Abstract
Background: Acute pancreatitis (AP) is a typical destructive inflammation of the pancreas. The direct effect of L. wallichii on AP remains unknown.
Methods: AP animal model was constructed by intraperitoneal injection of caerulein in male Sprague–Dawley rats. L. wallichii extracts were used to treat rats with AP. Then, tissue injury was evaluated by hematoxylin and eosin staining. Serum indicators, including amylase, lipase, KL-6, creatinine and urea, were measured by commercial kits. GC/MS-based metabolomics was utilized to characterize the metabolome.
Results: Intraperitoneal injection of L. wallichii extracts protected against lung, liver, and kidney injury in rats with caerulein-induced AP. GC/MS-based metabolomics analysis revealed that L. wallichii treatment could restore caerulein-induced alteration of the abundance of most metabolites. These differential metabolites enriched twelve metabolic pathways that were able to be reprogramed by L. wallichii administration. L. wallichii impacted arginine metabolism by inhibiting arginase expression and activity. Inhibition of arginase by S-(2-boronoethyl)-L-cysteine (BEC) could relieve caerulein-induced AP, suggesting that suppression of arginase is a potential mechanism for L. wallichii to ameliorate AP.
Conclusion: Our data provide the inter-relationship between metabolic reprograming and anti-AP effects of L. wallichii extracts in rats, and highlight the potential of metabolic reprograming against AP pathogenesis.
Introduction
Acute pancreatitis (AP) is a devastating inflammation caused by prematurely activated digestive proteases and leads to 10% mortality of patients. Approximately 10-35% of AP patients who survive the initial attack will go on to develop recurrent pancreatitis, of which 6-13% may develop chronic pancreatitis (Muddana et al. Citation2009; Sharma et al. Citation2021). Pancreatic injury and inflammation lead to systemic inflammatory response syndrome, and systemic single or multiple organ failure occurs in the early stage of AP, which is a major cause of high morbidity and mortality (Gukovskaya et al. Citation2017). However, the leading therapeutics for the early stage of AP are limited and merely consist of supportive medical treatment modalities (Watanabe et al. Citation2017). Thus, early therapeutic intervention to relieve injury and inflammation in the pancreas may be a potential strategy to improve the outcomes of AP.
Ligusticum wallichii (family Umbelliferae) is a traditional Chinese herbal medicine that can supply therapeutic intervention in cardiovascular and neurovascular disorders (Rastogi et al. Citation2016). The main bioactive components identified from this herb are ligustrazine, ferulic acid, cnidilide, and ligustilide (Liu et al. Citation2016). Ligustrazine can relieve AP-induced injury to pancreas and kidney (Xiang et al. Citation2017). Ferulic acid has been demonstrated to exert promising effects against oxidative stress, inflammatory reactions, and AP (Hassanzadeh et al. Citation2021). Surprisingly, the full effect of this herb on AP has not been investigated so far. The validation of the bioactivity of L. wallichii in AP has great practical and economic value for patients as the price of this herb is much cheaper than purified component ligustrazine and ferulic acid in China. Thus the major goal of the current study is to estimate the therapeutic effect of L. wallichii on AP in animals. In the current study, GC/MS-based metabolome was utilized to illustrate the valuable metabolisms, essential biomarkers contributing to metabolic features, and metabolic mechanisms in serum samples obtained from experimental rats.
Materials and methods
Preparation and treatment of Ligusticum wallichii extracts
The Soxhlet extractor loading with 2000ml deionized water was utilized to continuously decoct from a total of 200 grams of the L. wallichii powder (Chinese Herbs DIRECT, MW4801SFP). The water-soluble extracts from L. wallichii were subsequently harvested, centrifuged, filtered, concentrated, lyophilized, and weighed. The lyophilized powders were dissolved in PBS at a final concentration of 10 mg/ml. This solution was aliquoted and preserved at −80°C (Wei et al. Citation2020).
