Abstract
Alu elements, the most abundant retrotransposed elements in the human genome, are commonly located in introns and affect alternative splicing. We previously showed that an intronic antisense Alu element enhanced alternative splicing in a minigene model. The RNA splicing experiment was performed using a minigene consisting of exons 9–11 of ACAT1 with an antisense AluSx inserted into intron 10 in a pCAGGS vector. Here, we explored the sequence in the intronic antisense AluSx that affects downstream exon skipping. Our RNA splicing analysis with antisense AluSx deletion mutants confirmed a short sequence of 16 bp within the right arm of antisense AluSx that resulted in exon skipping. Our findings help clarify the mechanism of RNA splicing by Alu elements.
Introduction
Alternative splicing is an important mechanism for increasing the functional complexity and protein diversity in humans (Wang et al. Citation2008; Nilsen and Graveley Citation2010). Most human genes are transcribed by alternative splicing in a tissue-dependent or developmental stage-dependent manner (Stamm et al. Citation2005; Pan et al. Citation2008; Wang et al. Citation2008). Alu elements are the most abundant repetitive element in the human genome, composing more than 10% of the genome, because of their high copy number (International Human Genome Sequencing Consortium Citation2001). Alu elements are one of the transposable elements in primates and contribute to genomic and transcriptomic diversity in evolution (Deininger and Batzer Citation1999; Hasler et al. Citation2007). Although the mechanisms underlying the impact of Alu elements on alternative splicing are poorly understood, some studies showed that Alu elements affect alternative splicing by exonization of the Alu sequence itself (Sorek et al. Citation2002; Lev-Maor et al. Citation2003; Gal-Mark et al. Citation2008; Schwartz et al. Citation2009; Lin et al. Citation2016) and that intronic Alu elements play a role in RNA splicing (Lev-Maor et al. Citation2008; Pastor and Pagani Citation2011; Nakama et al. Citation2018). A typical Alu element is ∼300 bp in length, consisting of two similar but distinct monomers, the left and the right arms, joined by an A-rich linker and followed by a poly(A) tail (Batzer and Deininger Citation2002). The right arm contains a 31 bp insert that is not present in the left arm (Gal-Mark et al. Citation2008). AluSx is one of the major Alu subfamilies that contains sequences present in 80% of all Alu families and has been shown to harbor a large number of random mutations (Batzer et al. Citation1996). We previously established a minigene model using AluSx as a typical example of an Alu element to examine RNA splicing. We found that intronic antisense AluSx affects exon skipping in a minigene model consisting of exons 9–11 of the acetyl-CoA acetyltransferase 1 gene (ACAT1), especially when the splice acceptor site is suboptimal (Nakama et al. Citation2018). Here, we aimed to identify the minimal sequence element in intronic AluSx that affects RNA splicing (exon skipping) in the splicing model of ACAT1.
Materials and methods
Minigene constructs
We constructed a basic minigene construct based on a previously established minigene construct containing truncated intron 9 and truncated intron 10 of ACAT1 (Fukao et al. Citation2010; Nakama et al. Citation2018). Our basic minigene construct contained a fragment of wild-type human ACAT1 (exons 9–11) and an AluSx fragment in the pCAGGS vector (Figure (A)). Antisense AluSx, originally located in ACAT1 intron 5, was inserted into a Hind III site 226 bp upstream from the beginning of exon 10. We generated 29 antisense AluSx deletion constructs in this study using the KOD-Plus-Mutagenesis kit (TOYOBO Co., Ltd., Osaka, Japan). The primers are listed in the Supplementary Table. We confirmed the minigene constructs by direct sequencing.
