Abstract
The novel β-coronavirus, SARS-CoV-2, responsible for the coronavirus disease 2019 (COVID-19) emerged in China in December 2019. Due to its high transmission and infection rate, it has spread around the world and has transformed into a ravaging global pandemic with enormously unprecedented impacts globally on human, social, and economic health. Just like SARS-CoV and MERS-CoV, there is no specific antiviral drug for its treatment. The only available therapeutics are supportive and symptom-based. Thus, scientists are harnessing various strategies to expedite drug development. One such approach is drug repurposing through computational screening of phytocompounds, which leverages proteins that are essential for the entry, replication, pathogenesis, assembly, and release of SARS-CoV-2. Here, we review the available literature on molecular docking of phytoligands against SARS-CoV-2 integral proteins, in a bid to update our current knowledge and identify the most promising molecules. The overwhelming majority of the promising lead compounds are either phenolics or terpenoids. Furthermore, of the elucidated SARS-CoV-2 targets, the main protease (3CLpro) appears as one of the most attractive druggable targets. Notably, compounds such as rutin, quercetin, luteolin, neoandrographolide, curcumin, and others with evident anti-inflammatory benefits, in addition to their predicted anti-SARS-CoV-2 properties, deserve further studies to validate their activity.
Introduction
Coronaviruses (CoVs) are enveloped, positive-sense, single-stranded RNA (26-32 kb) viruses with biological sizes ranging from 65 to 125 nm (Li, Liu et al. Citation2020; Shereen et al. Citation2020). They belong to the subfamily Orthocoronavirinae within the family Coronaviridae. The four genera within this subfamily include Alphacoronavirus, Betacoronavirus, Deltacoronavirus, and Gammacoronavirus (Yang and Leibowitz Citation2015; Banerjee et al. Citation2019). The α- and β-coronaviruses have great medical and veterinary significance and contain 17 and 12 species, respectively. Members of the β-coronavirus include Human Coronavirus OC43 (HCoV-OC43), Severe Acute Respiratory Syndrome coronavirus (SARS-CoV), Human coronavirus HKU1 (HCoV-HKU1), Middle Eastern Respiratory Syndrome coronavirus (MERS-CoV), and Severe Acute Respiratory Syndrome coronavirus 2 (SARS-CoV-2) (Harapan et al. Citation2020). In November 2002, the SARS-CoV outbreak, with fatality rate of 11%, that later spread globally, emerged in Guangdong, China (Graham et al. Citation2013). Exactly a decade later (2012), the MERS outbreak, with an associated mortality rate of 37%, broke out in the Kingdom of Saudi Arabia and later spread to 27 other countries (Zumla et al. Citation2015).
Recently, a novel β-coronavirus, SARS-CoV-2, responsible for coronavirus disease 2019 (COVID-19) emerged in Wuhan, China, in December 2019. This virus belongs to β-coronavirus as SARS-CoV and MERS-CoV. It is genetically closely related to SARS-CoV with 82% similarity and has up to 50% similarity with MERS-CoV (Chan et al. Citation2020; Lu et al. Citation2020). Notably, SARS-CoV-2 has been reported to have high genetic similitude with a bat coronavirus, BatCoV RaTG13 (from the horseshoe bat), with 96.2% sequence homology (Zhou et al. Citation2020), possibly indicating that its reservoir host is bats. Due to its high infectivity and transmissibility, the virus has constituted a global pandemic and is a major public health menace. As of March 22, 2021, the virus has plagued more than 123 million individuals and resulted in 2,711,071 confirmed deaths in 235 countries (WHO, Citation2021). Typical symptoms of COVID-19 infection include sore throat, fever, dyspnoea, dry cough, headache, and pneumonia (Zhou et al. Citation2020). Just like SARS-CoV and MERS-CoV (de Wit et al. Citation2016; Zumla et al. Citation2015), there is no specific antiviral drug for the treatment of COVID-19, the only available therapeutic options are symptom-based or based on organ support (Li and Clercq Citation2020); thus, effective therapeutics are urgently needed.
Generally, four strategies are being employed to elucidate and develop potential anti-coronaviral agents. The first involves repurposing already available antivirals with proven efficacy against other viruses (e.g. remdesivir, an inhibitor of Ebola virus replication), with known dosage and clear metabolic characteristics. Remdesivir and favipiravir were found to exhibit great efficacy (Zhang, Xie et al. Citation2020). However, some of these antiviral therapeutics exhibit broad-spectrum activity and would not eliminate coronaviruses in a precise, targeted, and specific manner, and might be accompanied by non-negligible side effects (Chan et al. Citation2013). The second strategy involves the repositioning of already available non-antiviral drugs; for example, the anti-SARS-CoV-2 potential of an antimalarial drug, chloroquine, has been demonstrated (Gautret et al. Citation2020; Wang, Cao et al. Citation2020). The third method involves the study and characterisation of genomic, proteomic, and pathological features of coronaviruses in a bid to synthesise potential anti-coronaviral molecules from scratch (Omrani et al. Citation2014). Although agents discovered through this strategy would be more specific and exhibit better activity against coronaviruses, this procedure is often complex, time-consuming (requiring up to 10 years), and capital-intensive. Lastly, structure–activity relationship approach, involving computational (e.g. molecular docking) and high-throughput screening, is exploited to screen molecules (lead compounds), including phytocompounds, for their anti-coronaviral activity (Wu et al. Citation2020). This strategy is fast, affordable, and allows swift identification, characterisation, and screening of lead compounds while also limiting the use of animal models.
Plants and phytocompounds are receiving considerable attention as potential anti-coronaviral remedies due to their rich bioactive components and evident pharmacological activities which may guide the development of novel antiviral agents (Fischer et al. Citation2020; Jamiu et al. Citation2020). Importantly, many conventional drugs available today were designed using natural compounds as templates. Classic examples include the semisynthetic antimalarial drugs, chloroquine phosphate, and artemisinin obtained from Cinchona tree bark and Artemia annua, respectively. Moreover, emetine, an isoquinoline alkaloid, used as an amoebicidal drug is obtained from Cephaelis ipecacuanha (Weathers et al. Citation2014; Ganjhu et al. Citation2015). Other plant-derived semisynthetic drugs include quinidine, aspirin, morphine, digitalis, topotecan, taxol, and colchicine (Weaver Citation2014; Ganjhu et al. Citation2015). In addition, the use of Traditional Chinese medicines (TCM) for the management/treatment of SARS-CoV, Ebola virus, and H1N1 has been documented (Luo et al. Citation2019). In fact, the complementary use of TCM with conventional medicine for the successful treatment of COVID-19 has been exemplified (Wang, Chen et al. Citation2020).
Coronaviruses encode several proteins, some of which are essential for their life cycle (i.e. viral entry, replication, assembly, and release). Some of the most studied coronavirus proteins include 3-chymotrypsin-like protease (3CLpro), also referred to as main protease (Mpro), papain-like protease (PLpro), and spike glycoprotein (Sgp) (Shode et al. Citation2021). Both 3CLpro and PLpro are important for the proteolytic processing of viral polyproteins into functional units, coronaviral replication, and regulation of the immune system (Chen et al. Citation2020; Yu et al. Citation2020), while the Sgp is indispensable for viral entry into host cells (Isaacson and Ploegh Citation2009; Mukherjee et al. Citation2011). In addition, RNA-dependent RNA polymerase (RdRp) catalyses the replication of viral RNA from RNA templates, and the RdRp of SARS-CoV, MERS-CoV, and SARS-CoV-2 has similar sequences (Lung et al. Citation2020). Notably, due to the indispensable roles of 3CLpro, PLpro, Sgp, and RdRp in viral entry, replication, proliferation, and pathogenesis, they remain attractive targets for antiviral drug development. Unsurprisingly, scientists are already exploiting computational approaches, including molecular docking, to identify natural compounds capable of interacting with and modulating these viable targets. Such natural compounds have been speculated as potential inhibitors of their biological functions in silico; however, this must be well substantiated with appropriate laboratory and clinical tests. Here, we review the available scientific literature on the molecular docking-predicted anti-SARS-CoV-2 activity of phytocompounds, in a bid to update our current knowledge and identify the most promising lead compounds.
Materials and methods
Search strategy
The Preferred Reporting Items for Systematic Reviews and Meta-Analyses (PRISMA) was adopted (Moher et al. Citation2009). A search through literature databases, including PubMed and ScienceDirect, was made using keywords such as (‘COVID-19’ or ‘SARS-CoV-2’ or ‘coronavirus’) and (In silico or ‘molecular docking’ or ‘computational screening’ or ‘virtual screening’ or ‘drug repurposing’) and (‘Medicinal plants’ or ‘Natural products’ or ‘phytocompounds’).
Study selection
Only studies written in English that fulfilled the following criteria were included: (i) involved virtual screening of plant-derived natural products, (ii) involved natural inhibitors of SARS-CoV-2 from plant origin, and (iii) described the binding energy and binding sites of phytoligands. Articles excluded include (i) articles not written in English, (ii) studies without binding energy of phytoligand–target interaction, (iii) articles that exempted the binding sites of the phytoligand on the protein, (iv) studies that do not include molecular docking of phytocompounds, and (v) articles strictly involving virtual screening of conventional drugs. The authors independently searched, screened, and reviewed potentially pertinent publications. Duplicates were removed, disagreements were resolved when necessary, and this review represents a summary of data extracted from relevant studies. This PRISMA flowchart of this study is illustrated in Figure .