Experimental acute pancreatitis (AP) model in rat
The male Sprague–Dawley outbred rats aged 8–12 weeks were commercially available from the Animal Center of Dalian Medical University. They were housed in groups (6 rats per group, 2 rats per cage, and 24 rats in total) in 12-h light cycles. The animal protocol complied with the ARRIVE guidelines and was approved by the Institutional Animal Care and Use Committee of Dalian Medical University (Approval No. 2020DMUIACUC1987). They were intraperitoneally injected with caerulein at 150 µg/kg each time at 1 h intervals, and for a total of 6 times, to generate AP rat models (Chen et al. 016). In PBS or caerulein-injected rats, the L. wallichii extracts (150 mg/kg per day) or BEC [S-(2-boronoethyl)-L-cysteine, an arginase inhibitor at 10 mg/kg] were intraperitoneally injected 7, 9, 11 and 15 h after the first PBS or caerulein injection, so the treatment with L. wallichii and BEC for AP animals was therapeutic. After 24 h post-injection of caerulein, mice were euthanized by carbon dioxide inhalation, blood was collected, and tissues (pancreas, lung, and kidney) were retrieved into liquid nitrogen or 10% buffered formalin as appropriate.
Detection of serum amylase, lipase, arginase, KL-6, creatinine, MPO, MDA, and urea
The serum amylase was determined by using the Phadebas reagent (Magle Life Sciences). The serum lipase activity was measured by the lipid assay kits (Jiancheng Biotech, Nanjing, China). The rat KL-6 ELISA kit was obtained from ABclonal. An Arginase Activity Assay kit (MAK112, Sigma-Aldrich) was used to detect the activity of arginase in rat sera and renal samples. The creatinine and urea in sera samples were determined by using commercial kits (Alpha Laboratories Ltd, UK). MDA (A003-1, malondialdehyde (MDA) assay kit (TBA method), Nanjing Jiancheng Bioengineering Institute, China) and myeloperoxidase (MPO) activity (A044, MPO assay kit, Beyotime, China) were investigated in indicated tissues employing commercially available kits according to the manufacturer’s specifications.
Histological analysis
Pancreas, lung, and renal samples were dipped in 10% formalin for 24 h, embedded in paraffin, and sectioned. For histological analysis, paraffin sections were stained with hematoxylin and eosin (H & E) (Zhang et al. Citation2018). For the observation of vacuolization, interstitial edema, interstitial inflammation, and the number of acinar cell necroses, multiple arbitrarily chosen microscopic fields from at least three rats in each group were blindly examined by two pathologists, as previously described (Kubisch et al. Citation2004).
Metabolomics analysis
The previously published protocol was conducted to obtain the total metabolites (Li et al. Citation2015). Briefly, 50 µL rat serum was mixed with 50 µL 100% cold methanol to precipitate the proteins. After spinning at 12,000 rpm for 5 min, the cleared supernatant was saved. Ribitol (5 µL 0.1 mg/mL), an internal analytical standard, was included in this supernatant and then lyophilized. After oxidating and deriving, the metabolites in each sample were separated by Thermo Scientific Trace DSQ II. Two technical replicates were performed for each sample. For data processing, AMDIS and internal standards were simultaneously applied to spectral deconvolution and calibration. The XCalibur software (Thermo Fisher, version 2.1) was used to identify compounds by matching the GC-MS spectra to different metabolites. Rt-m/z pairs formed a single matrix with resulting normalized peak intensities. The data were normalized by total amount and ribitol corrections (Su et al. Citation2018). To obtain differential metabolites from metabolomic data, a permutation-based hypothesis testing method, designated significant analysis of microarray (SAM), was applied (Su et al. Citation2018). ClustVis was employed to create heat maps (Metsalu and Vilo Citation2015). Independent component analysis (ICA) was performed in a web-based tool: MetaGeneAlyse (Morgenthal et al. Citation2005). Metabolic pathways were enriched by utilizing MetaboAnalyst 4.0 (Chong et al. Citation2018).
Quantitative real time-polymerase chain reaction (RT–PCR)
The total RNA of blood and kidney was isolated by using the RNeasy RNA Isolation kit (Qiagen). The cDNA was synthesized by applying LunaScript® RT SuperMix Kit (New England BioLabs). All subsequent PCR reaction was carried out according to the protocol in 7 Universal PCR Master Mix (Applied Biosystems). The relative mRNA transcriptional levels were standardized against housekeeping gene coding for β-Actin (actb). The gene expression levels were calculated using the 2−ΔΔCT method. The nucleotide sequences of primers were as follows: arg1 primers (5′- TGAGCTTTGATGTCGACGGG-3′ and 5′- GTTGAGTTCCGAAGCAAGCC-3′), arg2 primers (5′-GGGGACAGAAGAAGCTAGG-3′ and 5′-ACTTCAGCCAGTT CCTGGTTG-3′), and actb primers (5′-AGAAGAGCTATGAGCTGCCTGACG-3′ and 5′-TACTTGCGCTCAGGAGGAGCAATG-3′).
