Abstract
The survival of the malaria parasite is highly dependent on withstanding physiological stresses, which are a proportional response to parasite invasion within the hostile human host environment. Plasmodium falciparum heat shock protein 70-1 (PfHSP70-1) plays a significant role through its network of interactions with the substrate scanners PfHSP40s, the functional maturation protein PfHSP90, the nucleotide exchange factor PfHSP110c/PfHSP70-z, apoptosis and parasite homeostasis agent PfHSP60, also the Clp machinery for the unfolding and degrading of misfolded proteins PfHSP100. These proteins work together to maintain the health and function of the parasite, but also possess individual functionalities. Here, we review the functional interplay between these heat shock proteins (HSPs), highlighting the central role of PfHSP70-1, its prospects in antimalarial drug discovery and possible implications in drug resistance.
Introduction
Malaria, caused by the protozoa Plasmodium falciparum (P. falciparum), is amongst the ‘big three’ neglected tropical diseases of the world together with tuberculosis and HIV/AIDS (Emerson et al. Citation2008; Bourzac Citation2014). Even with the onset of COVID-19, malaria is still amongst the top fatal infectious diseases in the world (World Health Organization Citation2021). Annually, malaria is responsible for over half a million deaths and 90% of these cases are from sub-Saharan Africa (Przyborski et al. Citation2015). The disease affects mostly children under the age of five years and pregnant women (Sannella et al. Citation2007; USAID Citation2018; Chakafana et al. Citation2019a).
P. falciparum is parasitic in nature, with a life cycle that exists in two different physiological environments, which are the vector Anopheles mosquito and the human host. It is an obligate intracellular parasite with an eroded genome because of depending on the life support of a host environment (Daniyan et al. Citation2019). In humans, the parasite invades the hepatocytes and erythrocytes, and adapts to their host environmental stressors such as temperature fluctuations which are responsible for febrile episodes in clinical malaria (Shonhai Citation2010; Przyborski et al. Citation2015; Njunge et al. Citation2015). This leads to heat shock stress that increases the propensity of proteins to unfold, thereby enhancing the formation of aggregates (Pavithra et al. Citation2007). As such, the parasite needs to employ a robust protein quality control mechanism for survival in the host environment. Thus, to establish a productive infection, the parasite needs to acclimatize against the unfamiliar harsh environment in which it is exposed. Among other survival strategies, the parasite deploys HSPs (Mabate et al. Citation2018) as part of its heat shock response. The heat shock response enables organisms to deal with physical stresses due to sudden changes in temperature, oxidative stresses, and exposure to toxic environments (Lilburn et al. Citation2014).
PfHSPs take up 2.8% of the parasite’s genome with an estimated 155 HSPs encoded by 95 genes (Lilburn et al. Citation2014; Yeo et al. Citation2015), constitute the cell’s protein-folding machinery and are upregulated during cellular stress. They also constitute about 5% of the population of approximately 450 exported parasitic proteins in the human red blood cells (Daniyan et al. Citation2019). The PfHSPs are a pervasive family of molecular chaperones that facilitate the survival and growth of the parasite in host cells by refolding misfolded proteins and unfolding preformed aggregates (Muralidharan et al. Citation2012; Zininga et al. Citation2015; Zininga et al. Citation2017a). In general, they form large oligomeric structures, and their functions are regulated by either co-chaperones or cofactors.
There are several families of HSPs classified according to their molecular weights, with the most common ones being PfHSP40, PfHSP60s, PfHSP70s, PfHSP90, PfHSP100s, PfHSP110s, and sPfHSPs (Shonhai Citation2010). The protein families are known to function independently as well as in partnerships. The functional valence of each PfHSP is complimented by their co-chaperones in that whilst the P. falciparum genes encode for only six PfHSP70s, there are at least 49 known PfHSP40s (Daniyan et al. Citation2019). The HSP40s are important substrate scanning recruiters for HSP70s for the purpose of refolding misfolded protein substrates (Pavithra et al. Citation2007; Daniyan et al. Citation2019).
HSP70s form one of the major chaperone families and are ATPases involved in protein folding, protein trafficking, and facilitation of the degradation of misfolded proteins through ubiquitination (Pesce et al. Citation2008; Bell et al. Citation2011). In short, they are protein-folding sentinels, and they have been cited to be essential and constitute potential drug targets across species (Pavithra et al. Citation2007; Pesce et al. Citation2008; Zininga and Shonhai Citation2014; Zininga et al. Citation2017a). To fulfil their protein-folding role, HSP70s interact with their substrates in an ATP-dependent function facilitated by nucleotide exchange factors (NEFs) (Njunge et al. Citation2015). They are mostly expressed in response to thermal stress across species (Lindquist and Craig Citation1988; Feder and Hofmann Citation1999). In P. falciparum, as well as in other organisms, these proteins function as protein-folding machinery, putative transporters, translocases, and secretory proteins associated with other PfHSP other than PfHSP70-1. In addition, PfHSP70s participate in translation involving multiple ribosomal proteins and a peptide release factor, regulation of transcription through interaction with several Zn-fingers and basic transcription factors to coordinate heat shock response (Lilburn et al. Citation2014), and the digestion of haemoglobin involving a cysteine protease falcipain-2, another promising antimalarial drug target (Deu Citation2017; Machin et al. Citation2019).
P. falciparum expresses six proteins of the HSP70s family which are PfHSP70-1 (PF3D7_0818900), PfHSP70-2 (PF3D7_0917900), PfHSP70-3 (PF3D7_113400), PfHSP70-x (PF3D7_0831700), PfHSP70-y (PF3D7_1344200), and PfHSP70-z (PF3D7_0708800) (Daniyan et al. Citation2019; Mabate et al. Citation2018; Zininga et al. Citation2017c). PfHsp70-1, -2, -3, and PfHsp70-x exhibit features of the canonical HSP70s, whilst PfHSP70-y and PfHSP70-z have features of the HSP110s (Lindquist and Craig Citation1988; Bell et al. Citation2011). Across species, HSP110s are regarded as NEFs of their canonical HSP70 counterparts (Zininga Citation2015; Zininga et al. Citation2015). NEFs stimulate the exchange of ADP for ATP which will be bound to other proteins, thus accelerating the release of ADP from HSP70 resulting in the rebinding of ATP, and the release of the substrate. In short, they regulate the lifespan of the HSP70–substrate complex (Zininga Citation2015; Liu et al. Citation2019). PfHSP70-1 has been studied extensively and shown to be at the centre of the protein-folding pathway. However, the chaperone does not function in isolation as it also possesses functional partners (Pavithra et al. Citation2007; Zininga et al. Citation2017c; Shonhai et al. Citation2007; Zininga et al. Citation2018; Acharya et al. Citation2007).
PfHSP70-x is closely related to PfHSP70-1 as indicated by a high percentage of sequence similarity (74%) thus both are highly conserved (Daniyan et al. Citation2019; Chakafana et al. Citation2019a). The protein is reported to be transported into the parasitophorous vacuole (PV) and host erythrocyte cytosol (Mabate Citation2017). The PV is an important pathway for the transportation of parasite-encoded proteins into the host erythrocyte. The localization of PfHSP70-x is highly debated, with many claims ending up being dismissed. Earlier studies suggested that the chaperone was localized in the Mauer’s cleft co-localizing with MAHRP1, a Mauer’s cleft marker, proposing the involvement of the protein in parasite protein sorting and export (Külzer et al. Citation2012; Daniyan et al. Citation2019). However, this assertion was dismissed as recent studies suggested that the chaperone is localized in distinct subcellular structures known as J Dots. It is known, however, that the chaperone is partially localized with PfEMP1 and is thought to be involved in the trafficking of PfEMP1 into the erythrocyte membrane (Külzer et al. Citation2012).
The pathogenicity of P. falciparum is partly due to parasite-induced host cell modifications initiated by a host of parasite’s exportome into the infected erythrocyte (Gitau Citation2014). This exportome transportation is facilitated by the Plasmodium export element (PEXEL) and host cell targeting signal (HT) (Botha et al. Citation2007; Schulze et al. Citation2015; Jha et al. Citation2017). The PfHSP70-x chaperone does not possess the PEXEL signal motif, but an N-terminal signal peptide of 24 amino acids directing it to the ER. The absence of the ER retention sequence suggests that the chaperone passes through the ER before being exported (Gitau Citation2014; Mabate et al. Citation2018). PfHSP70-x has been reported to have a role in regulating cytoadherence and folding proteins of parasitic origins in the infected red blood cell (RBC). The chaperone, however, has structural features uncommon in most cytosolic proteins, suggesting that it has distinct functions from the other proteins. HSP70-x homologues are unique only to P. falciparum and P. reichenowi (Chimpanzee malaria agent). Therefore, the chaperone is implicated in malaria pathogenicity. PfHSP70-x has been dismissed as being essential, but it is thought to be critically involved in the host immunity and cell invasion, as RBCs lacking the chaperone demonstrated reduced cytoadherence (Charnaud et al. Citation2017; Mabate et al. Citation2018).