Results and discussions
A brief overview of coronavirus structure and proteome
The proteome of a typical coronavirus including SARS-CoV-2 consists of at least four structural proteins and several non-structural proteins (nsps). The structural proteins include the nucleocapsid (N), membrane (M), envelope (E), and spike (S) proteins (Figure (a, b)), all of which play crucial roles in the regulation of viral structure and functions (Schoeman and Fielding Citation2019). The N protein has two domains; N-terminal domain, and C-terminal domain, both of which are capable of binding and forming complexes with the viral RNA genome, but with disparate mechanisms. The N protein can also form complex with non-structural protein 3 (nsp3) and performs the packaging of the encapsidated genome into virions (Fehr and Perlman Citation2015; Chen et al. Citation2020). In addition, M glycoprotein, the most abundant coronaviral structural protein, has a small size ranging 25–30 kDa (Nal et al. Citation2005; Neuman et al. Citation2011). The M protein gives the virion its unique shape and is also involved in viral assembly (Arndt et al. Citation2010; Kuo et al. Citation2016). The E protein is very small with a size of ∼8–12 kDa. It is not only important for viral pathogenesis but also plays crucial roles in viral budding, assembly, and release (DeDiego et al. Citation2007; Nieto-Torres et al. Citation2014). Lastly, the S glycoprotein (Sgp) is quite big with a size of approximately 150 kDA. This glycoprotein consists of two subunits, S1 and S2 units. It is this surface protein that forms the spikes (petal-shaped surface projections), which are indispensable for viral attachment to host receptor and viral entry (Benaic et al. Citation2006; Chen et al. Citation2020). Although the receptor-binding domain (RBD) of SARS-CoV and SARS-CoV- 2 Sgps has about 72% amino acid sequence identity (Hemida and Ba Abduallah, Citation2020; Sun et al. Citation2020), SARS-CoV-2 Sgp has been reported to bind more strongly with the host receptor (ACE2) (Ali and Vijayan Citation2020). In addition, the SARS-CoV-2 Sgp also includes a unique region that can interact with and is activated by another host enzyme called furin (Coutard et al. Citation2020).
Figure 2. An illustration of the genomic organization and structure of SARS-CoV-2. (A) Genomic organization of SARS-CoV-2. The ORF1a and ORF1b of the viral RNA genome encode 16 non-structural proteins (nsp1–nsp16). Nsp3 and nsp5 encode PLpro and 3CLpro, respectively, which are essential for viral replication and pathogenesis. The genomic organisation of S glycoprotein showing its two subunits (S1 and S1) is depicted below the viral genome. Dotted lines within this structure indicate the S1/S2 cleavage site. Other important components of the S glycoprotein such as signal protein (SP), receptor-binding domain (RBD), fusion peptide (FP), heptad repeat (HR1 and HR2), transmembrane domain (TM), and cytoplasm domain (CP) are depicted. (B) Structure of SARS-CoV-2 showing its single-stranded positive-sense RNA genome and essential structural proteins.
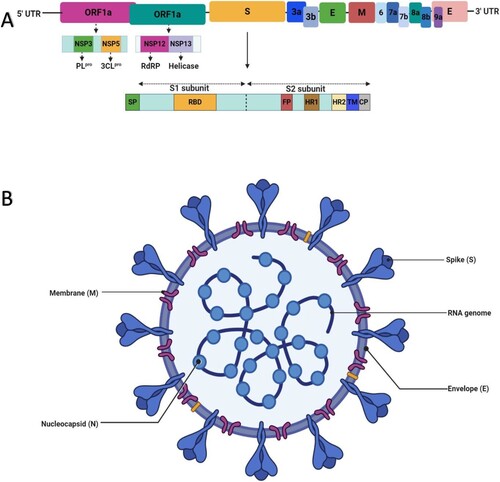
Furthermore, the ORFs – ORF1a and ORF1b – of the coronavirus genome encode 16 nsps, nsp1-11 and nsp1-16, that is subsequently cleaved into individual nsp (Ziebuhr et al. Citation2000; Fehr and Perlman Citation2015). The papain-like protease (PLpro) is encoded by nsp3. This protein catalyses the proteolytic processing of polyproteins (pp1a and pp1b) and plays a vital role in viral replication and host infection (Yu et al. Citation2020). It induces infection via its antagonistic effects on interferons (IFNs) and other immune cells. The nsp5 produces 3-chymotrypsin-like protease (3CLpro), also known as the main protease (Mpro). This protease is also essential for the cleavage of polyproteins and plays remarkable roles in viral replication and modulation of the immune system (Zhou et al. Citation2019; Chen et al. Citation2020). Interestingly, the 3CLpro of SARS-CoV-2 has a huge sequence resemblance (identity) of up to 96% with that of SARS-CoV (Fischer et al. Citation2020). In addition, the nsp12 and nsp13 of the coronavirus encode RdRp and helicase, respectively. Whilst RdRp catalyses the replication of viral RNA from the RNA templates, helicase also performs vital roles during viral replication and translation (Lung et al. Citation2020). Additionally, two other nsps – nsp14 and nsp15 encode exoribonuclease (ExoN) and endoribonuclease (EndoU), respectively (Figure a & b). Although ExoN acts as a proof-reader, EndoU cleaves viral polyuridine sequences, and this prevents the activation of host immune signals and viral evasion from immune cells (Graepel et al. Citation2017; Hackbart et al. Citation2020). A list of nsps with their functions are illustrated in Table .
Table 1. List of 16 coronaviral non-structural proteins with their functions.
Notably, whilst the dipeptidyl peptidase 4 (DPP4) represents the functional receptor of MERS-CoV, angiotensin-converting enzyme 2 (ACE2) is the host receptor that mediates and facilitates the entry of SARS-CoV and SARS-CoV-2 into the host cell, and this is achieved via the attachment and interaction of Sgp with this enzyme (Li et al. Citation2003; Raj et al. Citation2013; Li, Geng et al. Citation2020). Although other body organs such as kidneys, intestines, hearts, and brains contain ACE2, these receptors are found in great abundance in the lungs (alveoli) (Zhang, Xie et al. Citation2020), and this may partly explain why SARS-CoV-2 infection induces greater damage in the pulmonary system. Moreover, SARS-CoV-2 Sgp reportedly binds ACE2 more strongly than SARS-CoV (Coutard et al. Citation2020).
Virtual screening of phytocompounds as inhibitors of coronavirus-associated proteins
The traditional process of drug discovery is usually complex, strenuous, and quite inefficient. In addition, this process is often very expensive (more than $ 1 billion) and hugely time-consuming (up to 10 years) (Figure ) (Kapetanovic Citation2008; Al Qaraghuli et al. Citation2017). New approaches such as virtual screening with the use of computer docking models have been introduced. This process involves using computer-based techniques to screen and sieve drug candidates based on their desirability (activity, drug-like, and lead-like) and undesirability (toxicity and inactivity) as drugs, such that only suitable drug candidates proceed to subsequent animal testing and clinical trials (Kapetanovic Citation2008). This protocol does not only complement, facilitate, and accelerate the traditional drug discovery process, but also allows swift repurposing of already available drugs. In addition to its affordability, this technique can also be used for high-throughput screening of druggable targets and compounds (up to 10,000 compounds per day), to facilitate characterisation of drug side effects, reduce the need for animal models, and predict potential drug resistance (Davies and Davies Citation2010; Xia Citation2017). This method may be useful to guide the development of novel therapeutic agents for the present global pandemic. In fact, there is an unprecedent increase in the number of studies involving virtual screening of potential anti-COVID-19 agents ever since the structure of SARS-CoV-2 was characterised and solved in February 2020 (Fischer et al. Citation2020; Mohamed et al. Citation2020).
In silico modeling includes simulation, molecular docking, target point determination, and chemical stability studies for drug design. More specifically, the computational strategy is guided by the study and characterisation of protein–ligand interaction in a bid to identify potential drug candidates. (Mohammadi and Shaghaghi Citation2020). The strength of this interaction is elucidated by the calculated binding energy (kcal/mol). In other words, a more negative binding energy is an indicator of a stronger interaction and hence better activity. Notably, the remarkable anti-coronaviral potential of a number of phytocompounds has been highlighted using molecular docking technique (Chen and Du, Citation2020; Lung et al. Citation2020; Rane et al. Citation2020; Sampangi-Ramaiah et al. Citation2020; ul Qamar et al. Citation2020; Zhang, Wu et al. Citation2020). Table summarises molecules with considerable interaction with SARS-CoV-2 non-structural proteins, while Table depicts potential phytochemicals with activity against SARS-CoV-2 structural and host-associated proteins as predicted by computational studies.
Table 2. Compounds with potential against SARS-CoV-2 non-structural proteins evaluated through computational screening.
Table 3. Virtually screened phytocompounds with activity against SARS-CoV-2 structural proteins and host-associated proteins.
