Oliveira LM, Da Silva E, Rathinasabapathi B, Rensing C, Mahmood S, Liu X, Ma LQ. 2019. Interactive effects of As, Cd and Zn on their uptake and oxidative stress in As-hyperaccumulator Pteris vittata. Environ Pollut. 248:756–762. doi:10.1016/j.envpol.2019.02.054.
- Affholder MC, Pricop AD, Laffont-Schwob I, Coulomb B, Rabier J, Borla A, Prudent P. 2014. As, Pb, Sb and Zn transfer from soil to root of wild rosemary: Do native symbionts matter? Plant Soil. 382(1):219–236. doi:10.1007/s11104-014-2135-4.
- Ban Y, Xiao Z, Wu C, Lv Y, Meng F, Wang J, Xu Z. 2021. The positive effects of inoculation using arbuscular mycorrhizal fungi and/or dark septate endophytes on the purification efficiency of CuO-nanoparticles-polluted wastewater in constructed wetland. J Hazard Mater. 126095. doi:10.1016/j.jhazmat.2021.126095.
- Ban YH, Xu ZY, Yang YR, Zhang HH, Chen H, Tang M. 2017. Effect of dark septate endophytic fungus Gaeumannomyces cylindrosporus on plant growth, photosynthesis and Pb tolerance of maize (Zea mays L.). Pedosphere. 27(2):283–292. doi:10.1016/S1002-0160(17)60316-3.
- Barberis L, Chevalier W, Toussaint ML, Binet P, Piola F, Michalet S. 2020. Responses of the species complex Fallopia × bohemica to single-metal contaminations to Cd, Cr or Zn: growth traits, metal accumulation and secondary metabolism. Environ Monit Assess. 192(11):1–19. doi:10.1007/s10661-020-08627-1.
- Berch SM, Kendrick B. 1982. Vesicular-arbuscular mycorrhizae of southern Ontario ferns and fern-allies. Mycologia. 74(5):769–776. doi:10.2307/3792863.
- Brown PH, Cakmak I, Zhang QL. 1993. Zinc in soils and plants: form and function of Zinc plants, springer. Dordrecht. 93–106. doi:10.1007/978-94-011-0878-2_7.
- Cai ZZ, Kastell A, Speiser C, Smetanska I. 2013. Enhanced resveratrol production in Vitis vinifera cell suspension cultures by heavy metals without loss of cell viability. Appl Biochem Biotech. 171(2):330–340. doi:10.1007/s12010-013-0354-4.
- Cao GH, He S, Chen D, Li T, Zhao ZW. 2019. EpABC genes in the adaptive responses of Exophiala pisciphila to metal stress: functional importance and relation to metal tolerance. Appl Environ Microb. 85(23):e01844–19. doi:10.1128/AEM.01844-19.
- Diao YH, Li T, Zhao ZW. 2013. Zinc accumulation characteristics of two Exophiala strains and their antioxidant response to Zn2+ stress. J Environ Prot Ecol. 4:12–19. doi:10.4236/jep.2013.44A003.
- Galati S, Gullì M, Giannelli G, Furini A, DalCorso G, Fragni R, Buschini A, Visioli G. 2021. Heavy metals modulate DNA compaction and methylation at CpG sites in the metal hyperaccumulator Arabidopsis halleri. Environ Mol Mutagen. 62(2):133–142. doi:10.1002/em.22421.
- Gonzalez-Guerrero M, Melville LH, Ferrol N, Lott J, Azcón-Aguilar C, Peterson RL. 2008. Ultrastructural localization of heavy metals in the extraradical mycelium and spores of the arbuscular mycorrhizal fungus Glomus intraradices. Can J Microbiol. 54(2):103–110. doi:10.1139/w07-119.
- Gucwa-Przepióra E, Nadgórska-Socha A, Fojcik B, Chmura D. 2016. Enzymatic activities and arbuscular mycorrhizal colonization of Plantago lanceolata and Plantago major in a soil root zone under heavy metal stress. Environ Sci Pollut R. 23(5):4742–4755. doi:10.1007/s11356-015-5695-9.
- Gupta S, Thokchom SD, Kapoor R. 2021. Arbuscular mycorrhiza improves photosynthesis and restores alteration in sugar metabolism in triticum aestivum L. grown in arsenic contaminated soil. Front Plant Sci. 12:334. doi:10.3389/fpls.2021.640379.
- Haruma T, Yamaji K, Masuya H. 2021. Phialocephala fortinii increases aluminum tolerance in Miscanthus sinensis growing in acidic mine soil. Lett Appl Microbiol. doi:10.1111/lam.13514.
- Hoagland DR, Arnon DI. 1950. The Water-Culture Method for Growing Plants without Soil. Cal agric exp st circ. 347.2nd edit. doi:10.1016/S0140-6736(00)73482-9.
- Hui FQ, Liu J, Gao QK, Lou BG. 2015. Piriformospora indica confers cadmium tolerance in Nicotiana tabacum. Environ Sci Technol. 37(11):184–191. doi:10.1016/j.jes.2015.06.005.