Also known as the P. falciparum immunoglobulin binding protein (PfBiP) or the P. falciparum glucose-regulated protein 78 (PfGrp78), PfHSP70-2 is an endoplasmic reticulum (ER) protein that has not been extensively studied. It is most likely that the PfBiP is involved in protein secretion and degradation processes which are associated with the ER (Przyborski et al. Citation2015; Chen et al. Citation2018). Earlier studies also show that this ER protein also exhibits chaperone functions like PfHSP70-1 by protecting alcohol dehydrogenase and glutamate dehydrogenase from thermally induced stress leading to protein misfolding or unfolding (Ramya et al. Citation2006; Chen et al. Citation2018). In the ER, misfolded proteins beyond repair are removed by retrograde translocation using the ER-associated degradation mechanism (ERAD). The PfHSP70-2 chaperone is also thought to function in partnership with PfHSP40 and PfHSP70 proteins of the ER lumina (Botha et al. Citation2007; Pesce et al. Citation2010). PfHSP70-2 is an essential protein whose inhibition with small molecule inhibitors leads to the death of the parasite (Chen et al. Citation2018; Zininga and Shonhai Citation2019). However, the interaction of the small molecule cannot be entirely put on PfHSP70-2 alone, as there could be interaction with other essential parasitic proteins. Therefore, PfHSP70-2 could be a promising drug target through drug repurposing schemes (Zininga and Shonhai Citation2019).
The PfHSP110 are ‘adopted proteins’ of the PfHSP70 family
HSP110s are thought to be holdases with the capability to hold client proteins in a folding-worth manner under thermal stress. Thus, they subsequently titrate the proteins to their canonical partners for refolding (Zininga Citation2015). PfHSP70-z and PfHSP70-y are both proteins of the HSP110 family, besides having molecular weights of 108 and 100 kDa, respectively. They function primarily as NEFs for PfHSP70-1 and PfHSP70-2, respectively. Apart from that, as HSP110 proteins, they are predicted to have functions in suppressing protein aggregation of asparagine-rich repeats (Muralidharan et al. Citation2012; Zininga et al. Citation2017a; Daniyan et al. Citation2019; Zininga and Shonhai Citation2019).
The presence of the ER retention signal in PfHSP70-y suggests the protein is localized in the ER. As the chaperone also contains an apicoplast targeting signal, it was thought to be localized in the apicoplast (Shonhai et al. Citation2007; Gitau Citation2014). However, this assertion was dismissed and the apicoplast targeting peptide was shown to be overridden by the -KDEL sequence, thus making the chaperone a bona fide ER-luminal HSP. PfHSP70-y has been suggested to likely act as a NEF for PfHSP70-2 (Daniyan et al. Citation2019). There is little research on this protein, which may mean that it is not as essential as the other proteins in the family. However, it may be functionally essential as a NEF for PfHSP70-2 (Muralidharan et al. Citation2012; Liu and Houry Citation2014; Daniyan et al. Citation2019; Zininga and Shonhai Citation2019).
The general structure of HSP70s
HSP70s possess a highly conserved N-terminal housing the nucleotide (ATP/ADP) binding domain (NBD), a more varied C-terminal housing the peptide/substrate binding domain (SBD) connected via a highly conserved linker which facilitates allosteric communication between the domains (Figure ) (Zininga et al. Citation2017a; Mabate et al. Citation2018; Chakafana et al. Citation2019a; Chakafana et al. Citation2019b).
Figure 1. General structure of a canonical HSP70. The NBD, also known as the ATPase domain, is connected by a highly conserved linker to the SBD. The SBD, also known as the peptide binding domain, is characterized by an alpha-helical lid (red) which covers or exposes the binding site. Structure generated using PRIMO server (Hatherley et al. Citation2016).
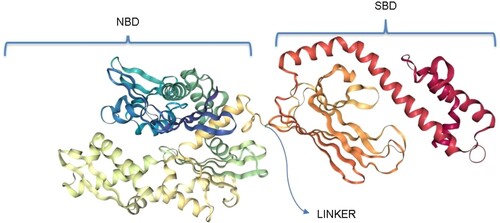
The distinct structural features of PfHSP70s
HSP70s are highly conserved proteins across species, and that poses a challenge of selectivity and specificity in drug design. However, despite their highly conserved features, PfHSP70s possess structural motifs distinctly unique to their functions coupled with their cooperation with co-chaperones which influences their specificity (Gong et al. Citation2018; Chakafana et al. Citation2019a; Chakafana et al. Citation2019b). Comparative studies carried on HSP70s from varied species highlight structural features that differentiate PfHSP70s from their human counterparts and other species (Shonhai Citation2010; Hatherley et al. Citation2014; Chakafana et al. Citation2019a).
The EEVD/N structural motif
Most of the HSP70s across species have the EEVD motif at the C-terminal, except for PfHSP70-x which has the EEVN motif instead (Figure ). The EEVD motif is thought to act as an anchor implicated in facilitating the binding of its functional partners and co-chaperones (Ramya et al. Citation2006; Gong et al. Citation2018). The motif was cited in earlier studies to be essential for ATPase activity thus the ability of the chaperone to interact with substrates, the deletion of which would lead to slowed activity or inactivity (Freeman et al. Citation1995; Misra and Ramachandran Citation2009). The EEVN motif in PfHSP70-x was shown to have the same functionality as the EEVD motif in facilitating the interaction of HSP70 with its functional partners such as heat shock organizing protein in vitro (HoP – which is the facilitator for HSP70-HSP90 interaction). The EEVN also contributes to the conformational integrity of PfHSP70-x (Mabate et al. Citation2018; Pesce et al. Citation2010; Gitau et al. Citation2012; Kravats et al. Citation2018).
Figure 2. The EEVD/N motif of the HSP70s across species reported with their corresponding Uniprot accession codes. PfHSP70-X has a variant motif, EEVN, from the common EEVD. PfHSP70-1 and PfHSP70-1 EEVD/N motif is preceded by a valine residue and not isoleucine like every other HSP70.
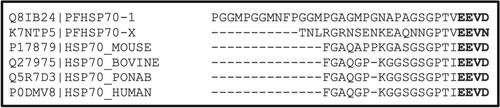
In almost every other species, the EEVD motif is preceded by an isoleucine residue and for the PfHSP70-x and PfHSP70-1, the EEVD/N motif is preceded by a valine residue. The two residues are closely identical in functionality; however, the alteration of these two residues affects the functionality of the motif (Yuan et al. Citation2010). The C-terminal TIEEVD sequence of Saccharomyces cerevisiae cytoplasmic HSP70 (Ssa1) was recently reported to play a role as a small ubiquitin-like modifiers (SUMOs)-interacting motif. SUMOs are essential regulators of a wide variety of cellular processes. The SUMOylation pathway is conserved in P. falciparum. As such, the TVEEVD motif present in the PfHSP70-1 suggests its potential involvement in the pathway. The TVEEVN variation for the PfHSP70-x counterpart may suggest the involvement of PfHSP70-x in SUMOylation as well. The essentiality of the SUMOylation pathway for normal cell function makes it a potential target for antiplasmodial small molecule inhibition (Reiter et al. Citation2013; Gong et al. Citation2018; Reiter and Matunis Citation2016). Therefore, the structural variations are crucial in structure-based design of selective inhibitors of PfHSP70-1.
The GGMP and EKEK motifs
PfHSP70-1 stands out due to the presence of glycine-glycine-methionine-proline (GGMP) tetrapeptide repeats, which also include a variation of GGMN twenty residues away from the TVEEVD sequence in the C-terminal (Daniyan et al. Citation2019; Makumire Citation2019). It is the only HSP70 with this repeat unit of all the HSP70 of both parasitic origin and human origin (Figure ). Makumire and colleagues postulate the involvement of the tetrapeptide motif in facilitating the interaction of PfHSP70-1 with the PfHop and that the motif may also have marginal effects on the structure (Makumire Citation2019). Gong et al. (Citation2018) reported the ability of a similar GGAP motif in Ssa1 to facilitate substrate binding through substrate recognition and the mediation of heat shock response. The GGMP variants were able to suppress heat-induced aggregation of MDH, in vitro, thereby suggesting that they bind or facilitate the binding of substrates and mediate heat shock response as is the case with the GGAP of Ssa1 (Makumire Citation2019). Studies by Gong et al. (Citation2018) also suggest combinatorial binding of substrates by the EEVD and GGAP motif. Since GGMP variants have the same effect on heat shock response and substrate recognition, we suggest that GGMP and EEVD may also be involved in combinatorial binding of some substrates in the same manner as GGAP. Makumire (Citation2019) showed that the GGMP motif enhances the chaperone function of DnaK which also explains why PfHSP70-1 has been found to be central in the chaperone network (Pavithra et al. Citation2007; Liu and Houry Citation2014).
Figure 3. The top box shows the GGMP motifs unique to PfHSP70-1. The bottom box shows the EKEK motif unique only to PfHSP70-z. The proteins were identified using Uniprot accession numbers.