Inhibitors of 3CLpro
Since the main protease of SARS-CoV-2, 3CLpro (also referred to as Mpro) is essential for proteolytic processing of polypeptides, viral replication, and immune system modulation. Its inhibition will result in significant inhibition of viral replication and pathogenesis, and subsequent attenuation of infection. Consequently, studies have resorted to structural analyses to identify potential inhibitors of this target in a bid to guide the development of potential drug candidates for COVID-19. Notably, the main protease of SARS-CoV-2 has up to 96% sequence identity with that of SARS-CoV. Moreover, the catalytic dyad residues (His41 and Cys145) of SARS-CoV-2 3CLpro are identical to that of SARS-CoV 3CLpro (Yang et al. Citation2003). This has allowed many studies to swiftly dock molecules with reported anti-SARS-CoV 3CLpro potential.
Through a series of molecular docking, simulation, and toxicity assessment protocols, huge high-throughput computational screenings of 606 million compounds have highlighted the anti-SARS-CoV -2 3CLpro activity of 16 compounds including two phytocompounds, (-)-taxifolin and rhamnetin, with docking scores of −16.0 and −16.8 kcal/mol, respectively (Fischer et al. Citation2020). Notably, the latter is commercially available and can be procured as supplements at pharmacies. Additionally, of the 27 phytoligands docked against 3CLpro in a study by Sampangi-Ramaiah and co-workers (Citation2020), 12 (i.e. apigenin, glabridin, glycoumarin, glycyrrhizin, hederagenin, liquiritigenin, oleanolic acid, quercetin, rosmarinic acid, sageone, ursolic acid, and glucobrassicin) had considerable docking scores within the cut-off value (<−7 kcal/mol) implemented in the study. In addition, none of the tested compounds had a greater binding affinity than the reference antiviral drug (saquinavir) used in the study. However, most of these molecules interacted considerably with one of the catalytic residues (Cys145) of 3CLpro. Interestingly, many of these compounds are either phenolic or terpenoid (Sampangi-Ramaiah et al. Citation2020). Similarly, three terpenoids (taraxerol, friedelin, and stigmasterol) demonstrated better efficacy against SARS-CoV-2 3CLpro than ritonavir (positive control) (Kar et al. Citation2020).
Another large screening of 32,297 phytochemical TCM compounds revealed the anti-SARS-CoV-2 3CLpro activity of nine natural compounds including myricitrin, methyl rosmarinate, licoleafol, and amaranthin with very low binding energy (−29.57 to −18.14 kcal/mol). Interestingly, these phytocompounds exhibited better and stronger interaction with the conserved catalytic dyad residues (His41 and Cys145) of SARS-CoV-2 3CLpro than the conventional drugs (nelfinavir, colistin, and prulifloxacin) used in the study (ul Qamar et al. Citation2020). Considerable interaction of other compounds such as ursolic acid, oleanolic acid, andrographolide, neoandrographolide, glycyrrhizin, and 6-oxoisoiguesterin and a number of phenolic compounds, including apiin, baicalin, carvacrol, desmethoxycurcumin, epigallocatechin, glabridin, hesperidin, leucoefdin, puerarin, rhoifolin, myricetin, curcumin, calceolarioside B, and scutellarin with either of the catalytic residues of 3CLpro have also been predicted (Das et al. Citation2020; Enmozhi et al. Citation2020; Gyebi et al. Citation2020; Islam et al. Citation2020; Kumar, Choudhir et al. Citation2020; Murugan et al. Citation2020; Singh and Mishra Citation2020). The significance of such prediction is that these molecules will remain in interaction with the SARS-CoV-2 3CLpro since the conserved sites are hardly susceptible to mutation(s). As such, these compounds may offer a more useful guide in the development of COVID-19 drug candidates with profound efficacy. Additionally, mutations of SARS-CoV-2 3CLpro are often fatal to the virus (Silvestrini et al. Citation2021), a property that makes it a very attractive druggable target.
Furthermore, in another study involving docking of 110 plant-derived compounds against SARS-CoV-2 3CLpro, only 11 complied with the cut-off value (−6 kcal/mol) and most of these phytoligands were phenolic compounds (Hu et al. Citation2020). Phenolic compounds were predicted to interact better with this protease partly due to their abundant hydroxyl groups with higher affinities for the heteroatoms of the protease amino acids (Hu et al. Citation2020). In addition, rutin (vitamin P), a widely known antioxidant and an active constituent of many TCM (Houttuynia cordata, Forsythia suspensa, Morus alba, and Prunella vulgaris), had the highest binding energy and displayed considerable interaction with one of the conserved catalytic residues (Cys145) of 3CLpro. Furthermore, this molecule also interacts with and regulates host toll-like receptors (TLR2, TLR6, and TLR7). Its interaction and regulation of TLRs, in addition to its interaction with SARS-CoV-2 3CLpro, present this compound as a potential inhibitor of SARS-CoV-2 that deserves further exploration, since TLRs are involved in SARS-CoV-2-induced cytokine storm and inflammation, and blockage of its signals has been indicated to prevent this storm (Conti et al. Citation2020; Ling et al. Citation2020). Since the study by Hu and workers (Citation2020) was strictly computer-based, further in vitro and in vivo studies are required to validate the reported promising anti-COVID-19 potential of this phytocompound. In addition, the anti-inflammatory, antioxidant, anti-allergy, and antitumour properties of rutin have been reported by earlier studies, and these previous pharmacological evidences may further strengthen speculations of its anti-SARS-CoV-2 and immunomodulatory potential (Koval’skii et al. Citation2014).
Although a number of studies have predicted the binding efficiency of small molecules with both the monomeric and dimeric forms of 3CLpro, it is important to reiterate that, while the monomeric state of this protease also contains the relevant His41-Cys145 catalytic dyads, which makes it appropriate for in silico drug–target interaction studies, it is enzymatically inactive. As such, its dimeric form, the active form, would be more suitable for in vitro drug–target interaction studies (Silvestrini et al. Citation2021; Tekpinar and Yildirim, Citation2021).
Furthermore, the anti-coronaviral potential of 13 natural compounds from traditional Chinese herbs was demonstrated. A total of 115 compounds were identified through a literature search of natural compounds with anti-SARS-CoV and anti-MERS-CoV activities. These compounds were subjected to absorption, distribution, metabolism, and excretion (ADME) screening, with 13 compounds showing promise. This was followed by the molecular examination of the interaction of these 13 compounds with SARS-CoV-2 proteases. Of these compounds, six compounds, namely coumaroyl tyramine, cryptotanshinone, kaempferol, N-cis-feruloyltyramine, quercetin, and tanshinone IIa, interacted efficiently with both 3CLpro and PLpro. Further, moupinamide interacted exclusively with PLpro, while betulinic acid, desmethoxyreserpine, dihomo-γ-linolenic acid, lignan, and sugiol interacted with 3CLpro only (Zhang, Wu et al. Citation2020). Overall, while 11 (84.6%) of the phytoligands interacted with 3CLpro, only seven (53.8%) interacted with PLpro, and this might suggest 3CLpro as a more attractive druggable target. Moreover, four other studies have highlighted the molecular interaction of quercetin, a phenolic antioxidant found in many fruits, grains, and vegetables with 3CLpro (Figure a) (Das et al. Citation2020; Hu et al. Citation2020; Mani et al. Citation2020; Mishra et al. Citation2020). It is noteworthy that this compound also demonstrates proven anti-inflammatory, antioxidant, antithrombotic, antidiabetic, antiviral, and hepatoprotective properties (Zakaryan et al. Citation2017). These available pharmacological evidences present this molecule as an attractive potential anti-SARS-CoV-2 agent that requires further exploration.
Figure 4. Interaction of representatives of phytoligands, quercetin, and tryptanthrin with SARS-CoV-2 3CLpro and PLpro, respectively (Mani et al. Citation2020) (Reuse permission was obtained from Elsevier, Licence number: 5034780990710).
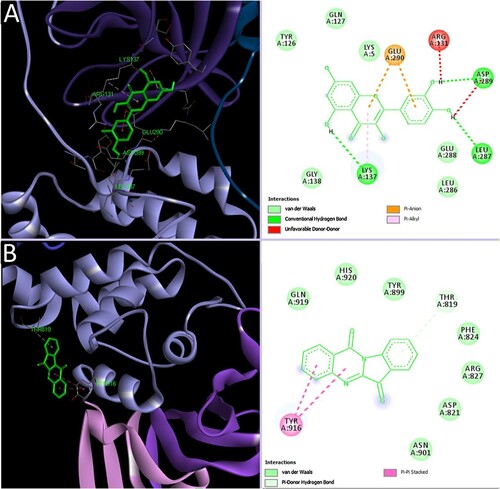
Inhibitors of PLpro
Similar to 3CLpro, PLpro plays important roles in the cleaving of polypeptides and viral replication. Additionally, it plays a role in the induction of viral infection. Inhibition of this essential protease is expected to result in the obstruction of viral replication and pathogenesis. Relative to 3CLpro, few studies have described the molecular interaction of PLpro with potential plant-derived inhibitors. The anti-SARS-CoV-2 PLpro potential of an alkaloid, tryptanthrin, found in Strobilanthes cusia, has been demonstrated (Figure ) (Mani et al. Citation2020). This compound has also been found to exhibit anti-HCoV-NL63, antimicrobial, antiparasitic, antioxidant, anticancer, antiallergic, and anti-inflammatory activities (Kaur et al. Citation2017; Tsai et al. Citation2020). A similar study has highlighted luteolin (a phenolic) as a potential inhibitor of SARS-CoV-2 PLpro (Yu et al. Citation2020).