Statistical analysis
Statistical significance among groups was calculated with the unpaired two-tailed Student t-test, one-way ANOVA, or two-way ANOVA as appropriate. All data were evaluated and visualized by GraphPad Prism 7, and statistical significance was indicated as follows: *, P < 0.05; **, P < 0.01.
Results
L. wallichii extracts alleviate pancreatic damage in caerulein-induced rats
AP was primarily triggered by intraperitoneal injection of a practical dose of caerulein (150 µg/kg) for 6 h, and then treated with L. wallichii extracts three times (150 mg/kg). Histological analysis of the pancreas in caerulein-induced rats showed the pancreatic injury was characterized by marked edema, inflammatory cell infiltration and a large number of necrotic acinar cells (Figure A), thereby causing higher histological scores for both pancreatic damage and inflammation (Figure B). Moreover, by performing two-way ANOVA, we found that serum amylase and lipase activity were significantly increased 3 fold in rats with AP (Figure C and D). Interestingly, pancreatic injury and the activity of serum amylase and lipase declined 2 fold in the L. wallichii-treated caerulein-exposed rats compared with the untreated caerulein-exposed rats (Figure A to D). The pancreatic MPO activity and MDA level in the L. wallichii-treated caerulein-exposed group was 58.8% and 74.3% weaker, respectively than that in the untreated caerulein-exposed group (Figure E and F).
Figure 1. L. wallichii extracts attenuated pancreatic, lung, and renal damage in caerulein-induced rats. (A) H&E staining of pancreas tissue from each group showed different levels of tissue damage including pancreatic edema, extravascular infiltration and acinar cell necrosis (×100). (B) Histologic inflammatory score of (A), (G), and (K). (C and D) The activity of serum amylase (C) and lipase (D) in each group (n = 6 per group). (E) Quantification of MPO activity in the pancreas tissues of each group. (F) Quantification of MDA content in the pancreatic tissues of each group. (G) H&E staining of lung tissue from each group presented different levels of tissue damage including thickening of the alveoli, neutrophil infiltration, and alveolar congestion (×200). (H) The level of serum KL-6 in each group (n = 6 per group). (I) Quantification of MPO activity in the lung tissues of each group. (J) Quantification of MDA content in the lung tissues of each group. (K) H&E staining of kidney tissue exhibited identifiable features of kidney injury including typical histological signs of glomerular and tubular damage. (×100). (L, M, and N) Quantification of serum creatinine (L), urea (M) and renal MDA (N) from each group (n = 6 per group). Mean ± SEM, *p < 0.05, **p < 0.01.
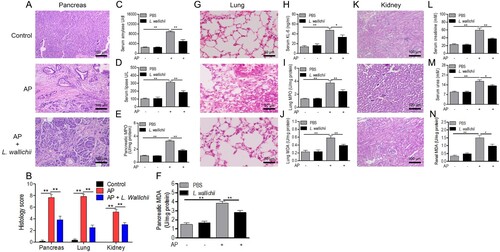
L. wallichii extracts relieve lung damage in caerulein-induced rats
AP often causes respiratory failure, thereby owning a high risk of death. Acute lung injury in AP has features consistent with respiratory failure (Mole et al. Citation2016). The caerulein-exposed rats presented pathological markers such as slight thickening of the alveoli, neutrophil infiltration, and alveolar congestion (Figure G). The administration of L. wallichii extracts in caerulein-treated rats showed more thinning of the alveoli and neutrophil infiltration. Krebs von den Lungen 6 (KL-6), a circulating serum marker of acute lung injury, was induced by caerulein but was repressed when L. wallichii extracts were applied to caerulein-induced rats (Figure H). Then, the lung damage was further evaluated by MPO activity and MDA levels and confirmed that MPO activity and MDA levels in lung were markedly enhanced by caerulein administration (Figure I and J). With the treatment of L. wallichii extracts, the levels of MPO activity and MDA were significantly lower than in the untreated caerulein-exposed group by applying two-way ANOVA (Figure I and J).