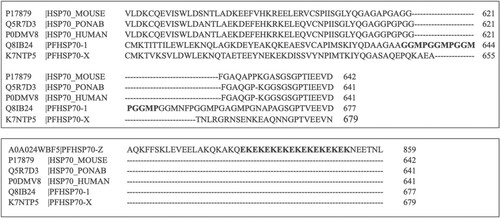
An 18 member EKEK repeat motif unique to PfHSP70-z was reported by Chakafana and colleagues (Figure ) (Chakafana et al. Citation2019a). The positioning of the 18mer repeat motif at the C-terminal suggests that it is part of the alpha-helical lid (a coil). As such, the motif may be responsible for the mechanical stability of the alpha-helical lid through intramolecular electrostatic interactions (Bornschlögl et al. Citation2009). The C-terminal sections of HSP70s have been reported to confer stability to the proteins (Misra and Ramachandran Citation2009; Mabate et al. Citation2018). This motif may also be responsible for electrostatic interactions with functional partners of this chaperone (Chakafana et al. Citation2019a).
This review focuses on the role of PfHSP70-1 in the parasite’s protein quality control mechanism, which is the protein-folding pathway, Figure . We highlight its functional partnerships with other chaperones with similar or complementary roles in protein quality control. The extensive research around the functional role of PfHSP70-1 in the survival of the parasite justified the approach taken in this review (Pesce et al. Citation2008; Bell et al. Citation2011; Gitau et al. Citation2012; Njunge et al. Citation2015; Zininga et al. Citation2017a, Citation2017c). The highlight on the functional players in the PfHSP70-1 will aid in the understanding of the protein-folding machinery towards drug discovery.
PfHSP70-1 interacts with other PfHSP70 family chaperones
PfHSP70-1 is upregulated by thermal stress and is thus implicated in parasite cytoprotection. The localization of PfHSP70-1 in the cytosol and nucleus indicates its function as an agent of proteostasis within the subcellular compartments. Earlier studies show that PfHSP70-1 is also involved in reversing oxidative stress in yeast cells with compromised HSP70 functionality (Bell et al. Citation2011; Gitau et al. Citation2012), improving the expression and purification of recombinant P. falciparum GTP cyclohydrolase I (PfGCHI) produced in E. coli (Shonhai et al. Citation2005; Stephens et al. Citation2011; Gitau et al. Citation2012). Some researchers suggest that PfHSP70-1 is involved in either protein aggregation or the folding of PfGCHI (Stephens et al. Citation2011). PfHSP70-1 is also thought to act in concert with a highly expressed Knob-associated histidine-rich protein (PF3D7_0202000) on the RBC cytosolic membrane side during the operation of the Plasmodium translocon of exported proteins (PTEX) translocon assisting the exported protein across the membrane (Deu Citation2017; Machin et al. Citation2019). The PTEX is partially composed of PfHSP101 which is known to associate functionally with HSP70s in other systems (De Koning-Ward et al. Citation2009; Przyborski et al. Citation2015).
PfHSP70-z is co-localized with PfHSP70-1 in the cytosol. PfHSP70-z is as thermostable as PfHSP70-1 and is thought to have evolved to protect the parasite against the harmful effects of protein aggregation during febrile episodes (Zininga et al. Citation2015). However, it has been shown that PfHSP70-z is more resilient to heat stress than PfHSP70-1, but both proteins are capable of suppressing heat-induced protein aggregation as is the case with malate dehydrogenase (MDH) (Zininga et al. Citation2017b). Therefore, these proteins have been shown to work together to maintain proteostasis in the parasite cytoplasm (Przyborski et al. Citation2015). PfHSP70-z works in concert with PfHSP70-1 by acting as the nucleotide exchange factor of the latter (Zininga et al. Citation2015). Zininga et al. (Citation2015) also postulated that PfHSP70-z has an independent chaperone function apart from its association with PfHSP70-1. The P. falciparum proteome is rich in asparagine-rich proteins which are highly prone to aggregation and thus moderation is required (Przyborski et al. Citation2015). PfHSP70-z has been shown to be essential for parasite viability and the suppression of aggregation of asparagine-rich proteins more effectively than any other chaperone (Muralidharan et al. Citation2012). PfHSP70-1 and PfHSP70-z have been reported to be essential for the survival and growth of the parasite, respectively (Zininga et al. Citation2015). Inhibitors of both chaperones are known to inhibit the ATPase and chaperone functions by abrogating their associations with functional partners (Zininga et al. Citation2017c).
The PfHSP40–PfHSP70 relationship
HSP40s are defined by the presence of a highly conserved J-domain which is characteristically located at the N-terminal of the proteins (Cheetham and Caplan Citation1998; Qiu et al. Citation2006). The J-domain is said to also facilitate cross talks with HSP70 (Gisselberg et al. Citation2017) adding to its involvement in stimulating its ATPase activity (Figure ) (Tsai and Douglas Citation1996; Cajo et al. Citation2006; Qiu et al. Citation2006; Chiang et al. Citation2009). The J-domain interacts with both functional domains of the HSP70 chaperone and is closely packed against the linker section of the chaperone (Kityk et al. Citation2018).
Figure 5. The structure of the complex of HSP70 bound to J-domain (HSP40) with all the domains shown as labelled. The J-domain facilitates cross talks with HSP70 through binding to both the SBD and NBD while closely packed against the linker of the HSP70 (PDB ID:5NRO) (Kityk et al. Citation2018).
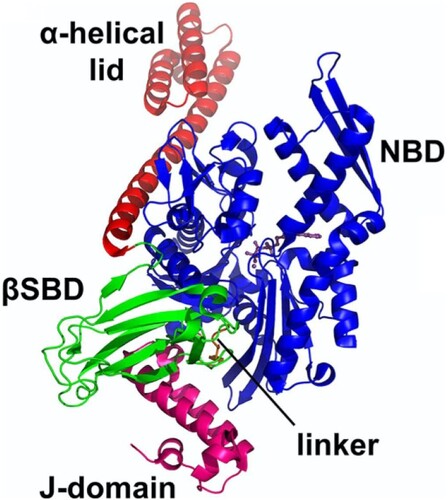
The HSP40s consist of the N-terminal (which houses the J-domain) which binds the NBD of HSP70 and the C-terminal which binds the client substrates (Figures and ). They consist of four helices (I–IV) containing a highly conserved histidine-proline-aspartate (HPD tripeptide) motif (Cheetham and Caplan Citation1998; Cajo et al. Citation2006; Qiu et al. Citation2006). The HPD motif has been cited to be crucial to the co-chaperone functionality with HSP70 and these interactions have been reported to be highly conserved (Kityk et al. Citation2018). HSP40s are categorized into four types based upon the organization of the domains (Cheetham and Caplan Citation1998; Qiu et al. Citation2006; Botha et al. Citation2007; Njunge et al. Citation2013); Figures and .
Figure 6. The general structure of the J-domain of an HSP40 (pdb: hdj1): Four helices numbered I–IV. The chain terminals are labelled C and N, respectively. The highly conserved His31 (red), Pro32 (blue), Asp33 (yellow) shown as HPD between Helices II–III.
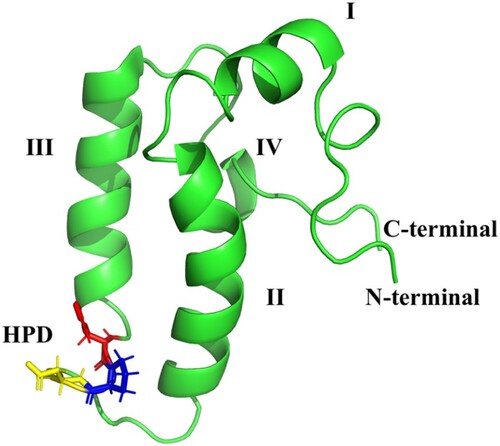
Figure 7. The classification of HSP40s according to domain organization and suggested functions of the domains. Type I possesses the J-domain, the glycine/phenylalanine (G/F) rich region, and zinc binding cysteine rich region which consists of CXXCXGXG repeats and a less conserved carboxyl-terminal domain (120–170 amino acids). Type II possesses the J-domain, the G/F rich region, and the carboxyl-terminal. Type I and II are believed to have similar functions and to bind to non-native substrates. Type III only possesses the J-domain and does not bind to non-native substrates. It has been suggested that it cannot function as an individual chaperone, and the J-domain may be located anywhere in the molecule. Type IV, first identified by Botha et al. (Citation2007) possesses the J-domain with variations in the HPD motif.
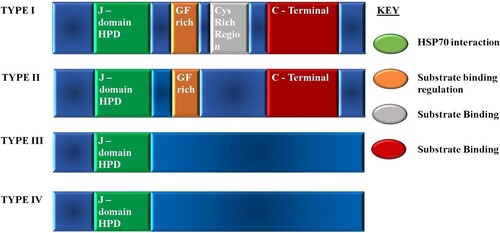
Type I and II of the HSP40s are known to be functioning as substrate ‘scanners’ for HSP70 by first binding to the substrates and delivering them to HSP70. Type III HSP40s were reported to recruit HSP70 to specific intracellular locations to fold their substrates (Rug and Maier Citation2011). The function of type IV is poorly known, but members are thought to be involved in cell remodelling (Pesce and Blatch Citation2014).