Furthermore, considerable anti-SARS-CoV-2 PLpro potential of two terpenoids, platycodin D found in Platycodon grandifloras and sugetriol-3, 9-diacetate found in Cyperus rotundus; an alkaloid, phaitanthrin D found in Isatis indigotica; and seven other phenolic compounds have been reported (Wu et al. 2020).
Inhibitors of rdrp
Since RdRp is important for viral replication, inhibitors of this target are expected to prevent viral RNA synthesis and proliferation. Few reports are available on the molecular interaction of phytocompounds with SARS-CoV-2 RdRp. A study by Lung and co-workers (2020) involving 83 TCMs identified theaflavin, a phenolic compound found in black tea, as a potent inhibitor of SARS-CoV, SARS-CoV-2, and MERS-CoV, with the best binding affinity observed with SARS-CoV-2 RdRp. Prior pharmacological evidence has revealed the broad-spectrum antiviral activity of this molecule against hepatitis and influenza viruses (Yang et al. Citation2014; Chowdhury et al. Citation2018). The stronger binding affinity of three terpenoid compounds, taraxerol, friedelin, and stigmasterol with SARS-CoV-2 RdRp, compared to known inhibitors (remdesivir and favipiravir) of viral RdRp, has been observed (Kar et al. Citation2020; Sanders et al. Citation2020). Another interesting study highlighted the stronger interaction of luteolin, a phenolic compound found in honeysuckle (a main antiviral component of lianhuaqingwen) with SARS-CoV-2 RdRp, compared to remdesivir and ribavirin (Yu et al. Citation2020).
Inhibitors of structural proteins
As earlier stated, the structural proteins (N, S, E, M) of coronavirus are essential for its success as a virus and pathogen, as they play vital roles in viral entry, pathogenesis, assembly, budding, and release (Schoeman and Fielding Citation2019; Asghari et al. Citation2020; Naqvi et al. Citation2020). As such, these proteins have also attracted attention as potential druggable targets, although less compared to that received by the nsps. Of these structural proteins, overwhelming attention has been afforded to spike glycoprotein (Sgp), and this could be due to its critical role in viral attachment and subsequent entry into the host (Beniac et al. Citation2006; Chen et al. Citation2020). Inhibitors of Sgp will obstruct its attachment and interaction with the host receptor, and ultimately result in inhibition of SARS-CoV-2 entry.
A computational study by Rane and co-workers (2020) highlighted the anti-SARS-CoV Sgp potential of 10 phenolic compounds including curcumin, a widely known constituent of turmeric, and kaempferol, an antioxidant found in many vegetables and fruits. Notably, these compounds exhibited better affinity and stability with the Sgp than hydroxychloroquine (HCQ), suggesting that they may offer a more useful guide in the development of COVID-19 drug candidates. A similar study has demonstrated the Sgp-inhibiting potential of another phenolic compound, luteolin, found in honeysuckle (Yu et al. Citation2020). Interestingly, an earlier in vitro study has reported the potential activity of this compound against SARS-CoV Sgp (Yi et al. Citation2004). Moreover, the interaction of dihydrotanshinone, a diterpene found in Salvia miltiorrhiza with SARS-CoV Sgp, has also been reported (Zhang, Wu et al. Citation2020).
Additionally, a similar study by Kar and co-workers (2020) involving about 29 phytomolecules found in Clerodendrum spp. leaf has demonstrated the inhibitory potential of three terpenoids, taraxerol, friedelin, and stigmasterol against SARS-CoV-2 Sgp with docking scores < −7.0 kcal/mol. Notably, their binding affinities with Sgp were better relative to that of the tested antiviral drug, arbidol. More interestingly, taraxerol interacted with spike’s residues (Leu455, Glu484, and Tyr489) speculatively critical for binding host receptor (ACE2) (Ortega et al. Citation2020). In addition, 20 bioactive constituents of Glycyrrhiza glabra with prior pharmacological evidence which include antiviral, antimicrobial, anticancer, and immunomodulatory properties were docked against Sgp. Of these compounds, one terpenoid; glycyrrhizin, and three phenolics; liquiritin, glyasperin A, and isoliquiritinapioside were top-ranked inhibitors with binding energies of −9.2, −7.7, −7.9, and −7.4 kcal/mol, respectively (Sinha et al. Citation2020).
Furthermore, with the use of molecular docking and simulation, another study involving 70 compounds from Indian medicinal plant origin (Azadirachta indica) has identified 7-deacetyl-7- benzoylgedunin, 24-methylenecycloartanol, and cycloeucalenone as inhibitors of SARS-CoV 2 E protein. The same study identified beta-amyrin, phytosterol, and 24-methylenecycloartan-3-one as inhibitors of SARS-CoV 2 M protein. Moreover, nimbolin A and nimocin had remarkable interaction with both proteins (Borkotoky and Banerjee Citation2020). Notably, most of these compounds are members of the terpenoid class of secondary metabolites. High-affinity interaction of two terpenoids – excoecariatoxin found in Excoecaria agallocha and oleanolic acid found in Swertia kouitchensis – and 10 other phenolic compounds with spike protein has been demonstrated (Wu et al. 2020). However, of these compounds, only hesperidin predictably bound the interface between the spike and ACE2 receptor (Table ).
Inhibitors of host-associated protein
Unsurprisingly, ACE2 has received considerable attention as a potential druggable target in humans, and this is largely due to its integral role as the functional receptor that facilitates SARS-CoV-2 entry. Inhibitors of ACE2 are expected to obstruct the ACE2–spike protein interaction and ultimately prevent viral entry. Computational studies have predicted the interaction of several phytoligands with ACE2 (Table ). For example, a docking study by Wu and co-workers (2020) predicted the interaction of some compounds including phyllaemblicin G7 found in Phyllanthus emblica, hesperidin, α-glucosyl hesperidin, and neohesperidin found in Citrus spp., theaflavin 3,3′-di-O-gallate and theliokeep found in Camellia sinensis, platycodin D found in Platycodon grandifloras, excoecariatoxin found in Excoecaria agallocha, andrographolide and 14-deoxy-11,12- didehydroandrographolide found in Andrographis paniculata, rosmarinic acid found in Salvia verticillata, rutin found in Sophora japonica, and kouitchenoid B found in Swertia kouitchensis with ACE2. The remarkable interaction of a metal chelator, nicotianamine; a terpenoid, glycyrrhizin found in Glycyrrhiza radix; and three phenolics, hesperidin, an antitumour and antioxidant agent, baicalin and scutellarin, antioxidant molecules found in Chinese skullcap with ACE2 enzyme have been reported (Chen and Du Citation2020). Another study by Basu and co-workers (Citation2020) also demonstrated the molecular interaction of four phenolics compounds including hesperidin, emodin, anthraquinone derivative, and chrysin with the ACE2 receptor. Moreover, the interaction of dithymoquinone, an ingredient found in the widely known panacea, Nigella sativa (black seed) with SARS-CoV-2 S:ACE2 interface is noteworthy as it exhibited better binding affinity (<−8.0 kcal/mol) with the interface than HCQ (Ahmad et al. Citation2020). In addition, the interaction of other phenolic compounds such as piceatannol, pterostilbene, and resveratrol with S:ACE complex has also been predicted (Wahedi et al. Citation2020).
Conclusion and perspective
The present COVID-19 pandemic caused by SARS-CoV-2 has not only brought the world to its knees economically but has continued to take its toll on social and public health. The virus appears more infectious than SARS-CoV and MERS-CoV, and increasing evidence has shown that it can be transmitted by pre-symptomatic and asymptomatic patients (Xian et al. Citation2020). Currently, there are no specific antiviral drugs for its treatment. While vaccines have been rolled out, computational screenings are also being adopted to evaluate and validate the ability of phytoligands to interact with druggable targets essential for SARS-CoV-2 replication and pathogenesis. Amongst the predicted SARS-CoV-2 targets, the main protease (3CLpro) remains a very important druggable target. Fascinatingly, this target is also highly conserved and its mutation would have a fatal effect on the virus, a property that makes it a more significant target (Silvestrini et al. Citation2021). Several plant-derived compounds have been identified and may offer useful guides in the development of COVID-19 drug candidates with remarkable activity. Many of these compounds are either phenolics or terpenoids. Regarding the mechanisms of action of these phytoligands, a conceptual model (Figure ) could be proposed for typical inhibitors (such as quercetin, luteolin, and neoandrographolide) of SARS-CoV-2 druggable protein targets. Phenolic compounds; quercetin and luteolin, and a terpenoid; neoandrographolide potentially target and interact with SARS-CoV-2 druggable targets, such as 3CLpro, PLpro, and Sgp. Such interaction could result in the disruption of viral replication and pathogenesis. Similarly, neoandrographolide could predictably act against the host receptor of SARS-CoV-2, consequently preventing viral entry into the host cell.
Figure 5. The life cycle of SARS-CoV-2 and potential druggable targets of representative phytocompounds. Quercetin and luteolin predictably interact with 3CLpro, PLpro, and S glycoprotein, while neoandrographolide acts against 3CLpro, PLpro, host ACE2 receptor, RdRp and may also inhibit RNA replication.
