Abstract
A system was developed measuring x-ray powder diffraction in high magnetic fields up to 5 T and at temperatures from 283 to 473 K. The stability of the temperature is within 1 K over 6 h. In order to examine the ability of the system, the high-field x-ray diffraction measurements were carried out for Si and a Ni-based ferromagnetic shape-memory alloy. The results show that the x-ray powder diffraction measurements in high magnetic fields and at high temperatures are useful for materials research.
Introduction
X-ray powder diffraction (XRD) measurement is a very useful and general method for investigating microscopic properties, such as the crystal structure, the crystal orientation and the crystal deformation. To develop materials, it is required that XRD measurement be carried out under various conditions. Therefore, many research groups have developed x-ray diffraction systems for use with high [Citation1] and low [Citation2] temperatures, high pressure [Citation3] and magnetic fields [Citation4, Citation5]. In the High Field Laboratory for Superconducting Materials, Institute for Materials Research, Tohoku University (HFLSM), a high-field and low-temperature x-ray powder diffraction (HF-XRD) system was developed in 1998, which is operated in fields up to 5 T and in the temperature range from 10 to 320 K [Citation6]. In this system, the magnetic field is generated by a cryocooled split-pair superconducting magnet with a Gifford–McMahon (GM) type cryocooler (helium gas closed-cycle refrigerator), and the sample temperature is controlled using another GM-type cryocooler and a heater in the sample cryostat.
On the other hand, recently, field-controlled magnetic materials are being actively studied all over the world, for example, magnetic refrigerants [Citation7–10] and ferromagnetic shape memory alloys [Citation11–13], which exhibit first-order magnetic phase transitions accompanied by structural transformation or crystal deformation. Using HF-XRD at HFLSM, several results, important for the development of field-controlled magnetic materials, have been reported [Citation14, Citation15].
There are many reports on HF-XRD measurements below room temperature. However, no systematic HF-XRD study for magnetic materials at above room temperature has been reported yet. For high-temperature HF-XRD measurement, it is required that a small furnace with an x-ray window be installed in a small bore (50 mm) of a high-field superconducting magnet, which is difficult.
In this work, we started a development of a sample furnace for HF-XRD measurement at high temperatures. The high-temperature HF-XRD measurement is performed by replacing the sample cryostat in the magnet of the low-temperature HF-XRD system with a sample furnace. Using this setup, we succeeded in HF-XRD measurements at temperature up to 473 K, and the field-induced structural transformation in a Ni-based ferromagnetic shape memory alloy was observed at 408 K. In this paper, we present the setup and typical results of the measurement.
Instrumental details
Figures (a) and (b) show the schematic diagram and overall view of the high-temperature HF-XRD system, respectively. The system mainly consists of a cryocooled split-pair NbTi superconducting magnet [Citation6], a diffractometer and a sample furnace. The magnet generates magnetic fields B up to 5 T at the centre of a vertical room-temperature bore of 50 mm and the horizontal room-temperature gap of 10 mm with the homogeneity of 0.1%. The magnet is mounted on a θ–2θ goniometer, and the sample furnace is set into the 50 mm room-temperature bore of the magnet. The apparatus employs a conventional shield tube (2 kW) for an x-ray source. A scintillation detector has a passive magnetic field shield made of silicon steel with high permeability. In order to eliminate Kβ radiation, a graphite-curved monochromator is utilized in front of the scintillation detector.
Figure 1 Schematic diagram (a) and overall view (b) of the high-temperature, high-field x-ray diffraction system.
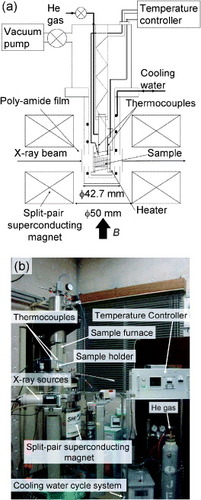
Powder is sprayed onto the sample boat (17 × 15 × 1 mm3) made of copper, and fixed using Apiezon H grease (M&I materials Ltd), which can be used in the temperature range from 264 to 513 K and exhibits good heat transfer properties. The sample boat is inserted into the sample holder made of MACOR glass-ceramic. The sample heater is wound with Nichrome wire as a noninductive coil. The temperature is controlled by the proportional-integral-derivative (PID) method using signals from two thermocouples inserted just behind the sample boat. No effect of magnetic field on the thermocouples is observed. Cooling water circulates between the outside of the furnace and the outer radiation-shield tube (42 mm diameter) to prevent radiation heat transfer to the superconducting magnet. The x-ray beam enters and leaves through the poly-amide film (75 μ m) window of the outer radiation-shield tube. The measurements are carried out in a He gas atmosphere (∼0.1 MPa) to prevent the oxidation of the powder.