The P. falciparum genome encodes 49 PfHSP40s for a minimal six PfHSP70s which covers the functional valence of the HSP70s (Hygon Citation2016; Daniyan et al. Citation2019). Of the 49 HSP40s, 36 are type I–III, and the additional 13 falls in the type IV HSP40s with a modified J-domain HPD motif (Njunge et al. Citation2013). Several of the HSP40s are predicted to be exported to the host cell as they contain the export element, PEXEL, and the vacuolar translocon signal, and most of them are thought to be type IV HSP40s (Botha et al. Citation2007; Njunge et al. Citation2013; Pesce and Blatch Citation2014). The exported HSP40s are thought to be directly involved in host cell remodelling (Pesce and Blatch Citation2014).
P. falciparum contains two type I HSP40s that have been shown to interact with the cytosolic PfHSP70-1 and these are (i) PFD0462w and (ii) PF14_0359. Both J-proteins do not have the export element. PFD0462w was shown to stimulate the protein-folding activity of PfHSP70-1, in vitro (Rug and Maier Citation2011), while PF14_0359 co-localizes with PfHSP70-1 and is similarly heat stress-induced (Botha et al. Citation2010; Pesce and Blatch Citation2014). PF14_0359 stimulated ATP hydrolysis of both parasitic HSP70 and human HSP70, in vitro, and these were differentially inhibited by small molecule modulators (Rug and Maier Citation2011). The in vitro ATPase and aggregation assays showed functional interaction between PF14_0359 and PfHSP70-1 (Botha et al. Citation2010). PfHSP70-1 was also reported to interact with some non-exported type II HSP40s and believed to have a cytoprotection function during cellular stress (Rug and Maier Citation2011). Again, PfHSP70-1 is shown to interact with two type II HSP40s. PFL0565w (Pfj4), by co-localization and co-immunoprecipitation, is reported to have the same heat induction patterns as PfHSP70-1 (Pesce et al. Citation2008). PFB0595w was predicted to interact with PfHSP70-1 earlier (Pavithra et al. Citation2007), and it was shown experimentally that the HSP40 stimulates ATP hydrolysis activity, in vitro (Njunge et al. Citation2015).
Whole-genome sequencing studies on clinical parasite samples, collected during the emergence of artemisinin-resistance (AR) in Thailand, showed a nonsynonymous mutation in HSP40. This may provide a background understanding of AR mutations (Cerqueira et al. Citation2017). As such, HSP40 may be involved in AR. Zhang et al. (Citation2021) report a high correlation between the P. falciparum transcriptional response to thermal stress and artemisinin. Evidence shows that targeting apicoplast functionality, prenylation or heat shock pathways is a promising start as adjunctive therapies to reverse AR or artemisinin susceptibility (Zhang et al. Citation2021; Mathews et al. Citation2021). Researchers also suggest that a combination therapy targeting both protein prenylation and HSPs directly is potentially parasiticidal.
The functional interactions which exist between PfHSP40 and PfHSP70-1 represent potential drug target pathways. As such, extensive studies have been done in this line (Botha et al. Citation2007; Pesce et al. Citation2008; Botha et al. Citation2010; Njunge et al. Citation2013; Njunge et al. Citation2015). As PfHSP40 and PfHSP70-1 functional partnership is essential in the parasite’s survival, combination therapy which targets individual functionality has the potential to be parasiticidal.
The PfHSP90–PfHSP70-1 relationship
The PfHSP90 family is a highly conserved family of ATP-dependent molecular chaperones that facilitate protein folding, namely cytosolic inducible PfHSP90α (PF3D7_0708400) and constructive PfHSP90β (PF3D7_1443900), endoplasmic reticulum Grp94 (PF3D7_1222300), apicoplast and mitochondrial tumour necrosis factor type 1 receptor-associated protein Trap1 (PF3D7_1118200), involved in diverse cellular processes (Shahinas et al. Citation2013a; Seraphim T et al. Citation2019).
PfHSP90 exists in a homodimer configuration with three domains, the nucleotide-binding domain located at the N-terminus, a middle domain supporting ATP-turnover, and a SBD at the C-terminal capable of dimerization, possessing an EEVD motif which is characteristic of the cytosolic chaperone (Acharya et al. Citation2007; Corbett and Berger Citation2010; Shahinas et al. Citation2013a; Chakafana et al. Citation2019a). It is known to be expressed in all three stages of the parasite life cycle, the ring stage, the trophozoite stage, and the schizont stage (Gitau et al. Citation2012; Chua et al. Citation2014; Seraphim T et al. Citation2019). Dobson et al. (Citation2001) report that PfHSP90 is anti-genetically similar to the mammalian HSP90. It is the most abundant chaperone in cells, playing several essential roles in protein folding and cell protein functionality. Kumar et al. (Citation2003) inform us of the PfHSP90 high similarity to its orthologs in other species, in sequence and biochemical activities that are relevant to its chaperone function. HSP90 is responsible for protein homeostasis and vital cellular process maintenance for the survival of the parasite. PfHSP90α plays a major role in protein translation and chromatin remodelling of the parasite through its interactions with chromatin assembly factor 1 p55 subunit (PFTANZ_05295), nucleosome assembly protein (PF3D7_0919000), and histone deacetylase 1 (PF3D7_0925700) (Pavithra et al. Citation2007; Priya et al. Citation2015; Chakafana et al. Citation2019a).
PfHSP70-1 (PF3D7_0818900) refolds misfolded proteins which are then handed over to PfHSP90 for functional maturation through complete folding, a vital function of the PfHSP90, aided by its capability to recognize target proteins in their near-native state (Kravats et al. Citation2018; Daniyan et al. Citation2019). The interplay between these two proteins becomes more apparent in the parasite’s plight towards drug resistance through rapid protein refolding.
PfHSP90α is upregulated in response to physiological stress and antimalarial drug-induced stress. Drug resistance arises as a result of interactions between HSP90 and the Cg4 (an HSP110 chaperone), the chloroquine resistance-associated protein (Daniyan et al. Pavithra et al. Citation2007; Shahinas et al. Citation2013a; Shahinas et al. Citation2013b). Posfai et al. (Citation2018) highlight a decrease in drug potency at the late liver stages of infection attributed to higher levels of the 90 kDa HSP, at a time when the parasite requires rapid folding of newly synthesized proteins. The connected interplay between PfHSP90α and PfHSP70-1 is linked by the PfHOP as the adapter drives this process. Richter and Buchner (Citation2001) highlight the signal transduction functionality of the HSP90 through facilitating folding of molecules involved in signal transduction, steroid hormone receptors, members of the Src-kinase family of non-receptor tyrosine kinases, cell cycle kinase Cdk4, Raf, and other serine/threonine kinases (Utpal and Soundara Raghavan Citation2004; Wayne et al. Citation2011; Chua et al. Citation2014).
The interaction between PfHSP70-1 and PfHSP90 is regulated by an adapter protein, the Pf HSP70-HSP90 organizing protein (PfHOP). PfHOP facilitates the transfer of proteins from PfHSP70 to PfHSP90 for completion of the folding process (functional maturation) (Gitau et al. Citation2012; Chua et al. Citation2014). Studies have shown that the functional interaction between the two chaperones is essential for the survival of the parasite, as such the complex functionality presents a potential drug target pathway. Mainly, the inhibition of PfHSP70-1 abrogated the interaction with the co-chaperone PfHOP and in turn the interaction with PfHSP90 (Gitau et al. Citation2012; Chua et al. Citation2014; Zininga et al. Citation2017a; Daniyan et al. Citation2019).
The PfHsp70-1 functional interaction network summary
See Figure .
PfHSP70-1 as an antimalarial drug target
This review shows the essentiality of PfHSP70-1 as an independent chaperone and also in its functional partnerships with other HSPs of P. falciparum. There are many inhibition studies that have been done targeting PfHSP70-1. These include ATPase activity assays, aggregation functional assays and allosteric modulation. HSP70 molecules are allosteric; as such their modulation by small molecule inhibitors impacts their global conformation and influences their interactions with functional partners. The allosteric nature of these molecules is aided by the linker facilitating signal transmission between the SBD and NBD. When the NBD is in an ATP-bound state, the SBD exhibits low affinity for the substrates, this leads to the release of bound substrate. A higher substrate affinity is observed for ADP-bound state (Zininga et al. Citation2017a, Citation2017b, Citation2017c). To date, researchers have demonstrated that PfHSP70-1 is capable of binding small molecules and inhibit its function as an ATPase.
Fewell et al. (Citation2004) reported the activity of 15-deoxyspergualin (15-DSG) and its analogues in the modulation of ATPase activity of HSP70-1 and HSP90. 15-DSG (Figure ) is an analogue of spergualins isolated from the culture broth of Bacillus laterosporus, and is known to inhibit the growth of P. falciparum in vitro and in vivo (Ramya et al. Citation2006). In their work, they discovered that 15-DSG does not inhibit the chaperone function of HSP70-1, but instead the compound stimulates the ATPase activity of the chaperone by binding to the EEVD motif of the C-terminal of the chaperone. This meant that 15-DSG and its analogues only have an effect on chaperones that have the EEVD motif (Fewell et al. Citation2004). This theory was supported by Ramya et al. (Citation2006, Citation2007) as other members of the HSP family without the EEVD motif were unaffected by the presence of 15-DSG.