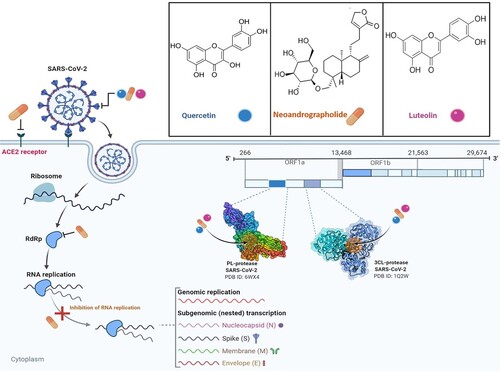
While computational approaches can offer leads for drug repurposing and novel discovery, the available data remain tentative and warrants further computational studies for validation, as well as developmental studies for clinical validation. It is hoped that this review will provide invaluable information that would inspire and guide the development of effective anti-SARS-CoV-2 agents from phytocompounds.
Acknowledgements
We express our sincere gratitude to those in the frontlines, in all realms of life, who are working tirelessly to restore global health and wellbeing. Figures 2, 3, and 5 were created with Biorender.com.
Disclosure statement
No potential conflict of interest was reported by the authors.
Data availability statement
Data sharing is not applicable to this article as no new data were created or analysed in this study.
References
- Abdelli I, Hassani F, Bekkel Brikci S, Ghalem S. 2020. In silico study the inhibition of angiotensin converting enzyme 2 receptor of COVID-19 by Ammoides verticillata components harvested from Western Algeria. J Biomol Struct Dyn. 1–14. DOI:10.1080/07391102.2020.1763199
- Adedeji AO, Lazarus H. 2016. Biochemical characterization of Middle East respiratory syndrome coronavirus helicase. mSphere. 1. DOI:10.1128/mSphere.00235-16
- Ahmad S, Abbasi HW, Shahid S, et al. 2020. Molecular docking, simulation and MM-PBSA studies of Nigella sativa compounds: a computational quest to identify potential natural antiviral for COVID-19 treatment. J Biomol Struct Dyn. 39, 12, 4225–4233. DOI:10.1080/07391102.2020.1775129
- Ahn DG, Choi JK, Taylor DR, Oh JW. 2012. Biochemical characterization of a recombinant SARS coronavirus nsp12 RNA-dependent RNA polymerase capable of copying viral RNA templates. Arch Virol. 157:2095–2104. DOI:10.1007/s00705-012-1404-x
- Ali A, Vijayan R. 2020. Dynamics of the ACE2–SARS-CoV-2/SARS-CoV spike protein interface reveal unique mechanisms. Sci Rep. 10:14214. DOI:10.1038/s41598-020-71188-3
- Al Qaraghuli MM, Alzahrani AR, Niwasabutra K, et al. 2017. Where traditional drug discovery meets modern technology in the quest for new drugs. Ann Pharmacol Pharm. 2:1061.
- Angelini MM, Akhlaghpour M, Neuman BW, Buchmeier MJ. 2013. Severe acute respiratory syndrome coronavirus nonstructural proteins 3, 4, and 6 induce double-membrane vesicles. mBio. 4. DOI:10.1128/mbio.00524-13
- Arndt AL, Larson BJ, Hogue BG. 2010. A conserved domain in the coronavirus membrane protein tail is important for virus assembly. J Virol. 84:11418–11428. DOI:10.1128/jvi.01131-10
- Asghari A, Naseri M, Safari H, et al. 2020. The novel insight of SARS-CoV-2 molecular biology and pathogenesis and therapeutic options. DNA Cell Biol. 39:1741–1753. DOI:10.1089/dna.2020.5703
- Ayeleso T, Matumba M, Mukwevho E. 2017. Oleanolic acid and its derivatives: biological activities and therapeutic potential in chronic diseases. Molecules. 22:1915. DOI:10.3390/molecules22111915
- Banerjee A, Kulcsar K, Misra V, et al. 2019. Bats and coronaviruses. Viruses. 11. 41, DOI:10.3390/v11010041
- Basu A, Sarkar A, Maulik U. 2020. Molecular docking study of potential phytochemicals and their effects on the complex of SARS-CoV2 spike protein and human ACE2. Sci Rep. 10. DOI:10.1038/s41598-020-74715-4
- Beachboard DC, Anderson-Daniels JM, Denison MR. 2015. Mutations across murine hepatitis virus nsp4 alter virus fitness and membrane modifications. J Virol. 89:2080–2089. DOI:10.1128/JVI.02776-14
- Beniac DR, Andonov A, Grudeski E, Booth TF. 2006. Architecture of the SARS coronavirus prefusion spike. Nat Struct Mol Biol. 13:751–752. DOI:10.1038/nsmb1123
- Borkotoky S, Banerjee M. 2020. A computational prediction of SARS-CoV-2 structural protein inhibitors from Azadirachta indica (Neem). J Biomol Struct Dyn. 39, 11, 4111–4121. DOI:10.1080/07391102.2020.1774419
- Bouvet M, Lugari A, Posthuma CC, et al. 2014. Coronavirus Nsp10, a critical co-factor for activation of multiple replicative enzymes. J Biol Chem. 289:25783–25796. DOI:10.1074/jbc.M114.577353.
- Chan JFW, Chan KH, Kao RY, et al. 2013. Broad-spectrum antivirals for the emerging Middle East respiratory syndrome coronavirus. J Infect. 67:606–616. DOI:10.1016/j.jinf.2013.09.029
- Chan JFW, Kok KH, Zhu Z, et al. 2020. Genomic characterization of the 2019 novel human-pathogenic coronavirus isolated from a patient with atypical pneumonia after visiting Wuhan. Emerg Microbes Infect. 9:221–236. DOI:10.1080/22221751.2020.1719902
- Chen H, Du Q. 2020. Potential natural compounds for preventing SARS-CoV-2 (2019-nCoV) infection. Preprints. DOI:10.20944/preprints202001.0358.v3
- Chen Y, Liu Q, Guo D. 2020. Emerging coronaviruses: genome structure, replication, and pathogenesis. J Med Virol. 92:418–423. DOI:10.1002/jmv.25681
- Chen Y, Su C, Ke M, et al. 2011. Biochemical and structural insights into the mechanisms of SARS coronavirus RNA ribose 2′-O-methylation by nsp16/nsp10 protein complex. PLoS Pathog. 7:e1002294. DOI:10.1371/journal.ppat.1002294
- Chowdhury P, Sahuc ME, Rouillé Y, et al. 2018. Theaflavins, polyphenols of black tea, inhibit entry of hepatitis C virus in cell culture. PLOS ONE. 13:e0198226.
- Chuang M-T, Ho F-M, Wu C-C, Zhuang S-Y, Lin S-Y, Suk F-M, Liang Y-C. 2011. 15,16-Dihydrotanshinone I, a Compound of Salvia miltiorrhiza Bunge, Induces Apoptosis through Inducing Endoplasmic Reticular Stress in Human Prostate Carcinoma Cells. Evidence-Based Complementary and Alternative Medicine. 2011:1–9.
- Conti P, Gallenga CE, Tetè G, et al. 2020. How to reduce the likelihood of coronavirus-19 (CoV-19 or SARS-CoV-2) infection and lung inflammation mediated by IL-1. J Biol Regul Homeost Agents. 34. DOI:10.23812/Editorial-Conti-2
- Cottam EM, Whelband MC, Wileman T. 2014. Coronavirus NSP6 restricts autophagosome expansion. Autophagy. 10:1426–1441. DOI:10.4161/auto.29309
- Coutard B, Valle C, de Lamballerie X, et al. 2020. The spike glycoprotein of the new coronavirus 2019-nCoV contains a furin-like cleavage site absent in CoV of the same clade. Antiviral Res. 176:104742. DOI:10.1016/j.antiviral.2020.104742
- Dai Y, Chen S-R, Chai L, et al. 2018. Overview of pharmacological activities of Andrographis paniculata and its major compound andrographolide. Crit Rev Food Sci Nutr. 59:S17–S29. DOI:10.1080/10408398.2018.1501657
- Das S, Sarmah S, Lyndem S, Singha Roy A. 2020. An investigation into the identification of potential inhibitors of SARS-CoV-2 main protease using molecular docking study. J Biomol Struct Dyn. 1–11. DOI:10.1080/07391102.2020.1763201
- Davies J, Davies D. 2010. Origins and evolution of antibiotic resistance. Microbiol Mol Biol Rev. 74:417–433. DOI:10.1128/mmbr.00016-10
- Decroly E, Debarnot C, Ferron F, et al. 2011. Crystal structure and functional analysis of the SARS-coronavirus RNA Cap 2′-O-methyltransferase nsp10/nsp16 complex. PLoS Pathog. 7:e1002059. DOI:10.1371/journal.ppat.1002059
- DeDiego ML, Álvarez E, Almazán F, et al. 2007. A severe acute respiratory syndrome coronavirus that lacks the E gene is attenuated in vitro and in vivo. J Virol. 81:1701–1713. DOI:10.1128/JVI.01467-06
- Dehelean CA, Şoica C, Ledeţi I, et al. 2012. Study of the betulin enriched birch bark extracts effects on human carcinoma cells and ear inflammation. Chem Cent J. 6. DOI:10.1186/1752-153x-6-137
- de Wit E, van Doremalen N, Falzarano D, Munster VJ. 2016. SARS and MERS: recent insights into emerging coronaviruses. Nat Rev Microbiol. 14:523–534. DOI:10.1038/nrmicro.2016.81
- Dong Z, Lu X, Tong X, et al. 2017. Forsythiae fructus: a review on its phytochemistry, quality control, pharmacology and pharmacokinetics. Molecules. 22:1466. DOI:10.3390/molecules22091466
- Egloff M-P, Ferron F, Campanacci V, et al. 2004. The severe acute respiratory syndrome-coronavirus replicative protein nsp9 is a single-stranded RNA-binding subunit unique in the RNA virus world. Proc Natl Acad Sci USA. 101:3792–3796. DOI:10.1073/pnas.0307877101
- Enmozhi SK, Raja K, Sebastine I, Joseph J. 2020. Andrographolide as a potential inhibitor of SARS-CoV-2 main protease: an in silico approach. J Biomol Struct Dyn. 1–7. DOI:10.1080/07391102.2020.1760136
- Fang SG, Shen H, Wang J, et al. 2008. Proteolytic processing of polyproteins 1a and 1ab between non-structural proteins 10 and 11/12 of coronavirus infectious bronchitis virus is dispensable for viral replication in cultured cells. Virology. 379:175–180. DOI:10.1016/j.virol.2008.06.038
- Fehr AR, Perlman S. 2015. Coronaviruses: an overview of their replication and pathogenesis. In: Maier H, Bickerton E, Britton P, editors. Coronaviruses. methods in molecular biology. New York, NY: Humana Press; p. 1–23.