Figure 1. Splicing assays using the ACAT1 minigene with AluSx deletions. (A) Schematic of the ACAT1 minigene including exon 9 to exon 11 of ACAT1. White boxes and white arrows indicate exons and the original Alu elements, respectively. Full AluSx or AluSx deletions were inserted in an antisense orientation into ACAT1 intron 9 at 226 bp upstream of exon 10. (B) The structure of full-length AluSx and the 10 antisense AluSx deletion constructs. (C) Minigene splicing experiments with the deletion constructs. Mock experiments were performed with an ACAT1 minigene construct without antisense AluSx. Normal splicing (exon 10 inclusion) and aberrant splicing (exon 10 skipping) generated 309 and 244 bp fragments, respectively.
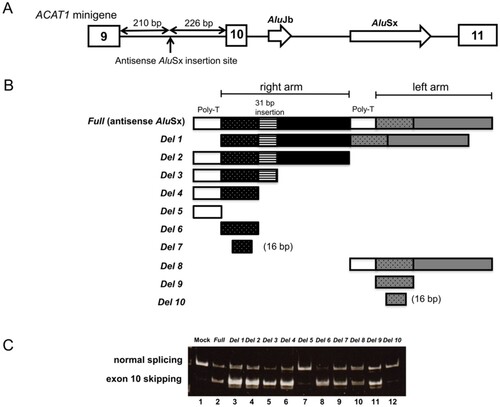
Minigene splicing experiment
SV40-transformed fibroblasts from a patient (GK03) with mitochondrial acetoacetyl-CoA thiolase deficiency were cultured (Fukao et al. Citation1993). Each expression vector was transfected into the SV40-transformed fibroblasts with Lipofectamine 2000 (Life Technologies, Carlsbad, CA, USA). RNA was harvested 48 h after transfection and was reverse-transcribed by the strand-specific β-glo2 primer and oligo(dT)12-18 primer. Chimeric cDNAs were amplified with Ex9 and β-glo3 primers (Fukao et al. Citation2010). We electrophoresed the PCR products on a 5% polyacrylamide gel; normal splicing resulted in a 309 bp fragment, while exon 10 skipping resulted in a 244 bp fragment. The experimental details were described in a previous paper (Nakama et al. Citation2018).
cDNAs with normal splicing or with exon 10 skipping produced in the splicing experiments (Figure (C), ‘Full’) were purified using the Geneclean II kit (BIO 101, Vista, CA, USA) and subcloned separately into the pGEM-T easy vector (Promega Corp., Madison, WI, USA). The normal splicing and exon skipping plasmids were mixed in nine serial molar ratios (10:0, 9:1, 8:2, 6:4, 5:5, 4:6, 2:8, 1:9, and 0:10), and the mixtures (about 5 × 10−5 pmol in total) were used as PCR controls for semi-quantitative evaluation (Supplementary Figure 1).
Results
We previously showed alternative splicing when antisense AluSx was inserted in intron 9 of the ACAT1 minigene model. The antisense AluSx caused exon 10 skipping in the partial transcripts (Nakama et al. Citation2018) (Figure (C), lane 2, ‘Full’). Based on these data, we presumed there may be a potential sequence within antisense AluSx that induces the downstream exon 10 skipping.