Figure 9. 15-DSG stalled protein synthesis in P. falciparum through PfHsp70 sequestration and phosphorylation of eukaryotic initiation factor (eIF2a) (Ramya et al. Citation2006; Fewell et al. Citation2004; Ramya et al. Citation2007).
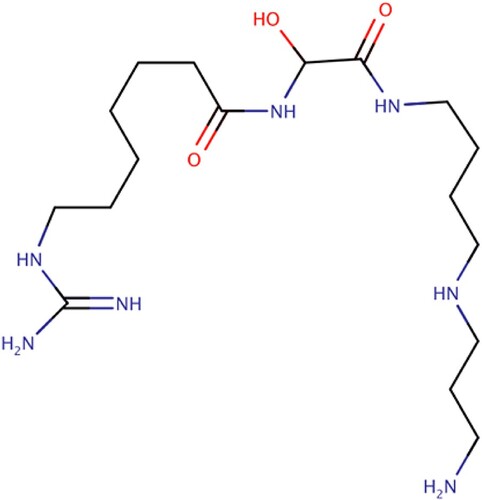
Fewell et al. (Citation2004) still identified two membrane permeable compounds, MAL3-39 and MAL3-101, that modulated an HSP40–HSP70-1 dependent process. In vitro, these compounds inhibited posttranslational translocation, which is a known HSP40-HSP70-1 partnership phenomenon. Inhibiting this partnership may have downstream effects on the processes facilitated by the two co-chaperones. One such presumptive effect would be the reduced ability of J-domain containing proteins to stimulate HSP70-1 ATPase activity. However, the downstream effects of this phenomenon have been poorly researched.
The mechanism by which 15-DSG (Shonhai Citation2010) inhibits the growth of P. falciparum is not well known; presumably this is by depleting P. falciparum’s polyamine reserves. However, similar molecules to 15-DSG have been studied for the modulation of HSP70-1 ATPase activity in P. falciparum. Ramya et al. (Citation2007) revealed that 15-DSG stalled protein synthesis in P. falciparum through PfHSP70-1 sequestration and subsequent phosphorylation of eukaryotic initiation factor, eIF2α. 15-DSG selectively enhances the ATPase activity of chaperones containing the C-terminal EEVD motif, which might be the basis of a specific modulation of the function of proteins that bind the EEVD motif of HSPs (Ramya et al. Citation2006). We know that interaction of the 15-DSG with the C-terminal of PfHsp70-1 EEVD motif is responsible for the reported autophosphorylation of eIF2α kinase and subsequent protein synthesis (Fewell et al. Citation2004; Ramya et al. Citation2006, Citation2007).
Another assumption of 15-DSG’s inhibitory activity in P. falciparum is the inhibition of trafficking of NEAT proteins by interfering with HSP70-1 binding to the transit peptide in NEAT proteins. This phenomenon prevents the transit peptide from remaining in the unfolded conformation that is essential to apicoplast targeting (Ramya et al. Citation2007). However, most of the work on 15-DSG is rather presumptive. Shonhai et al. (Citation2010) suggested the possibility of the influence 15-DSG could have on the interaction of HSP40 through both ATPase and C-terminal domains of PfHSP70-1 through their earlier observation of ionic contacts that could bring the NBD and SBD in close spatial orientation. Researchers suggest the C-terminal to bring stability to the chaperone against thermal stress (Misra and Ramachandran Citation2009; Shonhai Citation2010). Hence, 15-DSG may compromise the stability of PfHSP70-1 against heat stress by binding to the EEVD motif. Downstream, this phenomenon may affect the functional capability of PfHSP70-1 (Shonhai Citation2010). Hence, research into the apicoplast MEP pathway related to PfHSP40 may provide more knowledge towards the effect of 15-DSG on the PfHSP40-PfHSP70-1 partnership (Fewell et al. Citation2004; Mathews et al. Citation2021).
A variety of active compounds have been studied over the years, from synthetic compounds to natural extracts and isolations. All the compounds that have been reported to inhibit the (Utpal and Soundara Raghavan Citation2004) activity of PfHSP70-1 include ATP mimetics (adenosine analogues), spergualins, pyrimidinones, fatty acids, and peptides (Chiang et al. Citation2009; Cockburn et al. Citation2011; Cockburn et al. Citation2014; Evans et al. Citation2015; Zininga et al. Citation2017c, Citation2017b; Kayamba et al. Citation2021; Salomane et al. Citation2021).
Chiang et al. (Citation2009) identified synthetic pyrimidinones with in vitro activity against the ATPase activity of PfHSP70-1 at higher millimolar concentrations of the inhibitory agents (300 mM). The IC50 values required to inhibit parasite growth by these compounds were in the nanomolar–micromolar range of 30 nM–300 µM. A key occurrence in the cellular function of Hsp70 is its interaction with Hsp40 partners containing the J-domain. The discrepancy between in vivo and in vitro assays may be attributed to the compound’s ability to affect the J-domain-stimulated ATPase activity of the Hsp70. This has been seen in a study where a compromised J-domain containing co-chaperone (Hsp40) had limited ability in enhancing ATP hydrolysis (Rodina et al. Citation2007). Interestingly, these compounds were able to selectively inhibit PfHSP70-1 over its human HSP70-1 counterpart. Molecular modifications of these compounds may improve their activities against PfHSP70-1. Shonhai et al. (Citation2010) suggested that modification of the compounds from their pyrimidinone backbone which is thought to be essential to their activities may actually improve the activities of the compounds. The variations of these compounds mainly come from this pyrimidinone backbone, as shown in Figure below. This is evidenced by the varied effects of the compounds on the activity of PfHSP70-1 compared with its human HSP70-1 and yeast, Ssa1, counterparts (Chiang et al. Citation2009). These compounds’ activities seemed to stimulate the basal activity of the chaperone even in the absence of a J-domain which may affect its role under physiologically stressful conditions (Chiang et al. Citation2009; Shonhai Citation2010).
Figure 10. Pyrimidinones with in vitro activity against the ATPase activity of PfHsp70-1. These pyrimidinones are known to enhance the ATP hydrolysis of HSP70 as such have potential to affect the ability of PfHsp70-1 to act as a chaperone (Chiang et al. Citation2009).
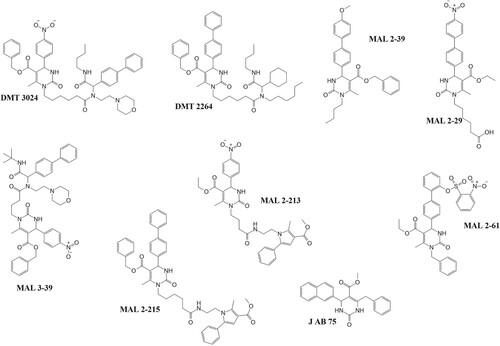
Three marine compounds of the malonganenone family extracted from Leptogorgia gilchristi selectively inhibit the ATPase activity of both cytosolic and RBC exported PfHSP70-x (Cockburn et al. Citation2011). These novel marine compounds (Figure below) were found to have cytotoxic effects towards a number of oesophageal cancer cells (Keyzers et al. Citation2006). This study serves as a starting point for synthetic derivatives of the novel malonganenone backbone. Cockburn et al. (Citation2011) also identified lapachol (Figure ), a 1,4 – naphthoquinone extract, which selectively inhibited the aforementioned HSP70s. Lapachol is a naturally occurring naphthoquinone found in the heart-wood of various trees of the bigonaceous family, and they possess both anticancer and antiplasmodial activity (Bonifazi et al. Citation2010). Both classes of compounds share structural similarities to purine analogues (ATP-like) and known inhibitors of PfHSP70 and PfHSP90 like geldanamycin (quinone analogues) (Cockburn et al. Citation2011). Both groups of compounds were shown to inhibit the protein aggregation activity of PfHSP70-1 with at least 90% malate dehydrogenase (MDH) aggregation. Malonganenone A and C also showed high inhibition of parasite growth comparable to the work by Chiang et al. (Citation2009; Cockburn et al. Citation2011). Aggregation assays in their study also required high concentrations of inhibitory agents in the micromolar range (100–300 µM) (Cockburn et al. Citation2011).
Figure 11. Chemical structures of PfHsp70-1 inhibitors: These compounds (with the exception of SANCs) inhibit the growth of the parasite and also suppress PfHsp70-1’s aggregation suppression function. SANC compounds induce allosteric conformational dynamics affecting nucleotide/substrate release events (Cockburn et al. Citation2011; Zininga et al. Citation2017a, Citation2017b, Citation2017c; Amusengeri et al. Citation2019).