- Jumpponen A. 2001. Dark septate endophytes-Are they mycorrhizal? Mycorrhiza. 11(4):207–211. doi:10.1007/s005720100112.
- Jumpponen A, Trappe JM. 1998. Dark septate endophytes: A review of facultative biotrophic root-colonizing fungi. New Phytol. 140(2):295–310. doi:10.1046/j.1469-8137.1998.00265.x.
- Khastini RO, Jannah R. 2021. Potential contribution of dark-septate endophytic fungus isolated from Pulau dua nature reserve, Banten on growth promotion of Chinese cabbage. In 2nd and 3rd International Conference on Food Security Innovation (ICFSI 2018-2019). Atlantis Press, 83–89. doi:10.2991/absr.k.210304.015.
- Khullar S, Reddy MS. 2018. Ectomycorrhizal fungi and its role in metal homeostasis through metallothionein and glutathione mechanisms. Curr Biotech. 7(3):231–241. doi:10.2174/2211550105666160531145544.
- Kim CG, Power SA, Bell J. 2003. Effects of cadmium on growth and glucose utilisation of ectomycorrhizal fungi in vitro. Mycorrhiza. 13(4):223–226. doi:10.1007/s00572-003-0235-8.
- Li X, He C, He XL, Su F, Hou LF, Ren Y, Hou YT. 2019. Dark septate endophytes improve the growth of host and non-host plants under drought stress through altered root development. Plant Soil. 439(1):259–272. doi:10.1007/s11104-019-04057-2.
- Li X, Lan X, Feng X, Luan X, Cao X, Cui Z. 2021. Biosorption capacity of Mucor circinelloides bioaugmented with Solanum nigrum L. for the cleanup of lead, cadmium and arsenic. Ecotox Environ Safe. 212:112014. doi:10.1016/j.ecoenv.2021.112014.
- Li T, Liu MJ, Zhang XT, Zhang HB, Sha T, Zhao ZW. 2011. Improved tolerance of maize (Zea mays L.) to heavy metals by colonization of a dark septate endophyte (DSE) Exophiala pisciphila. Sci Total Environ. 409(6):1069–1074. doi:10.1016/j.scitotenv.2010.12.012.
- Likar M, Regvar M. 2013. Isolates of dark septate endophytes reduce metal uptake and improve physiology of Salix caprea L. Plant Soil. 370(1):593–604. doi:10.1007/s11104-013-1656-6.
- Mani D, Kumar C, Patel NK. 2015. Integrated micro-biochemical approach for phytoremediation of cadmium and zinc contaminated soils. Ecotox Environ Safe. 111:86–95. doi:10.1016/j.ecoenv.2014.09.019.
- Mcgonigle TP, Miller MH, Evans DG, Fairchild GL, Swan JA. 1990. A new method which gives an objective measure of colonization of roots by vesicular-arbuscular mycorrhizal fungi. New Phytol. 115(3):495–501. doi:10.2307/2556652.
- Pourrut B, Shahid M, Dumat C, Winterton P, Pinelli E. 2011. Lead uptake, toxicity, and detoxification in plants. Rev Environ Contam T. 213:113–136. doi:10.1007/978-1-4419-9860-6_4.
- Priyadarshini E, Priyadarshini SS, Cousins BG, Pradhan N. 2021. Metal-Fungus interaction: review on cellular processes underlying heavy metal detoxification and synthesis of metal nanoparticles. Chemosphere. 129976. doi:10.1016/j.chemosphere.2021.129976.
- Rasafi TE, Nouri M, Bouda S, Haddioui A. 2016. The effect of Cd, Zn and Fe on seed germination and early seedling growth of wheat and bean. Ekológia (Bratislava). 35(3):213–223. doi:10.1515/eko-2016-0017.
- Rehman R, Asif M, Cakmak I, Ozturk L. 2021. Differences in uptake and translocation of foliar-applied Zn in maize and wheat. Plant Soil. 462(1):235–244. doi:10.1007/s11104-021-04867-3.
- Shadmani L, Jamali S, Fatemi A. 2021a. Isolation, identification, and characterization of cadmium-tolerant endophytic fungi isolated from barley (Hordeum vulgare L.) roots and their role in enhancing phytoremediation. Braz J Microbiol. 1–10. doi:10.1007/s42770-021-00493-4.
- Shadmani L, Jamali S, Fatemi A. 2021b. Effects of root endophytic fungus, Microdochium bolleyi on cadmium uptake, translocation and tolerance by Hordeum vulgare L. Biologia. 76(2):711–719. doi:10.2478/s11756-020-00598-5.
- Shen M, Schneider H, Xu RB, Cao GH, Zhang HB, Li T, Zhao ZW. 2020. Dark septate endophyte enhances maize cadmium (Cd) tolerance by the remodeled host cell walls and the altered Cd subcellular distribution. Environ Exp Bot. 172:104000. doi:10.1016/j.envexpbot.2020.104000.