L. wallichii extracts protect against renal injury in rats with AP
AP is frequently complicated by renal injury. Thus we next determined whether L. wallichii extracts have protections for AP-related renal injury. The control group showed normal histological features of renal glomerulus, tubule, and interstitium (Figure K). The identifiable structure of renal injury including typical histological signs of glomerular and tubular damage was observed in the AP group. In contrast, the administration of L. wallichii extracts ameliorated the damage (Figure K). Moreover, by implementing two-way ANOVA, we discovered a significant loss of renal function as demonstrated by 300% and 220% enhancement of serum creatinine and urea, respectively, after caerulein injection (Figure L and M). The renal damage was further validated by the elevated levels of MDA (Figure N). All data suggested the therapeutic effect of L. wallichii extracts on AP-related renal injury.
Differential serum metabolome associated with AP and L. wallichii administration
To reveal the vital crucial metabolites and accompanying metabolic pathways that result in the therapeutic effect of L. wallichii extracts on AP-treated rats, GC/MS was applied to value the level of metabolites quantitatively. As presented in Figure A, each sample harboring 74 metabolites was clustered as a heat map. When comparing between the control and AP groups, and between AP and AP + L. wallichii groups, 60 and 47 metabolites were differential at P < 0.05, respectively. Out of them, 44 metabolites were shared in both comparisons (Figure B to D). A heat map was applied to present the differential metabolites according to their relative abundances across samples (Figure B and C). In the AP group, the levels of 41 and 19 metabolites were up-regulated and down-regulated, respectively. In the AP + L. wallichii-treated group, the levels of 19 and 28 metabolites were increased and decreased, respectively (Figure B to D). Despite common metabolites, 12 metabolites had enhanced, and four metabolites had reduced levels in the AP group, while only 3 metabolites had enhanced levels in the AP + L. wallichii group (Figure D). Among 44 common metabolites, two metabolites had consistently up-regulated levels in AP and AP + L. wallichii groups, 27 metabolites had up-regulated levels in the AP group but down-regulated levels in the AP + L. wallichii group, and 14 metabolites had down-regulated levels in the AP group but up-regulated levels in the AP + L. wallichii group (Figure D), suggesting that the reduction or induction of most differential metabolites (n = 41) by AP was able to be re-modulated by the treatment of L. wallichii. Furthermore, we assessed the metabolic categories of differential metabolites and found that the administration of L. wallichii extracts had more impact in lipid metabolism (Figure E).
Figure 2. Differential metabolome related to AP and L. wallichii treatment. (A) Hierarchical clustering showing the 74 metabolites. Green and blue present an increase and decrease of metabolites relative to the median metabolite level, respectively (see color scale). (B) Heat map for relative abundances of differential metabolites (n = 60) in the serum of AP-induced rats compared with the control. (C) Heat map for relative abundances of differential metabolites (n = 47) in the serum of AP + L. wallichii-treated rats compared with the serum of AP-induced rats. Green and blue present an increase and decrease of metabolites relative to the median metabolite level, respectively (see color scale). (D) Venn diagram displaying the overlap of differential metabolites between the AP and AP + L. wallichii groups. (E) The number of metabolites up-regulated and down-regulated in different metabolic categories.
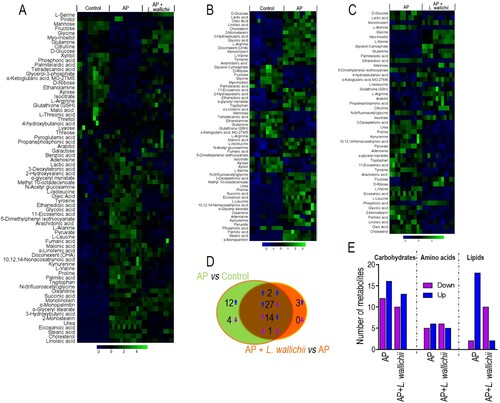
Identification of metabolic pathways associated with therapeutic effect triggered by L. wallichii extracts
Results of ICA were able to distinguish control, AP, and AP + L. wallichii groups (Figure A), which suggested an essential inter-relationship between the metabolomics response and degree of AP. Then, the metabolic pathways were enriched by all common metabolites displayed in Figure D. In total, twelve pathways were obtained when P < 0.05 was used as an evaluation indicator (Figure B). Out of them, five pathways were involved in amino acid metabolisms. These pathways included: valine, leucine, and isoleucine biosynthesis; glycine, serine, and threonine metabolism; d-glutamine and d-glutamate metabolism; arginine biosynthesis; and alanine, aspartate, and glutamate metabolism. Moreover, five pathways were related to carbohydrate metabolism. These pathways included: pyruvate metabolism; glyoxylate and dicarboxylate metabolism; galactose metabolism; TCA cycle; and butanoate metabolism. The only lipid metabolism was the biosynthesis of unsaturated fatty acids. Thus, all pathway-involved metabolites were further visualized in Figure C for understanding the metabolic shift of serum in rats treated by AP or AP + L. wallichii treatment.