To identify this sequence, we performed minigene splicing experiments using deletion constructs of antisense AluSx (Figure (A, B)). Mock reactions were performed using a ACAT1 minigene construct without AluSx (Figure (C), lane 1). For semi-quantitative evaluation of the level of skipping, we performed PCR using mixtures of plasmid DNAs with and without exon 10 skipping at various molar ratios (10:0, 9:1, 8:2, 6:4, 5:5, 4:6, 2:8, 1:9, and 0:10) (Supplementary Figure 1). We defined exon skipping at levels over those observed with the 5:5 mixture. We first focused on the effect of the poly-T sequence, as a C/T stretch can serve as poly-pyrimidine tracts to promote recognition of cryptic splice sites (Gal-Mark et al. Citation2008). However, the construct lacking two poly-T sites (‘Del 1’) still induced exon 10 skipping (Figure (C)). Moreover, both constructs that contained just the left arm or right arm (Del 2 and Del 8) also showed exon 10 skipping. All constructs with right arm deletions, Del 1, Del 2, Del 3, Del 4 and Del 6, showed exon 10 skipping. Notably, Del 5, which consisted of just a poly-T sequence, showed mostly normal splicing (Figure (C), lane 7). To further narrow down the potential sequence that induces exon 10 skipping, we performed splicing experiments using additional deletion constructs based on Del 6 of the right arm, as the level of exon skipping was higher with the right arm sequence compared with that of the left arm (Figure (C), lane 8 vs. lane 11). Del 7 in the right arm was determined as the shortest sequence that impacted exon 10 skipping (Figure (A), lane 10 and Figure (B), lane 1). Del 7, which consisted of 16 nucleotides, had exon 10 skipping corresponding approximately to the 5:5 mixture (Figure (C), lane 9). The Del 10 left arm construct, which was a 16 nucleotide sequence counterpart of Del 7, the most shortened region in the left arm, showed a low level of exon 10 skipping (Figure (C), lane 12 and Figure (C), lane 7); the band intensity with the Del 10 construct was comparable to that observed with the 9:1 mixture. The amount of exon 10 skipping with the Del 10 sequence of the left arm was much smaller than that of the Del 7 of the right arm. We, therefore, conclude that 16 nucleotides in the right arm region of the AluSx element are sufficient for exon 10 skipping in our minigene model.
Discussion
In this study, we identified a minimal sequence in intronic Alu that affects downstream exon skipping using a minigene splicing model consisting of ACAT1 exons 9–11. We previously reported that exon 10 skipping was induced by Alus inserted in intron 9 using the same minigene model (Nakama et al. Citation2018). Here, we performed the splicing experiments with deletion constructs and identified a 16-bp sequence in the antisense AluSx right arm that promoted exon 10 skipping. Although we have not yet elucidated the mechanism of exon skipping by Alu, we provide evidence this 16-bp sequence in Alu keeps a potential for exon skipping. Regarding potential mechanisms, this sequence may have served as binding sites for RNA splicing factors required for exon skipping. And/or double-stranded formation with opposite-orientation Alu in intron 10 might promote exon 10 skipping because exon 9 and exon 11 are closer. A construct with this sequence and that without this sequence are both sufficient conditions for exon skipping (Supplementary Figure 2). Then we consider this sequence was not essential, however, just keep a potential for exon skipping at the same level as that of a full length Alu. Moreover, why the left arm construct containing the 16-bp sequence did not induce exon skipping is unclear. One possible explanation of the different effects on exon skipping between the left arm and the right arm is that the consequence sequence is not well conserved between the arms and share just 7 bp within the 16 bp (Supplementary Figure 3). This result also supports the hypothesis that the exon skipping effect by Alu is dependent on the sequence itself; the nucleotide sequence or type of DNA nucleotides seem to be required for exon skipping rather than just a certain length of nucleotides. Our findings suggested only 16 nucleotides could substitute for the full length Alu, with a sequence of 300 bp, in inducing the exon skipping effect. These results represent a new finding in the clarification of the mechanism of Alu elements.
Supplemental Material
Download Zip (277.3 KB)Acknowledgments
We gratefully appreciate the late Professor Toshiyuki Fukao, who established the minigene splicing model of this research and gave us this research theme. We thank Gabrielle White Wolf, PhD, from Edanz Group (https://en-author-services.edanzgroup.com/ac) for editing a draft of this manuscript.
Disclosure statement
No potential conflict of interest was reported by the author(s).
Data availability statement
The data that support the findings of this study are available at the figshare repository (https://figshare.com/) at https://doi.org/10.6084/m9.figshare.16531881.v1. Nucleotide sequences of antisense AluSx in this study are available at https://doi.org/10.6084/m9.figshare.16531659.