- Smith FA, Grace EJ, Smith SE. 2009. More than a carbon economy: nutrient trade and ecological sustainability in facultative arbuscular mycorrhizal symbioses. New Phytol. 182(2):347–358. doi:10.1111/j.1469-8137.2008.02753.x.
- Szopinski M, Sitko K, Gieron Z, Rusinowski S, Corso M, Hermans C, Verbruggen N, Malkowski E. 2019. Toxic effects of Cd and Zn on the photosynthetic apparatus of the Arabidopsis halleri and Arabidopsis arenosa Pseudo-Metallophytes. Front Plant Sci. 10:748. doi:10.3389/fpls.2019.00748.
- Teng Y, Du XZ, Wang T, Mi CY, Yu HY, Zou LY. 2018. Isolation of a fungus Penicillium sp. with zinc tolerance and its mechanism of resistance. Arch Microbiol. 200(1):159–169. doi:10.1007/s00203-017-1430-x.
- Wang JL, Li T, Liu GY, Smith JM, Zhao ZW. 2016. Unraveling the role of dark septate endophyte (DSE). colonizing maize (Zea mays) under cadmium stress: physiological, cytological and genic aspects. Sci Rep-uk. 6(1):1–12. doi:10.1038/srep22028.
- Yin Z, Zhang Y, Hu N, Shi Y, Li T, Zhao Z. 2021. Differential responses of 23 maize cultivar seedlings to an arbuscular mycorrhizal fungus when grown in a metal-polluted soil. Sci Total Environ. 148015. doi:10.1016/j.scitotenv.2021.148015.
- Yung L, Blaudez D, Maurice N, Azou-Barré A, Sirguey C. 2021. Dark septate endophytes isolated from non-hyperaccumulator plants can increase phytoextraction of Cd and Zn by the hyperaccumulator Noccaea caerulescens. Environ Sci Pollut R. 1–14. doi:10.1007/s11356-020-11793-x.
- Zhan FD, He YM, Li Y, Li T, Yang YY, Toor GS, Zhao ZW. 2015a. Subcellular distribution and chemical forms of cadmium in a dark septate endophyte (DSE). Exophiala pisciphila. Environ Sci Pollut R. 22(22):17897–17905. doi:10.1007/s11356-015-5012-7.
- Zhan FD, He YM, Li T, Yang YY, Toor GS, Zhao ZW. 2015b. Tolerance and antioxidant response of a dark septate endophyte (DSE), Exophiala pisciphila, to cadmium stress. B Environ Contam Tox. 94(1):96–102. doi:10.1007/s00128-014-1401-8.
- Zhang XF, Hu ZH, Yan TX, Lu RR, Peng CL, Li SS, Jing YX. 2019. Arbuscular mycorrhizal fungi alleviate Cd phytotoxicity by altering Cd subcellular distribution and chemical forms in Zea mays. Ecotox Environ Safe. 171:352–360. doi:10.1016/j.ecoenv.2018.12.097.
- Zhang G, Su L, Li T, Li MR, He YM, Qin L. 2020. Effects of nitrogen on mineral nutrients and cadmium accumulation in a strain of DSE mycelium under cadmium stress. IOP Conf Ser: Earth Environ Sci. 446(3):032082. doi:10.1088/1755-1315/446/3/032082.
- Zhang YJ, Zhang Y, Liu MJ, Shi XD, Zhao ZW. 2008. Dark septate endophyte (DSE) fungi isolated from metal polluted soils: their taxonomic position, tolerance, and accumulation of heavy metals in vitro. J Microbiol. 46(6):624–632. doi:10.1007/s12275-008-0163-6.
- Zhao DK, Li T, Shen M, Wang JL, Zhao ZW. 2015a. Diverse strategies conferring extreme cadmium (Cd) tolerance in the dark septate endophyte (DSE), Exophiala pisciphila: evidence from RNA-seq data. Microbiol Res. 170:27–35. doi:10.1016/j.micres.2014.09.005.
- Zhao YF, Wu JF, Shang DR, Ning JS, Zhai YX, Sheng XF, Ding HY. 2015b. Subcellular distribution and chemical forms of cadmium in the edible seaweed, porphyra yezoensis. Food Chem. 168:48–54. doi:10.1016/j.foodchem.2014.07.054.
- Zhu LL, Li T, Wang CJ, Zhang XR, Xu LJ, Xu RB, Zhao ZW. 2018. The effects of dark septate endophyte (DSE) inoculation on tomato seedlings under Zn and Cd stress. Environ Sci Pollut R. 25(35):35232–35241. doi:10.1007/s11356-018-3456-2.
- Zulfiqar U, Hussain S, Ishfaq M, Matloob A, Ali N, Ahmad M, Ali N, Ahmad M, Alyemeni MN, Ahmad P. 2020. Zinc-induced Effects on productivity, Zinc use efficiency, and grain biofortification of bread wheat under different tillage permutations. Agronomy. 10(10):1566. doi:10.3390/agronomy10101566.