Figure 3. Pathway enrichment analysis and potential metabolic mechanisms of AP-induced injury and anti-AP activity of L. wallichii extracts. (A) ICA directly reveals a variety of metabolites among control, AP, and AP + L. wallichii groups. Each dot in the panel indicates the replicate analysis of samples. (B) By using an online tool, Metaboanalyst 4.0 (http://www.metaboanalyst.ca/), several pathways were enriched. (C) The metabolic flow of serum in AP-induced or AP + L. wallichii–induced rats. Symbol circle and square represent the relative metabolite changes in the AP group and the AP + L. wallichii groups, respectively. The decrease, increase and no change in levels with statistical significance are presented in purple, blue and orange, respectively.
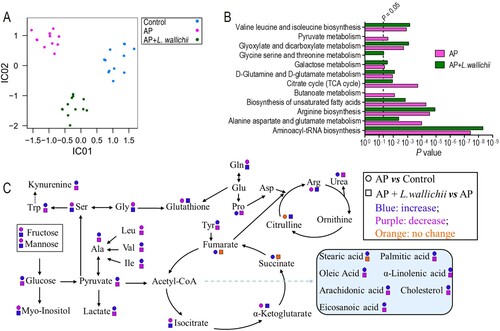
L. wallichii inhibits caerulein-induced arginase expression and activity, and inhibition of arginase activity by BEC ameliorates caerulein-induced AP in rats
After stimulation by caerulein, the rat serum had a lower l-arginine level and higher urea level relative to the control groups (Figure C), suggesting that caerulein induced arginase activity. As expected, QRT-PCR analysis showed the increased transcriptional levels of arg1 and arg2 and arginase activity in AP blood and kidney samples (Figure A to C). Interestingly, L. wallichii administration ameliorated caerulein-induced arginase expression and activity in rat blood and kidney. Testing an arginase inhibitor, BEC, in AP rats showed that inhibition of arginase activity reduced the caerulein-induced serum amylase, lipase, and pancreatic MPO levels (Figure D to F). BEC treatment also blocked the arginase activity and serum urea level in AP animals (Figure G and H). Histological analysis of different tissues (pancreas, lung, and kidney) in rats showed that tissue injury and inflammation induced by AP could be improved by the BEC treatment (Figure I and J). These data suggest that suppressing arginase activity could be a potential therapeutic intervention for the treatment of caerulein-induced AP.
Figure 4. Inhibition of arginase is a potential mechanism for L. wallichii to relieve AP in rats. (A and B) The mRNA level of two arginase (arg1 and arg2) isoforms in the blood (A) and kidney (B) samples that were isolated from caerulein-induced rats treated with or without L. wallichii. (C) The arginase activity in blood and kidney samples that were isolated from caerulein-induced rats treated with or without L. wallichii. (D to F) The impact of a competitive arginase inhibitor, BEC (S-(2-boronoethyl)-L-cysteine), on serum amylase (D), lipase (E), and pancreatic MPO levels (F) of AP-injured rats. (G) The arginase activity in blood samples from caerulein-induced rats treated with or without BEC. (H) Quantification of serum urea from caerulein-induced rats treated with or without BEC. (I) H&E staining of pancreas, lung, and kidney from each group indicated different levels of tissue damage. (J) Histologic inflammatory score calculated from (I). Mean ± SEM, *p < 0.05, **p < 0.01.