- Fischer A, Sellner M, Neranjan S, et al. 2020. Potential inhibitors for novel coronavirus protease identified by virtual screening of 606 million compounds. Int J Mol Sci. 21:3626. DOI:10.3390/ijms21103626
- Gadlage MJ, Sparks JS, Beachboard DC, et al. 2010. Murine hepatitis virus nonstructural protein 4 regulates virus-induced membrane modifications and replication complex function. J Virol. 84:280–290. DOI:10.1128/JVI.01772-09
- Gallagher H, Williams JO, Ferekidis N, et al. 2019. Dihomo-γ-linolenic acid inhibits several key cellular processes associated with atherosclerosis. Biochim Biophys Acta (BBA) - Mol Basis Dis. 1865:2538–2550. DOI:10.1016/j.bbadis.2019.06.011
- Ganjhu RK, Mudgal PP, Maity H, et al. 2015. Herbal plants and plant preparations as remedial approach for viral diseases. VirusDisease. 26:225–236. DOI:10.1007/s13337-015-0276-6
- Gautret P, Lagier J-C, Parola P, et al. 2020. Hydroxychloroquine and azithromycin as a treatment of COVID-19: results of an open- label non-randomized clinical trial. Int J Antimicrob Agents. 56, 1, 105949. DOI:10.1016/j.ijantimicag.2020.105949
- Graepel KW, Lu X, Case JB, et al. 2017. Proofreading-deficient coronaviruses adapt for increased fitness over long-term passage without reversion of exoribonuclease-inactivating mutations. mBio. 8. DOI:10.1128/mBio.01503-17
- Graham RL, Donaldson EF, Baric RS. 2013. A decade after SARS: strategies for controlling emerging coronaviruses. Nat Rev Microbiol. 11:836–848. DOI:10.1038/nrmicro3143
- Gyebi GA, Ogunro OB, Adegunloye AP, et al. 2020. Potential inhibitors of coronavirus 3-chymotrypsin-like protease (3CLpro): an in silico screening of alkaloids and terpenoids from African medicinal plants. J Biomol Struct Dyn. 1–13. DOI:10.1080/07391102.2020.1764868
- Hackbart M, Deng X, Baker SC. 2020. Coronavirus endoribonuclease targets viral polyuridine sequences to evade activating host sensors. Proc Natl Acad Sci USA. 117:8094–8103. DOI:10.1073/pnas.1921485117
- Hao W, Wojdyla JA, Zhao R, et al. 2017. Crystal structure of Middle East respiratory syndrome coronavirus helicase. PLoS Pathog. 13:e1006474. DOI:10.1371/journal.ppat.1006474
- Harapan H, Itoh N, Yufika A, et al. 2020. Coronavirus disease 2019 (COVID-19): a literature review. J Infect Public Health. 13:667–673. DOI:10.1016/j.jiph.2020.03.019
- Harcourt BH, Jukneliene D, Kanjanahaluethai A, et al. 2004. Identification of severe acute respiratory syndrome coronavirus replicase products and characterization of papain-like protease activity. J Virol. 78:13600–13612. DOI:10.1128/jvi.78.24.13600-13612.2004
- Hemida MG, Ba Abduallah MM. 2020. The SARS-CoV-2 outbreak from a one health perspective. One Health. 10, 100127. DOI:10.1016/j.onehlt.2020.100127
- Hu X, Cai X, Song X, et al. 2020. Possible SARS-coronavirus 2 inhibitor revealed by simulated molecular docking to viral main protease and host toll-like receptor. Future Virol. 15, 6, 359–368. DOI:10.2217/fvl-2020-0099
- Imran M, Salehi B, Sharifi-Rad J, et al. 2019. Kaempferol: a key emphasis to its anticancer potential. Molecules. 24:2277. DOI:10.3390/molecules24122277
- Isaacson MK, Ploegh HL. 2009. Ubiquitination, ubiquitin-like modifiers, and deubiquitination in viral infection. Cell Host Microbe. 5:559–570. DOI:10.1016/j.chom.2009.05.012
- Islam R, Parves MR, Paul AS, et al. 2020. A molecular modeling approach to identify effective antiviral phytochemicals against the main protease of SARS-CoV-2. J Biomol Struct Dyn. 1–12. DOI:10.1080/07391102.2020.1761883
- Jamiu AT, Aruwa CE, Abdulakeem IA, et al. 2020. Phytotherapeutic evidence against coronaviruses and prospects for COVID-19. Pharmacognosy Journal. 12:1252–1267. DOI:10.5530/pj.2020.12.174
- Jesus JA, Lago JHG, Laurenti MD, et al. 2015. Antimicrobial activity of oleanolic and ursolic acids: an update. Evidence-Based Complementary Altern Med. 2015:1–14. DOI:10.1155/2015/620472
- Jia Z, Yan L, Ren Z, et al. 2019. Delicate structural coordination of the severe acute respiratory syndrome coronavirus Nsp13 upon ATP hydrolysis. Nucleic Acids Res. 47:6538–6550. DOI:10.1093/nar/gkz409
- Kapetanovic IM. 2008. Computer-aided drug discovery and development (CADDD): in silico-chemico-biological approach. Chem-Biol Interact. 171:165–176. DOI:10.1016/j.cbi.2006.12.006
- Kar P, Sharma NR, Singh B, et al. 2020. Natural compounds from Clerodendrum spp. as possible therapeutic candidates against SARS-CoV-2: An in silico investigation. J Biomol Struct Dyn. 39, 13, 4774–4785. DOI:10.1080/07391102.2020.1780947
- Kaur R, Manjal SK, Rawal RK, Kumar K. 2017. Recent synthetic and medicinal perspectives of tryptanthrin. Bioorg Med Chem. 25:4533–4552. DOI:10.1016/j.bmc.2017.07.003
- Kim S, Chen J, Cheng T, et al. 2019. Pubchem 2019 update: improved access to chemical data. Nucleic Acids Res. 47:D1102–D1109. DOI:10.1093/nar/gky1033
- Kim Y, Jedrzejczak R, Maltseva NI, et al. 2020. Crystal structure of Nsp15 endoribonuclease NendoU from SARS-CoV -2. Protein Sci. 29, 7, 1596–1605. DOI:10.1002/pro.3873
- Kirchdoerfer RN, Ward AB. 2019. Structure of the SARS-CoV nsp12 polymerase bound to nsp7 and nsp8 co-factors. Nat Commun. 10. DOI:10.1038/s41467-019-10280-3
- Koval’skii IV, Krasnyuk II, Krasnyuk II, et al. 2014. Mechanisms of rutin pharmacological action (review). Pharm Chem J. 48:73–76. DOI:10.1007/s11094-014-1050-6
- Kumar A, Choudhir G, Shukla SK, et al. 2020. Identification of phytochemical inhibitors against main protease of COVID-19 using molecular modeling approaches. J Biomol Struct Dyn. 39, 10, 3760–3770. DOI:10.1016/j.jpha.2020.03.001
- Kumar V, Dhanjal JK, Kaul SC, et al. 2020. Withanone and caffeic acid phenethyl ester are predicted to interact with main protease (Mpro) of SARS-CoV-2 and inhibit its activity. J Biomol Struct Dyn. 39, 11, 3842–3854. DOI:10.1016/j.jpha.2020.03.001
- Kuo L, Hurst-Hess KR, Koetzner CA, Masters PS. 2016. Analyses of coronavirus assembly Interactions with interspecies membrane and nucleocapsid protein chimeras. J Virol. 90:4357–4368. DOI:10.1128/jvi.03212-15
- Lei J, Kusov Y, Hilgenfeld R. 2018. Nsp3 of coronaviruses: structures and functions of a large multi-domain protein. Antiviral Res. 149:58–74. DOI:10.1016/j.antiviral.2017.11.001
- Li H, Liu SM, Yu XH, et al. 2020. Coronavirus disease 2019 (COVID-19): current status and future perspective. Int J Antimicrob Agents. 55, 5, 105951. DOI:10.1016/j.ijantimicag.2020.105951
- Li W, Moore MJ, Vasilieva N, et al. 2003. Angiotensin-converting enzyme 2 is a functional receptor for the SARS coronavirus. Nature. 426:450–454. DOI:10.1038/nature02145
- Li X, Geng M, Peng Y, et al. 2020. Molecular immune pathogenesis and diagnosis of COVID-19. J Pharm Anal. DOI:10.1016/j.jpha.2020.03.001
- Ling LJ, Lu Y, Zhang YY, et al. 2020. Flavonoids from Houttuynia cordata attenuate H1N1-induced acute lung injury in mice via inhibition of influenza virus and toll-like receptor signalling. Phytomedicine. 67:153150. DOI:10.1016/j.phymed.2019.153150
- Liu M, Yu Q, Yi Y, et al. 2020. Antiviral activities of Lonicera japonica thunb. components against grouper iridovirus in vitro and in vivo. Aquaculture. 519:734882. DOI:10.1016/j.aquaculture.2019.734882
- Lu R, Zhao X, Li J, et al. 2020. Genomic characterisation and epidemiology of 2019 novel coronavirus: implications for virus origins and receptor binding. The Lancet. 395, 10224, 565–574. DOI:10.2139/ssrn.3583748