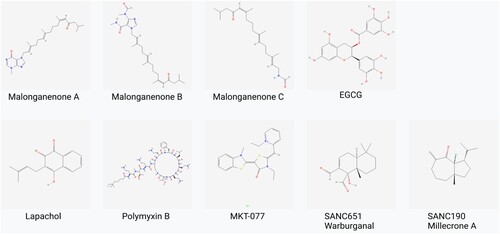
The Zininga et al. (Citation2017b, Citation2017c) group also identified two compounds that bind to the ATPase domain of PfHSP70-1 in the micromolar range. These two compounds include a cyclic peptide, polymyxin B (PMB) and a green tea flavonoid, (-) – epigallocatechin-3-O-gallate (EGCG) (Figure ) which also inhibit both ATPase activity and chaperone function of PfHSP70-1 and PfHSP70-z. Micromolar ranges for the inhibition of ATPase activity on both chaperones are indicative of the druggability potential of PfHSP70-1 and possible functionality interchange between PfHSP70-1 and PfHSP70-z (Zininga et al. Citation2017a, Citation2017c; Kayamba et al. Citation2021; Tavella et al. Citation2021).
Amusengeri et al. (Citation2019) devised an in-silico method to identify small molecule allosteric modulators of PfHSP70-1 and PfHSP70-x. In their work, they identified two natural products, SANC190 and SANC651, which displayed considerable allosteric regulation characteristics (Pavithra et al. Citation2007). The binding of these two compounds modulates the conformational dynamics of the two chaperones and subsequently regulates succeeding ADP/substrate release events (Amusengeri et al. Citation2019). There is a need for biochemical evaluation of these compounds to validate their allosteric modulation activities.
Conclusion
HSPs, as molecular chaperones, ensure the functional and structural integrity of other proteins. This is important for the parasite’s genome as it seeks to adapt to the physiologically stressful human host genome. The parasite encodes for a variety of these HSPs, but some have been reported to be highly essential that their functional distraction leads to lethal effects for the parasite. However, the functional interplay which exists in these proteins means that the functional valence of one protein may be covered by another (compensatory up-regulation). Vital and crucial functions of HSPs, including protein folding, refolding, translocation, trafficking, and degradation are carried out as corporate activities which is an essential factor in drug resistance. PfHSP70-1, is an essential chaperone with many functional partners in the chaperone network. We demonstrate here the functional centrality of the chaperone compared to other HSPs involved in the chaperone network. We show here that the chaperone has crucial partners to its functional valence, some HSP40s, PfHSP90α, and PfHSP70-z (PfHSP110c), and these have direct relations to the functionality of the chaperone. Inhibiting the function of PfHSP70-1 has been reported to be lethal to the parasite, as such it is a promising drug target in antimalarial drug discovery research. Research should look at modifying existing promising antiHSP70 compounds to improve the activities reported from earlier work, and to synthesize derivatives of the novel groups of compounds reported herein. Understanding the functional partners and interplay of these proteins is important towards efforts of modifying the most promising antiplasmodial compounds targeting these HSPs. It may also be possible to target the pathway to curb the possible emergence of drug resistance from compensatory up-regulation of functional partners.
Disclosure statement
No potential conflict of interest was reported by the author(s).
Data availability statement
Data sharing not applicable to this article as no datasets were generated or analysed during the current study.
Additional information
Funding
References
- Acharya P, Kumar R, Tatu U. 2007. Chaperoning a cellular upheaval in malaria: heat shock proteins in Plasmodium falciparum. Mol Biochem Parasitol. 153(2):85–94.
- Amusengeri A, Astl L, Lobb K, Verkhivker GM, Bishop ÖT. 2019. Establishing computational approaches towards identifying malarial allosteric modulators: a case study of plasmodium falciparum hsp70s. Int J Mol Sci. 20(22):5574.
- Bell SL, Chiang AN, Brodsky JL. 2011. Expression of a malarial Hsp70 improves defects in chaperone-dependent activities in ssa1 mutant yeast. PLoS One. 6(5):1–10.
- Bonifazi EL, Ríos-Luci C, León LG, Burton G, Padrón JM, Misico RI. 2010. Antiproliferative activity of synthetic naphthoquinones related to lapachol. First synthesis of 5-hydroxylapachol. Bioorganic Med Chem. 18(7):2621–2630. http://doi.org/10.1016/j.bmc.2010.02.032.
- Bornschlögl T, Woehlke G, Rief M. 2009. Single molecule mechanics of the kinesin neck. Proc Natl Acad Sci U S A. 106(17):6992–6997.
- Botha M, Chiang AN, Needham PB, Stephens LL, Hoppe HC, Külzer S, Przyborski JM, Lingelbach K, Wipf P, Brodsky JL, et al. 2010. Plasmodium falciparum encodes a single cytosolic type i Hsp40 that functionally interacts with Hsp70 and is upregulated by heat shock. Cell Stress Chaperones. 16(4):389–401.
- Botha M, Pesce ER, Blatch GL. 2007. The Hsp40 proteins of Plasmodium falciparum and other apicomplexa: regulating chaperone power in the parasite and the host. Int J Biochem Cell Biol. 39(10):1781–1803.
- Bourzac K. 2014. Infectious disease: beating the big three. Nature. 507:S4–S7.
- Cajo GC, Horne BE, Kelley WL, Schwager F, Georgopoulos C, Genevaux P. 2006. The role of the DIF motif of the DnaJ (Hsp40) co-chaperone in the regulation of the DnaK (Hsp70) chaperone cycle. J Biol Chem. 281(18):12436–12444.
- Cerqueira GC, Cheeseman IH, Schaffner SF, Nair S, McDew-White M, Phyo AP, Ashley EA, Melnikov A, Rogov P, Birren BW, et al. 2017. Longitudinal genomic surveillance of Plasmodium falciparum malaria parasites reveals complex genomic architecture of emerging artemisinin resistance. Genome Biol. 18(1):1–13.
- Chakafana G, Zininga T, Shonhai A. 2019a. Comparative structure-function features of Hsp70s of Plasmodium falciparum and human origins. Biophys Rev. 11(4):591–602.
- Chakafana G, Zininga T, Shonhai A. 2019b. The link that binds: the linker of Hsp70 as a helm of the protein’s function. Biomolecules. 9(10):543.
- Charnaud SC, Dixon MWA, Nie CQ, Chappell L, Sanders PR, Nebl T, Hanssen E, Berriman M, Chan J-A, Blanch AJ, et al. 2017. The exported chaperone Hsp70-x supports virulence functions for Plasmodium falciparum blood stage parasites. PLoS One. 12(7):e0181656.
- Cheetham ME, Caplan AJ. 1998. Structure, function and evolution of DnaJ: conservation and adaptation of chaperone function. Cell Stress and Chaperones. 3:28–36.
- Chen Y, Murillo-Solano C, Kirkpatrick MG, Antoshchenko T, Park HW, Pizarro JC. 2018. Repurposing drugs to target the malaria parasite unfolding protein response. Sci Rep. 8(1):1–12. http://doi.org/10.1038/s41598-018-28608-2.
- Chiang AN, Valderramos J-C, Balachandran R, Chovatiya RJ, Mead BP, Schneider C, Bell SL, Klein MG, Huryn DM, Chen XS, et al. 2009 Feb 15. Select pyrimidinones inhibit the propagation of the malarial parasite Plasmodium Falciparum. Bioorg Med Chem. 17(4):1527–1533. http://www.ncbi.nlm.nih.gov/pubmed/19195901.
- Chua C-SS, Low H, Sim T-SS. 2014 Aug. Co-chaperones of Hsp90 in plasmodium falciparum and their concerted roles in cellular regulation. Parasitology. 141(9):1177–1191.
- Cockburn IL, Boshoff A, Pesce ER, Blatch GL. 2014. Selective modulation of plasmodial Hsp70s by small molecules with antimalarial activity. Biol Chem. 395(11):1353–1362.
- Cockburn IL, Pesce ER, Pryzborski JM, Davies-Coleman MT, Clark PGK, Keyzers RA, Stephens LL, Blatch GL. 2011. Screening for small molecule modulators of Hsp70 chaperone activity using protein aggregation suppression assays: inhibition of the plasmodial chaperone PfHsp70-1. Biol Chem. 392(5):431–438.
- Corbett KD, Berger JM. 2010. Structure of the ATP-binding domain of Plasmodium falciparum Hsp90. Proteins Struct Funct Bioinforma. 78(13):2738–2744.
- Daniyan MO, Przyborski JM, Shonhai A. 2019. Partners in mischief: functional networks of heat shock proteins of Plasmodium falciparum and their influence on parasite virulence. Biomolecules. MDPI AG. 9:295.
- De Koning-Ward TF, Gilson PR, Boddey JA, Rug M, Smith BJ, Papenfuss AT, Sanders PR, Lundie RJ, Maier AG, Cowman AF, Crabb BS. 2009. A newly discovered protein export machine in malaria parasites. Nature. 459(7249):945–949.
- Deu E. 2017. Proteases as antimalarial targets: strategies for genetic, chemical, and therapeutic validation. FEBS J. 284(16):2604–2628.
- Dobson S, Kar B, Kumar R, Adams B, Barik S. 2001. A novel tetratricopeptide repeat (TPR) containing PP5 serine/threonine protein phosphatase in the malaria parasite, Plasmodium falciparum. BMC Microbiol. 1:1–10.