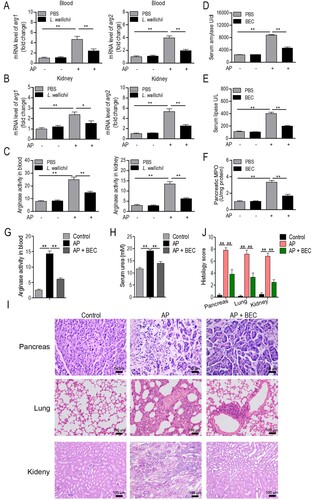
Discussion
AP is a systemic inflammatory disease that causes leukocyte-endothelium, platelet-endothelium, and platelet-leukocyte interactions and vascular thrombosis due to excessive release of inflammatory cytokines and vasoactive substances, thereby inducing microcirculation disturbance and eventually pathological changes in multiple organs (Dobosz et al. Citation2005). Ischemia, hypoxia, and reperfusion injury caused by microcirculation disturbance are the main reasons for the progression of extra-pancreatic organ damage in AP (Zhang et al. Citation2011). The present study suggested that the pancreas, lung, and kidney of rats in the AP group presented diverse degrees of pathological damage with edema, acinar necrosis, and inflammatory cell infiltration, as well as the change of some serological indicators. Interestingly, L. wallichii extracts had therapeutic effects on AP model rats by restoring the above injury and serological indicators. In the subsequent investigation, we confirmed a mechanistic clue that metabolic response was likely involved in the anti-AP function of L. wallichii extracts on rats by the detection of key pathways and vital metabolites.
We primarily found that AP-induced lipid metabolism could be reduced by L. wallichii extracts from the analysis of the metabolic category. The further pathway enrichment analysis clarified that the biosynthesis of unsaturated fatty acids is potentially correlated with the L. wallichii-triggered metabolic response. Six fatty acids, arachidonic acid, linoleic acid, stearic acid, oleic acid, eicosanoic acid, and palmitic acid, were included in this metabolism. The previous report confirmed that the AP patients with complications had higher levels of serum fatty acids (palmitic acid, stearic acid, oleic acid, linoleic acid, linolenic acid, and arachidonic acid) than healthy volunteers (Sztefko and Panek Citation2001). Adiponectin, a protein hormone regulating fatty acid oxidation, owns a protective activity in caerulein-induced AP in high-fat diet-fed animals (Araki et al. Citation2008). The addition of oleic acid to isolated pancreatic acinar cells leads to the stimulation of amylase release and cell damage (Yang et al. Citation2009). Arachidonic acid triggers a TLR4-dependent inflammatory response in pancreatic acinar cells of rats (Mateu et al. Citation2015). Thus, inhibiting the level of serum fatty acids mentioned above is a reliable metabolic mechanism for L. wallichii-triggered protection against AP in rats.
It is generally believed that AP significantly enhances proteolysis, resulting in changes in serum concentration of a series of amino acids (O’Keefe and McClave Citation2005 Meier and Beglinger Citation2006;). Results from the current investigation also displayed an AP-induced alteration of amino acid levels. The following analysis of the metabolic pathway evidenced that L. wallichii modulated five amino acid metabolisms (valine, leucine, and isoleucine biosynthesis; glycine, serine, and threonine metabolism; d-glutamine and d-glutamate metabolism; arginine biosynthesis; and alanine, aspartate, and glutamate metabolism) on AP rats. The administration of L. wallichii extracts could reprogram the level of most involved amino acids that were down-regulated or up-regulated by AP induction. Among them, several amino acids, including glutamine, serine, glycine, arginine, and tryptophan, play roles in the pathogenesis of AP. Oxidative stress is a key contributor to the development of AP and can be alleviated by glutathione, a tri-peptide (glutamate-cysteine–glycine) antioxidant that prevents damage caused by reactive oxygen species (ROS) (Lutgendorff et al. Citation2008; Escobar et al. Citation2009; Abreu et al. Citation2016). Here, AP-induced loss of glycine, l-serine, l-glutamine, and glutathione were reversed by the administration of L. wallichii extracts, suggesting that the regulation of these metabolites seems to rationally explain how L. wallichii is durably protective against AP. Not only ROS, nitric oxide (NO) also acts as an essential mediator in oxidative stress. L-arginine can only be metabolized by nitric oxide synthase (NOS) and arginase; both enzymes’ expression and activity are enhanced during AP (Al-Mufti et al. Citation1998; Scibior et al. Citation2006), indicating the strong requirement and consumption of l-arginine. Consistently, our data showed that the rats with AP have a lower abundance of l-arginine and a higher abundance of urea (the enzymatic product of cellular arginase) than the control. The AP-induced changes of l-arginine and urea were relieved by the administration of L. wallichii extracts. More importantly, L. wallichii can attenuate caerulein-triggered arginase expression and activity. All these data show that improving arginase activity is a potentially fundamental metabolic mechanism for L. wallichii-induced protection in rats with AP. One limitation of our study is that the metabolite profile was only detected in serum samples, the tissue-specific changes in metabolite need to be further examined.