- Lung J, Lin Y, Yang Y, et al. 2020. The potential chemical structure of anti-SARS-CoV-2 RNA-dependent RNA polymerase. J Med Virol. 92:693–697. DOI:10.1002/jmv.25761
- Luo Y, Wang CZ, Hesse-Fong J, et al. 2019. Application of Chinese medicine in acute and critical medical conditions. Am J Chin Med. 47:1223–1235. DOI:10.1142/s0192415(19500629
- Ma Y, Wu L, Shaw N, et al. 2015. Structural basis and functional analysis of the SARS coronavirus nsp14–nsp10 complex. Proc Natl Acad Sci USA. 112:9436–9441. DOI:10.1073/pnas.1508686112
- Mani JS, Johnson JB, Steel JC, et al. 2020. Natural product-derived phytochemicals as potential agents against coronaviruses: a review. Virus Res. 284:197989. DOI:10.1016/j.virusres.2020.197989
- Marinelli L, Fornasari E, Eusepi P, et al. 2019. Carvacrol prodrugs as novel antimicrobial agents. Eur J Med Chem. 178:515–529. DOI:10.1016/j.ejmech.2019.05.093
- Mishra RC, Kumari R, Yadav S, Yadav JP. 2020. Antiviral potential of phytoligands against chymotrypsin-like protease of COVID-19 virus using molecular docking studies: an optimistic approach. Research Square Prepri. DOI:10.2139/ssrn.3583748
- Miyaichi Y, Segawa A, Tomimori T. 2006. Studies on Nepalese crude drugs. XXIX. Chemical constituents of dronapuspi, the whole herb of Leucas cephalotes SPRENG. Chem Pharm Bull. 54:1370–1379. DOI:10.1248/cpb.54.1370
- Mohamed K, Yazdanpanah N, Saghazadeh A, Rezaei N. 2020. Computational drug discovery and repurposing for the treatment of COVID-19: a systematic review. SSRN Electronic Journal. DOI:10.2139/ssrn.3583748
- Mohammadi N, Shaghaghi N. 2020. Inhibitory effect of eight secondary metabolites from conventional medicinal plants on COVID_19 virus protease by molecular docking analysis. ChemRxiv. DOI:10.26434/chemrxiv.11987475.v1
- Moher D, Liberati A, Tetzlaff J, Altman DG. 2009. Preferred reporting items for systematic reviews and meta-analyses: the PRISMA statement. PLoS Med. 6:e1000097. DOI:10.1371/journal.pmed.1000097
- Mukherjee P, Shah F, Desai P, Avery M. 2011. Inhibitors of SARS-3CLpro: virtual screening, biological evaluation, and molecular dynamics simulation studies. J Chem Inf Model. 51:1376–1392. DOI:10.1021/ci1004916
- Murugan NA, Pandian CJ, Jeyakanthan J. 2020. Computational investigation on Andrographis paniculata phytochemicals to evaluate their potency against SARS-CoV-2 in comparison to known antiviral compounds in drug trials. J Biomol Struct Dyn. 39, 12, 4415–4426. DOI:10.1080/07391102.2020.1777901
- Nal B, Chan C, Kien F, et al. 2005. Differential maturation and subcellular localization of severe acute respiratory syndrome coronavirus surface proteins S, M and E. J Gen Virol. 86:1423–1434. DOI:10.1099/vir.0.80671-0
- Naqvi AAT, Fatima K, Mohammad T, et al. 2020. Insights into SARS-CoV-2 genome, structure, evolution, pathogenesis and therapies: structural genomics approach. Biochim Biophys Acta Mol Basis Dis. 1866:165878. DOI:10.1016/j.bbadis.2020.165878
- Neelam S, Gokara M, Sudhamalla B, et al. 2010. Interaction studies of coumaroyltyramine with human serum albumin and its biological importance. J Phys Chem B. 114:3005–3012. DOI:10.1021/jp910156k
- Neuman BW, Kiss G, Kunding AH, et al. 2011. A structural analysis of M protein in coronavirus assembly and morphology. J Struct Biol. 174:11–22. DOI:10.1016/j.jsb.2010.11.021
- Nieto-Torres JL, DeDiego ML, Verdiá-Báguena C, et al. 2014. Severe acute respiratory syndrome coronavirus envelope protein Ion channel activity promotes virus fitness and pathogenesis. PLoS Pathog. 10:e1004077. DOI:10.1371/journal.ppat.1004077
- Omrani AS, Saad MM, Baig K, et al. 2014. Ribavirin and interferon alfa-2a for severe Middle East respiratory syndrome coronavirus infection: a retrospective cohort study. Lancet Infect Dis. 14:1090–1095. DOI:10.1016/s1473-3099(14)70920-x
- Ortega JT, Serrano ML, Pujol FH, Rangel HR. 2020. Role of changes in SARS-CoV-2 spike protein in the interaction with the human ACE2 receptor: an in silico analysis. EXCLI J. 19:410–417. DOI:10.17179/excli2020-1167
- Raj VS, Mou H, Smits SL, et al. 2013. Dipeptidyl peptidase 4 is a functional receptor for the emerging human coronavirus-EMC. Nature. 495:251–254. DOI:10.1038/nature12005
- Rane JS, Chatterjee A, Kumar A, Ray S. 2020. Targeting SARS-CoV-2 spike protein of COVID-19 with naturally occurring phytochemicals: an in silco study for drug development. chemrxivorg. DOI:10.3389/fphar.2018.00481
- Saeed MEM, Mahmoud N, Sugimoto Y, et al. 2018. Betulinic acid exerts cytotoxic activity against multidrug-resistant tumor cells via targeting autocrine motility factor receptor (AMFR). Front Pharmacol. 9. DOI:10.3389/fphar.2018.00481
- Sampangi-Ramaiah HM, Vishwakarma R, Shaanker RU. 2020. Molecular docking analysis of selected natural products from plants for inhibition of SARS-CoV-2 main protease. Curr Sci. 118:1087–1092. DOI:10.18520/cs/v118/i7/1087-1092
- Sanders JM, Monogue ML, Jodlowski TZ, Cutrell JB. 2020. Pharmacologic treatments for coronavirus disease 2019 (COVID-19). JAMA.DOI:10.1001/jama.2020.6019
- Santos FA, Frota JT, Arruda BR, et al. 2012. Antihyperglycemic and hypolipidemic effects of α, β-amyrin, a triterpenoid mixture from Protium heptaphyllum in mice. Lipids Health Dis. 11. DOI:10.1186/1476-511x-11-98
- Schoeman D, Fielding BC. 2019. Coronavirus envelope protein: current knowledge. Virol J. 16. DOI:10.1186/s12985-019-1182-0
- Shang Q, Xu H, Huang L. 2012. Tanshinone IIA: a promising natural cardioprotective agent. Evidence-Based Complementary Altern Med. 2012:1–7. DOI:10.1155/2012/716459.
- Shereen MA, Khan S, Kazmi A, et al. 2020. COVID-19 infection: origin, transmission, and characteristics of human coronaviruses. J Adv Res. 24, 91–98. DOI:10.1016/j.jare.2020.03.005
- Shi P, Su Y, Li R, et al. 2019. PEDV nsp16 negatively regulates innate immunity to promote viral proliferation. Virus Res. 265:57–66. DOI:10.1016/j.virusres.2019.03.005
- Shode FO, Idowu ASK, Uhomoibhi OJ, Sabiu S. 2021. Repurposing drugs and identification of inhibitors of integral proteins (spike protein and main protease) of SARS-CoV-2. J Biomol Struct Dyn. 1–16. DOI:10.1080/07391102.2021.1886993
- Silvestrini L, Belhaj N, Comez L, Gerelli Y, Lauria A, Libera V, Mariani P, Marzullo P, Ortore MG, Piccionello A, et al. 2021. The dimer-monomer equilibrium of SARS-CoV-2 main protease is affected by small molecule inhibitors. Sci Rep. 11,. DOI:10.1038/s41598-021-88630-9
- Singh A, Mishra A. 2020. Leucoefdin a potential inhibitor against SARS CoV-2 mpro. J Biomol Struct Dyn. 39, 12, 4427–4432. DOI:10.1080/07391102.2020.1777903
- Sinha SK, Prasad SK, Islam MA, et al. 2020. Identification of bioactive compounds from Glycyrrhiza glabra as possible inhibitor of SARS-CoV-2 spike glycoprotein and non-structural protein-15: a pharmacoinformatics study. J Biomol Struct Dyn. 39, 13, 4686–4700. DOI:10.1080/07391102.2020.1779132
- Sun J, He WT, Wang L, et al. 2020. COVID-19: epidemiology, evolution, and cross-disciplinary perspectives. Trends Mol Med. 26, 5, 483–495. DOI:10.1016/j.molmed.2020.02.008
- Sutton G, Fry E, Carter L, et al. 2004. The nsp9 replicase protein of SARS-coronavirus, structure and functional insights. Structure. 12:341–353. DOI:10.1016/j.str.2004.01.016.