The temperature of the sample can be increased up to 473 K so far. As a typical result of temperature stability, we show the time dependence of the temperatures at 473 K in figure (a) and at 398 K in figure (b). The temperature is controlled to within 1 K over 6 h at 473 K and 3 h at 398 K by resistive heating up to 40 W at 15 Ω. The diffraction data can be taken in the 2θ range from 0 to 120° with a step size of 0.002°.
Experimental results
Measurement of Si in zero and 5 T fields
In order to test the setup, we carried out HF-XRD measurement for Si (99.999%) at 300–473 K in 0 and 5 T. Here, the sample was subsequently reheated in a magnetic field of 5 T after zero-field measurements. Figure shows the typical result of the high-temperature XRD pattern of Si at 473 K in 0 T. As seen in this figure, the diffraction peaks of Cu from the copper frame of the sample holder were detected. However, we confirmed that the grease did not melt and the powder sample did not fall even at 473 K.
Figures (a) and (b) show the 422 XRD peaks of Si for 87.5° 2θ 89.0° at several temperatures in 0 and 5 T, respectively. The reflection peaks attributed to Kα 1 and Kα 2 radiations are observed. We can see that the 422 peaks shift to the lower 2θ angle side with increasing temperature, indicating thermal expansion. In addition, we note that the intensity of some peaks was suppressed. This was caused by a change in the flow rate of He gas. When the He gas was controlled so that the flow rate was steady, the intensity of the 422 peak remained almost constant in the temperature range of 323 K T 473 K in 5 T, as shown in figure (b).
The lattice parameter was determined from the position of the 422 peak. The temperature dependence of the lattice parameter a in 0 and 5 T is shown in figure . No effect of the magnetic field is observed. The average coefficient of thermal expansion α in the 273–447 K temperature range is estimated as 3.7 × 10- 6 K- 1 in 0 or 5 T, which is consistent with reported data (3.57 × 10- 6 K- 1 at 430 K in 0 T) [Citation15]. Thus, we do not see any field effect on nonmagnetic Si in the grease at high temperature.
Measurement of 
In order to further test the setup, we measured the powder (grain size <50 μ m) of ferromagnetic shape memory alloy Ni40Co10Mn34Al16. We show only some results to demonstrate the instrument capability. Details of the experimental results and discussions will be presented elsewhere. The sample was prepared by Kainuma et al [Citation16]. They determined the phase transition temperatures by magnetization measurement in 0.05 T [Citation16]. With temperature increasing from room temperature, the reverse martensitic transition starts at 388 K (=As) and finishes at 403 K (=Af). Upon temperature decrease from 473 K, the martensitic transition starts at 385 K (=Ms) and finishes at 369 K (=Mf). The Curie temperature was determined as 429 K.
The XRD patterns of Ni40Co10Mn34Al16 at 283, 398 and 473 K in 0 T are shown in figure . Here, hklt and hklc denote the Miller indices in the tetragonal structure (martensite phase) and the cubic structure (parent phase), respectively. At 283 K, diffraction peaks of only the martensite phase were observed. At 398 K, the 110c peak of the parent phase appears. This suggests that the reverse martensitic transition had already started at 398 K. At 473 K, the diffraction peaks of the martensite phase have almost vanished, and the 110c peak is strongly enhanced. These results are consistent with those reported by Kainuma et al [Citation16]. It is well known that the phase transition temperatures of shape memory alloys are affected by external stress. However, when the powder sample is fixed with grease, the high-temperature XRD measurement is not affected.
Figure 6 X-ray diffraction patterns of Ni40 Co10 Mn34 Al16 at 283, 398 and 473 K in 0 T. hklt and hklc denote the Miller indices in the tetragonal (martensite phase) and cubic (parent phase) structures, respectively.