Authors’ contributions
All authors contributed to the study conception and design. Material preparation, data collection, experiment design, experiment implementation and analysis were performed by YJ, HW and XL, who also wrote the first draft of the manuscript. HW and JL confirm the authenticity of all the raw data. YJ and XL provided valuable opinions and reviewed the draft of the paper. All authors have read and approved the final manuscript.
Availability of data and materials
The datasets used and/or analyzed during the current study are available at http://www.scidb.cn/doi/10.11922/sciencedb.01218.
Disclosure statement
No potential conflict of interest was reported by the author(s).
References
- Abreu FF, Souza ACA, Teixeira SA, Soares AG, Teixeira DF, Soares RC, Santana MT, Lauton Santos S, Costa SKP, Muscará MN. 2016. Elucidating the role of oxidative stress in the therapeutic effect of rutin on experimental acute pancreatitis. Free Radical Res. 50:1350–1360.
- Al-Mufti R, Williamson R, Mathie R. 1998. Increased nitric oxide activity in a rat model of acute pancreatitis. Gut. 43:564–570.
- Araki H, Nishihara T, Matsuda M, Fukuhara A, Kihara S, Funahashi T, Kataoka TR, Kamada Y, Kiyohara T, Tamura S. 2008. Adiponectin plays a protective role in caerulein-induced acute pancreatitis in mice fed a high-fat diet. Gut. 57:1431–1440.
- Chong J, Soufan O, Li C, Caraus I, Li S, Bourque G, Wishart DS, Xia J. 2018. MetaboAnalyst 4.0: towards more transparent and integrative metabolomics analysis. Nucleic Acids Res. 46:W486–WW94.
- Dobosz M, Hac S, Mionskowska L, Dymecki D, Dobrowolski S, Wajda Z. 2005. Organ microcirculatory disturbances in experimental acute pancreatitis: a role of nitric oxide. Physiol Res. 54:363.
- Escobar J, Pereda J, Arduini A, Sandoval J, Sabater L, Aparisi L, López-Rodas G, Sastre J. 2009. Cross-talk between oxidative stress and pro-inflammatory cytokines in acute pancreatitis: a key role for protein phosphatases. Curr Pharm Des. 15:3027–3042.
- Gukovskaya AS, Gukovsky I, Algül H, Habtezion A. 2017. Autophagy, inflammation, and immune dysfunction in the pathogenesis of pancreatitis. Gastroenterology. 153:1212–1226.
- Hassanzadeh P, Arbabi E, Rostami F. 2021. Coating of ferulic acid-loaded silk fibroin nanoparticles with neutrophil membranes: A promising strategy against the acute pancreatitis. Life sciences. 270:119128.
- Kubisch C, Dimagno MJ, Tietz AB, Welsh MJ, Ernst SA, Brandt-Nedelev B, Diebold J, Wagner AC, Göke B, Williams JA. 2004. Overexpression of heat shock protein Hsp27 protects against cerulein-induced pancreatitis. Gastroenterology. 127:275–286.
- Li J, Zhu S-F, Zhao X-L, Liu Y-X,, Wan M-H, Guo H, Liu Y-L, Gong H-L, Chen G-Y, Tang W-F. 2015. Metabolomic profiles illuminate the efficacy of Chinese herbal Da-Cheng-Qi decoction on acute pancreatitis in rats. Pancreatology. 15:337–343.
- Liu X, Li X, Ji S, Cui X, Li M. 2016. Screening of bioactive ingredients in ligusticum chuanxiong hort for protection against myocardial ischemia. Cell Physiol Biochem. 40:770–780.
- Lutgendorff F, Trulsson LM, van Minnen LP, Rijkers GT, Timmerman HM, Franzén LE, Gooszen HG, Akkermans LM, Soderholm JD, Sandstrom PA. 2008. Probiotics enhance pancreatic glutathione biosynthesis and reduce oxidative stress in experimental acute pancreatitis. Am J Physiol-Gastrointestinal and Liver Physiol. 295:G1111–G1G21.