- Tanaka T, Kamitani W, Dediego M L, Enjuanes L, Matsuura Y. 2012. Severe Acute Respiratory Syndrome Coronavirus nsp1 Facilitates Efficient Propagation in Cells through a Specific Translational Shutoff of Host mRNA. Journal of Virology. 86(20):11128–11137.
- Tekpinar M, Yildirim A. 2021. Impact of dimerization and N3 binding on molecular dynamics of SARS-CoV and SARS-CoV-2 main proteases. J Biomol Struct Dyn. 1–12. DOI:10.1080/07391102.2021.1880481
- te Velthuis AJW, van den Worm SHE, Snijder EJ. 2012. The SARS-coronavirus nsp7+nsp8 complex is a unique multimeric RNA polymerase capable of both de novo initiation and primer extension. Nucleic Acids Res. 40:1737–1747. DOI:10.1093/nar/gkr893
- Thirupathaiah A, Rao GV, Takaishi Y. 2015. A lignan from the roots of Crossandra nilotica. Biomed Pharmacol J. 1:311–314.
- Tsai YC, Lee CL, Yen HR, et al. 2020. Antiviral action of tryptanthrin isolated from Strobilanthes cusia leaf against human coronavirus NL63. Biomolecules. 10:366. DOI:10.3390/biom10030366
- ul Qamar MT, Alqahtani SM, Alamri MA, Chen LL. 2020. Structural basis of SARS-CoV-2 3CLpro and anti-COVID-19 drug discovery from medicinal plants. J Pharm Anal. 10, 4, 313–319. DOI:10.1016/j.jpha.2020.03.009
- Umesh KD, Selvaraj C, et al. 2020. Identification of new anti-nCoV drug chemical compounds from Indian spices exploiting SARS-CoV-2 main protease as target. J Biomol Struct Dyn. 1–9. DOI:10.1080/07391102.2020.1763202
- van de Waterbeemd H, Gifford E. 2003. ADMET in silico modelling: towards prediction paradise? Nat Rev Drug Discovery. 2:192–204. DOI:10.1038/nrd1032
- Wahedi HM, Ahmad S, Abbasi SW. 2020. Stilbene-based natural compounds as promising drug candidates against COVID-19. J Biomol Struct Dyn. 1–10. DOI:10.1080/07391102.2020.1762743
- Wang M, Cao R, Zhang L, et al. 2020. Remdesivir and chloroquine effectively inhibit the recently emerged novel coronavirus (2019-nCoV) in vitro. Cell Res. 30, 3, 269–271. DOI:10.1038/s41422-020-0282-0
- Wang X, Lin H, Gu Y. 2012. Multiple roles of dihomo-γ-linolenic acid against proliferation diseases. Lipids Health Dis. 11:25. DOI:10.1186/1476-511x-11-25
- Wang Z, Chen X, Lu Y, et al. 2020. Clinical characteristics and therapeutic procedure for four cases with 2019 novel coronavirus pneumonia receiving combined Chinese and Western medicine treatment. Biosci Trends. 14, 1, 64–68. DOI:10.5582/bst.2020.01030
- Weathers PJ, Towler M, Hassanali A, et al. 2014. Dried-leaf artemisia annua: a practical malaria therapeutic for developing countries? World J Pharmacol. 3:39–55. DOI:10.5497/wjp.v3.i4.39
- Weaver BA. 2014. How taxol/paclitaxel kills cancer cells. Mol Biol Cell. 25:2677–2681. DOI:10.1091/mbc.e14-04-0916
- Wishart DS, Feunang YD, Marcu A, et al. 2018. HMDB 4.0: the human metabolome database for 2018. Nucleic Acids Res. 46:D608–D617. DOI:10.1093/nar/gkx1089
- World Health Organization (WHO). 2021. Coronavirus disease pandemic. [cited 2021 Mar 22].Available from: https://covid19.who.int/
- Wu C, Liu Y, Yang Y, Zhang P, Zhong W, Wang Y, Wang Q, Xu Y, Li M, Li X, et al. 2020. Analysis of therapeutic targets for SARS-CoV-2 and discovery of potential drugs by computational methods. Acta Pharmaceutica Sinica B. 10(5).
- Xia X. 2017. Bioinformatics and drug discovery. Curr Top Med Chem. 17:1709–1726. DOI:10.2174/1568026617666161116143440
- Xian Y, Zhang J, Bian Z, et al. 2020. Bioactive natural compounds against human coronaviruses: a review and perspective. Acta Pharm Sin B. 10, 7, 1163–1174. DOI:10.1016/j.apsb.2020.06.002
- Yang D, Leibowitz JL. 2015. The structure and functions of coronavirus genomic 3′ and 5′ ends. Virus Res. 206:120–133. DOI:10.1016/j.virusres.2015.02.025
- Yang H, Yang M, Ding Y, et al. 2003. The crystal structures of severe acute respiratory syndrome virus main protease and its complex with an inhibitor. Proc Natl Acad Sci USA. 100:13190–13195. DOI:10.1073/pnas.1835675100
- Yang ZF, Bai LP, Huang Wb, et al. 2014. Comparison of in vitro antiviral activity of tea polyphenols against influenza A and B viruses and structure–activity relationship analysis. Fitoterapia. 93:47–53. DOI:10.1016/j.fitote.2013.12.011
- Yi L, Li Z, Yuan K, et al. 2004. Small molecules blocking the entry of severe acute respiratory syndrome coronavirus into host cells. J Virol. 78:11334–11339. DOI:10.1128/JVI.78.20.11334-11339.2004
- Yoshida Y, Niki E. 2003. Antioxidant effects of phytosterol and its components. J Nutr Sci Vitaminol. 49:277–280. DOI:10.3177/jnsv.49.277
- Yu R, Chen L, Lan R, et al. 2020. Computational screening of antagonists against the SARS-CoV-2 (COVID-19) coronavirus by molecular docking. Int J Antimicrob Agents. 56 106012. DOI:10.1016/j.ijantimicag.2020.106012
- Zakaryan H, Arabyan E, Oo A, Zandi K. 2017. Flavonoids: promising natural compounds against viral infections. Arch Virol. 162:2539–2551. DOI:10.1007/s00705-017-3417-y
- Zeng J, Fan Y, Tan B, et al. 2018. Charactering the metabolism of cryptotanshinone by human P450 enzymes and uridine diphosphate glucuronosyltransferases in vitro. Acta Pharmacol Sin. 39:1393–1404. DOI:10.1038/aps.2017.144
- Zeng Z, Deng F, Shi K, et al. 2018. Dimerization of coronavirus nsp9 with diverse modes enhances its nucleic acid binding affinity. J Virol. 92. DOI:10.1128/JVI.00692-18
- Zhai Y, Sun F, Li X, et al. 2005. Insights into SARS-CoV transcription and replication from the structure of the nsp7–nsp8 hexadecamer. Nat Struct Mol Biol. 12:980–986. DOI:10.1038/nsmb999
- Zhang D, Wu K, Zhang X, et al. 2020. In silico screening of Chinese herbal medicines with the potential to directly inhibit 2019 novel coronavirus. J Integr Med. 18:152–158. DOI:10.1016/j.joim.2020.02.005
- Zhang J, Xie B, Hashimoto K. 2020. Current status of potential therapeutic candidates for the COVID-19 crisis. Brain Behav Immun. DOI:10.1038/s41586-020-2012-7
- Zhang X, Hu J, Chen Y. 2016. Betulinic acid and the pharmacological effects of tumor suppression. Mol Med Rep. 14:4489–4495. DOI:10.3892/mmr.2016.5792
- Zhou J, Fang L, Yang Z, et al. 2019. Identification of novel proteolytically inactive mutations in coronavirus 3C-like protease using a combined approach. FASEB J. 33:14575–14587. DOI:10.1096/fj.201901624rr
- Zhou P, Yang XL, Wang XG, et al. 2020. A pneumonia outbreak associated with a new coronavirus of probable bat origin. Nature. 579, 7798, 270–273. DOI:10.1038/s41586-020-2012-7
- Ziebuhr J, Gorbalenya AE, Snijder EJ. 2000. Virus-encoded proteinases and proteolytic processing in the nidovirales. J Gen Virol. 81:853–879. DOI:10.1099/0022-1317-81-4-853
- Zumla A, Hui DS, Perlman S. 2015. Middle East respiratory syndrome. The Lancet. 386:995–1007. DOI:10.1016/s0140-6736(15)60454-8