- Emerson PM, Ngondi J, Biru E, Graves PM, Ejigsemahu Y, Gebre T, Endeshaw T, Genet A, Mosher AW, Zerihun M, et al. 2008. Integrating an NTD with one of ‘the big three’ combined malaria and trachoma survery in Amhara region of Ethiopia. PLoS Negl Trop Dis. 2(3):e197.
- Evans LE, Cheeseman MD, Yahya N, Jones K. 2015. Investigating apoptozole as a chemical probe for HSP70 inhibition. PLoS One. 10(10):1–13.
- Feder ME, Hofmann GE. 1999. Heat-shock proteins, molecular chaperones, and the stress response: evolutionary and ecological physiology. Annu Rev Physiol. 61:243–282.
- Fewell SW, Smith CM, Lyon MA, Dumitrescu TP, Wipf P, Day BW, Brodsky JL. 2004. Small molecule modulators of endogenous and co-chaperone-stimulated Hsp70 ATPase activity. J Biol Chem. 279(49):51131–51140. http://doi.org/10.1074/jbc.M404857200.
- Freeman BC, Myers MP, Schumacher R, Morimoto RI. 1995. Identification of a regulatory motif in Hsp70 that affects ATPase activity, substrate binding and interaction with HDJ-1. EMBO J. 14(10):2281–2292.
- Gisselberg JE, Zhang L, Elias JE, Yeh E. 2017. The prenylated proteome of Plasmodium falciparum reveals pathogen-specific prenylation activity and drug mechanism-of-action. Mol Cell Proteomics. 16(4):S54–S64. http://doi.org/10.1074/mcp.M116.064550.
- Gitau GW. 2014. Characterisation of the plasmodium falciparum Hsp70- A Thesis submitted in fulfillment of the requirements for the degree. University of Zululand.
- Gitau GW, Mandal P, Blatch GL, Przyborski J, Shonhai A. 2012. Characterisation of the Plasmodium falciparum Hsp70-Hsp90 organising protein (PfHop). Cell Stress Chaperones. 17(2):191–202.
- Gong W, Hu W, Xu L, Wu H, Wu S, Zhang H, Wang J, Jones GW, Perrett S. 2018. The C-terminal GGAP motif of Hsp70 mediates substrate recognition and stress response in yeast. J Biol Chem. 293(46):17663–17675.
- Hatherley R, Blatch GL, Bishop ÖT. 2014. Plasmodium falciparum Hsp70-x: a heat shock protein at the host-parasite interface. J Biomol Struct Dyn. 32(11):1766–1779. http://doi.org/10.1080/07391102.2013.834849.
- Hatherley R, Brown DK, Glenister M, Bishop ÖT. 2016. PRIMO: An interactive homology modeling pipeline. PLoS One. 11(11):1–20.
- Hygon M. 2016. Characterization of PFF1010c, a type IV Plasmodium falciparum heat shock protein 40. Thohoyandou: University of Venda.
- Jha P, Laskar S, Dubey S, Bhattacharyya MK, Bhattacharyya S. 2017. Plasmodium Hsp40 and human Hsp70: a potential cochaperone-chaperone complex. Mol Biochem Parasitol. 214:10–13. http://doi.org/10.1016/j.molbiopara.2017.03.003.
- Kayamba F, Malimabe T, Ademola IK, Pooe OJ, Kushwaha ND, Mahlalela M, van Zyl RL, Gordon M, Mudau PT, Zininga T, et al. 2021. Design and synthesis of quinoline-pyrimidine inspired hybrids as potential plasmodial inhibitors. Eur J Med Chem. 217:113330. https://doi.org/10.1016/j.ejmech.2021.113330.
- Keyzers RA, Gray CA, Schleyer MH, Whibley CE, Hendricks DT, Davies-Coleman MT. 2006. Malonganenones A-C, novel tetraprenylated alkaloids from the Mozambique gorgonian Leptogorgia gilchristi. Tetrahedron. 62(10):2200–2206.
- Kityk R, Kopp J, Mayer MP. 2018. Molecular mechanism of J-domain-triggered ATP hydrolysis by Hsp70 chaperones. Mol Cell. 69(2):227–237.e4.
- Kravats AN, Hoskins JR, Reidy M, Johnson JL, Doyle SM, Genest O, Masison DC, Wickner S. 2018. Functional and physical interaction between yeast Hsp90 and Hsp70. Proc Natl Acad Sci U S A. 115(10):E2210–9.
- Külzer S, Charnaud S, Dagan T, Riedel J, Mandal P, Pesce ER, Blatch GL, Crabb BS, Gilson PR, Przyborski JM. 2012 Nov. Plasmodium falciparum-encoded exported hsp70/hsp40 chaperone/co-chaperone complexes within the host erythrocyte. Cell Microbiol. 14(11):1784–1795.
- Kumar R, Musiyenko A, Barik S. 2003. The heat shock protein 90 of Plasmodium falciparum and antimalarial activity of its inhibitor, geldanamycin. Malar J. 2:1–11.
- Lilburn TG, Cai H, Gu J, Zhou Z, Wang Y. 2014. Exploring systems affected by the heat shock response in Plasmodium falciparum via protein association networks. Int J Comput Biol Drug Des. 7(4):369–383.
- Lindquist S, Craig EA. 1988. The heat-shock proteins. Annu Rev Genet. 22(1):631–677. https://doi.org/10.1146/annurev.ge.22.120188.003215.
- Liu K, Houry WA. 2014. Heat shock proteins of malaria. In: Shonhai A, Blatch GL, editor. Heat shock proteins of malaria. London: Springer; p. 1–223.
- Liu Q, Liang C, Zhou L. 2019. Structural and functional analysis of the Hsp70/Hsp40 chaperone system. Protein Science. 29:378–390.
- Mabate B. 2017. Exploration of interaction between Plasmodium falciparum Hsp70-x (PfHsp70-x) and human Hsp70-Hsp90 organizing protein (hHop) by, A thesis submitted in fulfillment of the requirements for the degree of Master of Science in the subject of Biochemistry at.
- Mabate B, Zininga T, Ramatsui L, Makumire S, Achilonu I, Dirr HW, Shonhai A. 2018. Structural and biochemical characterization of Plasmodium falciparum Hsp70-x reveals functional versatility of its C-terminal EEVN motif. Proteins Struct Funct Bioinforma. 86(11):1189–1201. https://doi.org/10.1016/j.compbiolchem.2019.107099.
- Machin JM, Kantsadi AL, Vakonakis I. 2019. The complex of Plasmodium falciparum falcipain-2 protease with an (E)-chalcone-based inhibitor highlights a novel, small, molecule-binding site. Malar J. 18(1):1–9. https://doi.org/10.1186/s12936-019-3043-0.
- Makumire S. 2019. Investigation of the role of the GGMP motif of Plasmodium falciparum Hsp70-1 on the chaperone function of the protein and its interaction with a co-chaperone, PfHop by Stanley Makumire submitted in fulfilment of the requirements for the degree of Doctor. University of Venda.
- Mathews ES, Jezewski AJ, Odom John AR. 2021 Jun 29. Protein prenylation and Hsp40 in thermotolerance of Plasmodium falciparum malaria parasites. MBio. 12(3):e0076021.
- Misra G, Ramachandran R. 2009. Hsp70-1 from Plasmodium falciparum: protein stability, domain analysis and chaperone activity. Biophys Chem. 142(1–3):55–64. http://doi.org/10.1016/j.bpc.2009.03.006.
- Muralidharan V, Oksman A, Pal P, Lindquist S, Goldberg DE. 2012. Plasmodium falciparum heat shock protein 110 stabilizes the asparagine repeat-rich parasite proteome during malarial fevers. Nat Commun. 3:1310–1310. http://www.nature.com/.
- Njunge J, Ludewig MH, Boshoff A, Pesce E-R, Blatch GL. 2013. Hsp70s and J proteins of plasmodium parasites infecting rodents and primates: structure, function, clinical relevance, and drug targets. Curr Pharm Des. 19(3):387–403.
- Njunge JM, Mandal P, Przyborski JM, Boshoff A, Pesce ER, Blatch GL. 2015. PFB0595w is a Plasmodium falciparum J protein that co-localizes with PfHsp70-1 and can stimulate its in vitro ATP hydrolysis activity. Int J Biochem Cell Biol. 62:47–53.
- Pavithra SR, Kumar R, Tatu U. 2007. Systems analysis of chaperone networks in the malarial parasite Plasmodium falciparum. PLoS Comput Biol. 3(9):1701–1715.
- Pesce ER, Acharya P, Tatu U, Nicoll WS, Shonhai A, Hoppe HC, Blatch GL. 2008. The Plasmodium falciparum heat shock protein 40, Pfj4, associates with heat shock protein 70 and shows similar heat induction and localisation patterns. Int J Biochem Cell Biol. 40(12):2914–2926.
- Pesce ER, Blatch GL.. 2014. Plasmodial Hsp40 and Hsp70 chaperones: current and future perspectives. Parasitology. 141(9):1167–1176.