References
- Batzer MA, Arcot SS, Phinney JW, Alegria-Hartman M, Kass DH, Milligan SM, Kimpton C, Gill P, Hochmeister M, Ioannou PA, et al. 1996. Genetic variation of recent Alu insertions in human populations. J Mol Evol. 42:22–29.
- Batzer MA, Deininger PL. 2002. Alu repeats and human genomic diversity. Nat Rev Genet. 3:370–379.
- Deininger PL, Batzer MA. 1999. Alu repeats and human disease. Mol Genet Metab. 67:189–193.
- Fukao T, Horikawa R, Naiki Y, Tanaka T, Takayanagi M, Yamaguchi S, Kondo N. 2010. A novel mutation (c.951C>T) in an exonic splicing enhancer results in exon 10 skipping in the human mitochondrial acetoacetyl-CoA thiolase gene. Mol Genet Metab. 100:339–344.
- Fukao T, Yamaguchi S, Scriver CR, Dunbar G, Wakazono A, Kano M, Orii T, Hasimoto T. 1993. Molecular studies of mitochondrial acetoacetyl-coenzyme A thiolase deficiency in the two original families. Hum Mutat. 2:214–220.
- Gal-Mark N, Schwartz S, Ast G. 2008. Alternative splicing of Alu exons–two arms are better than one. Nucleic Acids Res. 36:2012–2023.
- Hasler J, Samuelsson T, Strub K. 2007. Useful ‘junk’: Alu RNAs in the human transcriptome. Cell Mol Life Sci. 64:1793–1800.
- International Human Genome Sequencing Consortium. 2001. Initial sequencing and analysis of the human genome. Nature. 409:860–921.
- Lev-Maor G, Ram O, Kim E, Sela N, Goren A, Levanon EY, Ast G. 2008. Intronic Alus influence alternative splicing. PLoS Genet. 4:1–12.
- Lev-Maor G, Sorek R, Shomron N, Ast G. 2003. The birth of an alternatively spliced exon: 3’ splice-site selection in Alu exons. Science. 300:1288–1291.
- Lin L, Jiang P, Park JW, Wang J, Lu Z, Lam MPY, Ping P, Xing Y. 2016. The contribution of Alu exons to the human proteome. Genome Biol. 17:15.
- Nakama M, Otsuka H, Ago Y, Sasai H, Abdelkreem E, Aoyama Y, Fukao T. 2018. Intronic antisense Alu elements have a negative splicing effect on the inclusion of adjacent downstream exons. Gene. 664:84–89.
- Nilsen TW, Graveley BR. 2010. Expansion of the eukaryotic proteome by alternative splicing. Nature. 463:457–463.
- Pan Q, Shai O, Lee LJ, Frey BJ, Blencowe BJ. 2008. Deep surveying of alternative splicing complexity in the human transcriptome by high-throughput sequencing. Nat Genet. 40:1413–1415.
- Pastor T, Pagani F. 2011. Interaction of hnRNPA1/A2 and DAZAP1 with an Alu-derived intronic splicing enhancer regulates ATM aberrant splicing. PLoS One. 6:e23349.
- Schwartz S, Gal-Mark N, Kfir N, Oren R, Kim E, Ast G. 2009. Alu exonization events reveal features required for precise recognition of exons by the splicing machinery. PLoS Comput Biol. 5:e1000300.
- Sorek R, Ast G, Graur D. 2002. Alu-containing exons are alternatively spliced. Genome Res. 12:1060–1067.
- Stamm S, Ben-Ari S, Rafalska I, Tang Y, Zhang Z, Toiber D, Thnaraj TA, Soreq H. 2005. Function of alternative splicing. Gene. 344:1–20.
- Wang ET, Sandberg R, Luo S, Khrebtukova I, Zhang L, Mayr C, Kingsmore SF, Schroth GP, Burge CB. 2008. Alternative isoform regulation in human tissue transcriptomes. Nature. 456:470–476.