- Mateu A, Ramudo L, Manso M, De Dios I. 2015. Cross-talk between TLR4 and PPARγ pathways in the arachidonic acid-induced inflammatory response in pancreatic acini. Int J Biochem Cell Biol. 69:132–141.
- Meier RF, Beglinger C. 2006. Nutrition in pancreatic diseases. Best Pract Res Clin Gastroenterol. 20:507–529.
- Metsalu T, Vilo J. 2015. ClustVis: a web tool for visualizing clustering of multivariate data using Principal Component Analysis and heatmap. Nucleic Acids Res. 43:W566–WW70.
- Mole DJ, Webster SP, Uings I, Zheng X, Binnie M, Wilson K, Hutchinson JP, Mirguet O, Walker A, Beaufils B. 2016. Kynurenine-3-monooxygenase inhibition prevents multiple organ failure in rodent models of acute pancreatitis. Nat Med. 22:202.
- Morgenthal K, Wienkoop S, Scholz M, Selbig J, Weckwerth W. 2005. Correlative GC-TOF-MS-based metabolite profiling and LC-MS-based protein profiling reveal time-related systemic regulation of metabolite–protein networks and improve pattern recognition for multiple biomarker selection. Metabolomics. 1:109–121.
- Muddana V, Whitcomb DC, Papachristou GI. 2009. Current management and novel insights in acute pancreatitis. Expert Rev Gastroenterol Hepatol. 3:435–444.
- O’Keefe SJ, McClave SA. 2005. Feeding the injured pancreas. Gastroenterol. 129:1129–1130.
- Rastogi S, Pandey MM, Rawat A. 2016. Traditional herbs: a remedy for cardiovascular disorders. Phytomedicine. 23:1082–1089.
- Scibior D, Ashamiss F, Wierzbicki Z, Barańczyk-Kuźma A. 2006. Arginase activity in blood serum of patients with acute and chronic pancreatitis. Polski Merkuriusz Lekarski: Organ Polskiego Towarzystwa Lekarskiego. 21:522–524.
- Sharma D, Mallick B, Samanta J, Gupta V, Sinha SK, Kochhar R. 2021. Acute-on-chronic pancreatitis: analysis of clinical profile and outcome. Cureus. 13:e14242.
- Su Y-B, Peng B, Li H, Cheng Z-X, Zhang T-T, Zhu J-X, Li D, Li M-Y,, Ye J-Z, Du C-C. 2018. Pyruvate cycle increases aminoglycoside efficacy and provides respiratory energy in bacteria. Proc Natl Acad Sci USA. 115:E1578–E1E87.
- Sztefko K, Panek J. 2001. Serum free fatty acid concentration in patients with acute pancreatitis. Pancreatology. 1:230–236.
- Watanabe T, Kudo M, Strober W. 2017. Immunopathogenesis of pancreatitis. Mucosal Immunol. 10:283–298.
- Wei Z, Dong C, Guan L, Wang Y, Huang J, Wen X. 2020. A metabolic exploration of the protective effect of Ligusticum wallichii on IL-1β-injured mouse chondrocytes. Chin Med. 15:1–12.
- Xiang H, Zhang Q, Qi B, Tao X, Xia S, Song H, Qu J, Shang D. 2017. Chinese herbal medicines attenuate acute pancreatitis: pharmacological activities and mechanisms. Front Pharmacol. 8:216.
- Yang F, Wang Y, Sternfeld L, Rodriguez J, Ross C, Hayden M, Carriere F, Liu G, Schulz I. 2009. The role of free fatty acids, pancreatic lipase and Ca2 + signalling in injury of isolated acinar cells and pancreatitis model in lipoprotein lipase-deficient mice. Acta Physiol. 195:13–28.
- Zhang X-P, Jiang J, Cheng Q-H, Ye Q, Li W-J, Zhu H, Shen J-Y. 2011. Protective effects of Ligustrazine, Kakonein and Panax Notoginsenoside on the small intestine and immune organs of rats with severe acute pancreatitis. Hepatobiliary Pancreat Dis Int. 10:632–637.
- Zhang Y, Li W, Zou L, Gong Y, Zhang P, Xing S, Yang H. 2018. Metabonomic study of the protective effect of Fukeqianjin formula on multi-pathogen induced pelvic inflammatory disease in rats. Chin Med. 13:1–13.