- Pesce E-R, Cockburn IL, Goble JL, Stephens LL, Blatch GL. 2010. Malaria heat shock proteins: drug targets that chaperone other drug targets. Infect Disord Drug Targets. 10(3):147–157. https://www.eurekaselect.com/86120/article.
- Posfai D, Eubanks AL, Keim AI, Lu KY, Wang GZ, Hughes PF, Kato N, Haystead TA, Derbyshire ER. 2018. Identification of Hsp90 inhibitors with anti-plasmodium activity. Antimicrob Agents Chemother. 62(4):e01799-17.
- Priya PP, Grover M, Tatu US, Natarajan V, Langsley G. 2015. Characterization of precursor PfHsp60 in Plasmodium falciparum cytosol during its asexual development in human erythrocytes. PLoS One. 10(8):e0136401.
- Przyborski JM, Diehl M, Blatch GL. 2015. Plasmodial HSP70s are functionally adapted to the malaria parasite life cycle. Front Mol Biosci. 2(JUN):1–7. http://doi.org/10.3389/fmolb.2015.00034/abstract.
- Qiu XB, Shao YM, Miao S, Wang L. 2006. The diversity of the DnaJ/Hsp40 family, the crucial partners for Hsp70 chaperones. Cell Mol Life Sci. 63(22):2560–2570.
- Ramya TNC, Surolia N, Surolia A. 2006. 15-Deoxyspergualin modulates Plasmodium falciparum heat shock protein function. Biochem Biophys Res Commun. 348(2):585–592.
- Ramya TNC, Surolia N, Surolia A. 2007. 15-Deoxyspergualin inhibits eukaryotic protein synthesis through eIF2α phosphorylation. Biochem J. 401(2):411–420.
- Reiter K, Mukhopadhyay D, Zhang H, Boucher LE, Kumar N, Bosch J, Matunis MJ. 2013. Identification of biochemically distinct properties of the small ubiquitin-related modifier (SUMO) conjugation pathway in Plasmodium falciparum. J Biol Chem. 288(39):27724–27736.
- Reiter KH, Matunis MJ. 2016. Detection of SUMOylation in Plasmodium falciparum. Methods in Molecular Biology. 1475:283–290.
- Richter K, Buchner J. 2001. Hsp90: chaperoning signal transduction. J Cell Physiol. 188(3):281–290.
- Rodina A, Vilenchik M, Moulick K, Aguirre J, Kim J, Chiang A, Litz J, Clement CC, Kang Y, She Y, et al. 2007. Selective compounds define Hsp90 as a major inhibitor of apoptosis in small-cell lung cancer. Nat Chem Biol. 3(8):498–507.
- Rug M, Maier AG. 2011. The heat shock protein 40 family of the malaria parasite Plasmodium falciparum. IUBMB Life. 63(12):1081–1086.
- Salomane N, Pooe OJ, Simelane MBC. 2021. Iso-mukaadial acetate and ursolic acid acetate inhibit the chaperone activity of Plasmodium falciparum heat shock protein 70-1. Cell Stress Chaperones. 26(4):685–693.
- Sannella AR, Messori L, Casini A, Francesco Vincieri F, Bilia AR, Majori G, Severini C. 2007. Antimalarial properties of green tea. Biochem Biophys Res Commun. 353(1):177–181.
- Schulze J, Kwiatkowski M, Borner J, Schlüter H, Bruchhaus I, Burmester T, Spielmann T, Pick C. 2015. The Plasmodium falciparum exportome contains non-canonical PEXEL/HT proteins. Mol Microbiol. 97(2):301–314.
- Seraphim T V, Chakafana G, Shonhai A, Houry WA. 2019. Plasmodium falciparum R2TP complex: driver of parasite Hsp90 function. Biophys Rev. 11(6):1007–1015.
- Shahinas D, Folefoc A, Pillai DR. 2013a. Targeting plasmodium falciparum Hsp90: towards reversing antimalarial resistance. Pathogens. 2(1):33–54.
- Shahinas D, Folefoc A, Taldone T, Chiosis G, Crandall I, Pillai DR. 2013b. A purine analog synergizes with chloroquine (CQ) by targeting Plasmodium falciparum Hsp90 (PfHsp90). PLoS One. 8(9):e75446.
- Shonhai A. 2010. Plasmodial heat shock proteins: targets for chemotherapy. FEMS immunology & medical microbiology. 58(1):61–74. https://doi.org/10.1111/j.1574-695X.2009.00639.x
- Shonhai A, Boshoff A, Blatch GL. 2005. Plasmodium falciparum heat shock protein 70 is able to suppress the thermosensitivity of an Escherichia coli DnaK mutant strain. Mol Genet Genomics. 274(1):70–78.
- Shonhai A, Boshoff A, Blatch GL. 2007. The structural and functional diversity of Hsp70 proteins from Plasmodium falciparum. Protein Sci. 16(9):1803–1818.
- Stephens LL, Shonhai A, Blatch GL. 2011. Co-expression of the Plasmodium falciparum molecular chaperone, PfHsp70, improves the heterologous production of the antimalarial drug target GTP cyclohydrolase I, PfGCHI. Protein Expr Purif. 77(2):159–165. http://doi.org/10.1016/j.pep.2011.01.005.
- Tavella TA, Da Silva NSM, Spillman N, Kayano ACAV, Cassiano GC, Vasconcelos AA, Camargo AP, da Silva DCB, Fontinha D, Salazar Alvarez LC, et al. 2021. Violacein-Induced chaperone system collapse underlies multistage antiplasmodial activity. ACS Infect Dis. 7(4):759–776.
- Tsai J, Douglas MG. 1996. A conserved HPD sequence of the J-domain is necessary for YDJ1 stimulation of Hsp70 ATPase activity at a site distinct from substrate binding. J Biol Chem. 271(16):9347–9354.
- USAID. 2018. President’s Malaria Initiative – Zimbabwe Country Profile.
- Utpal T, Soundara Raghavan P, Gowrishankar B. 2004. A Novel Assay to Screen for Anti-Malarials.
- Wayne N, Mishra P, Bolon DN. 2011. Hsp90 and client protein maturation. Methods Mol Biol. 787:33–44.
- World Health Organization. 2021. World malaria report 2021 [Internet]. [cited 2022 Feb 12]. https://apps.who.int/iris/rest/bitstreams/1398397/retrieve.
- Yeo SJ, Liu DX, Park H. 2015. Potential interaction of Plasmodium falciparum Hsp60 and calpain. Korean J Parasitol. 53(6):665–673.
- Yuan X, Yin P, Hao Q, Yan C, Wang J, Yan N. 2010. Single amino acid alteration between valine and isoleucine determines the distinct pyrabactin selectivity by PYL1 and PYL2. J Biol Chem. 285(37):28953–8.
- Zhang M, Wang C, Oberstaller J, Thomas P, Otto TD, Casandra D, Boyapalle S, Adapa SR, Xu S, Button-Simons K, Mayho M. 2021. The apicoplast link to fever-survival and artemisinin-resistance in the malaria parasite. Nat Commun. 12(1):1–15. http://doi.org/10.1038/s41467-021-24814-1.
- Zininga T. 2015. Characterization of heat shock protein 70-z (PfHsp70-z) from Plasmodium falciparium; (November). http://univendspace.univen.ac.za/handle/11602/619.
- Zininga T, Achilonu I, Hoppe H, Prinsloo E, Dirr HW, Shonhai A. 2015. Overexpression, purification and characterisation of the Plasmodium falciparum Hsp70-z (PfHsp70-z) protein. PLoS One. 10(6):1–13.
- Zininga T, Anokwuru CP, Sigidi MT, Tshisikhawe MP, Ramaite IID, Traoré AN, Hoppe H, Shonhai A, Potgieter N. 2017a. Extracts obtained from pterocarpus angolensis dc and ziziphus mucronata exhibit antiplasmodial activity and inhibit heat shock protein 70 (Hsp70) function. Molecules. 22(8):1224.
- Zininga T, Pooe OJ, Makhado PB, Ramatsui L, Prinsloo E, Achilonu I, Dirr H, Shonhai A. 2017b. Polymyxin B inhibits the chaperone activity of Plasmodium falciparum Hsp70. Cell Stress Chaperones. 22(5):707–715.
- Zininga T, Ramatsui L, Makhado PB, Makumire S, Achilinou I, Hoppe H, Dirr H, Shonhai A. 2017c. (−)-Epigallocatechin-3-gallate inhibits the chaperone activity of Plasmodium falciparum Hsp70 chaperones and abrogates their association with functional partners. Molecules. 22(12):2139.
- Zininga T, Ramatsui L, Shonhai A. 2018. Heat shock proteins as immunomodulants. Molecules. 23(11):2846.
- Zininga T, Shonhai A. 2014. Are heat shock proteins druggable candidates? Am J Biochem Biotechnol Sci Publ. 10(2014):208–210.
- Zininga T, Shonhai A. 2019. Small molecule inhibitors targeting the heat shock protein system of human obligate protozoan parasites. Int J Mol Sci. 20(23):1–29.