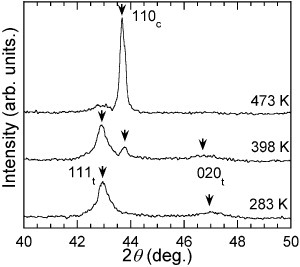
Next, the magnetic field dependence of the XRD patterns was measured. In figure , we show typical HF-XRD patterns at 408 K in 0 and 5 T. Table shows the integrated intensities of 111t and 110c in 0 and 5 T. The integrated intensities are normalized by that of the 111t reflection in 0 T. At this temperature, the martensite and parent phases are observed. In 0 T, the intensity of the 111t peak is higher than that of the 110c peak. By applying a magnetic field of 5 T, the 110c intensity was enhanced, and the intensity of the 111t peak was suppressed. This result indicates that the reverse martensitic transition was induced by applying a magnetic field. It should be noted that when the ferromagnetic powder was prepared and evenly attached using the Apiezon H grease in the high magnetic field of 5 T, the field-induced structural transformation was observed. That is, the results show that our high-temperature HF-XRD apparatus and technique are useful for materials research in high magnetic fields. In order to perform the HF-XRD measurement at above 473 K, we have developed another method of fixing powder at high temperature. In addition, we proceed to prepare a new heater for use in the HF-XRD measurement. We will present the results of HF-XRD measurements at high temperatures over 473 K in the near future.
Figure 7 X-ray diffraction patterns of Ni40Co10Mn34Al16 in 0 and 5 T at 408 K. hklt and hklc denote the Miller indices in the tetragonal (martensite phase) and cubic (parent phase) structures, respectively.
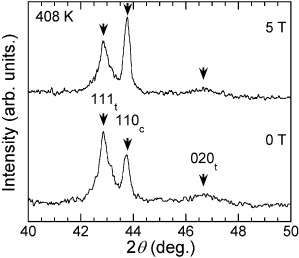
Table 1 Integrated intensities of 111t and 110c reflections in 0 T and 5 T. Integrated intensities are normalized by the intensity of 111t reflection in 0 T.
Conclusions
A HF-XRD system for use at high temperatures has been developed. We can measure the HF-XRD patterns under the experimental conditions of 0 < 2θ < 120°, 0 <B< 5 T, and 283 <T< 473 K. The stability of the temperature is within 1 K over 6 h. The powder is fixed using Apiezon H grease. The HF-XRD measurements of Si in 0 and 5 T were carried out, and the temperature dependence of the lattice parameter was obtained. The average coefficient of the thermal expansion of Si was consistent with the reported data. In addition, the HF-XRD measurement of the ferromagnetic shape memory alloy Ni40Co10Mn34Al16 was carried out in the temperature range 283–473 K. In 0 T, the reverse martensitic transformation was observed close to 398 K, which is consistent with the results of thermal analysis. Furthermore, we observed the magnetic field-induced reverse martensitic transition at 408 K.
Acknowledgments
The authors are grateful to Professor Kainuma for providing the Ni40Co10Mn34Al16 ferromagnetic shape memory alloy. This study is partially supported by the Nippon Sheet Glass Foundation for Materials Science and Engineering.
References
- GoldschmidtH JCunninghamJ 1950 J. Sci. Instrum. 27 177 http://dx.doi.org/10.1088/0950-7671/27/7/301
- SteinfinkHLadellJPostBFrankuchenI 1953 Rev. Sci. Instrum. 24 882 http://dx.doi.org/10.1063/1.1770866
- JayaramanA 1983 Rev. Mod. Phys. 55 65 http://dx.doi.org/10.1103/RevModPhys.55.65
- TajimaKSuzukiNOguchiK 1990 Japan. J. Appl. Phys. 29 986 http://dx.doi.org/10.1143/JJAP.29.986
- PecharskyV KGschneidnerK AJr 2004 Rev. Sci. Instrum. 75 4
- WatanabeKWatanabeYAwajiSFujiwaraMKobayashiNHasebeT 1998 Adv. Cryo. Eng. 44 747
- PecharskyV KGschneidnerK AJr 1997 Phys. Rev. Lett. 78 4494 http://dx.doi.org/10.1103/PhysRevLett.78.4494
- TegusOBrückEBuschowK H Jde BoerF R 2002 Nature 415 150 http://dx.doi.org/10.1038/415150a
- WadaHTanabeY 2001 Appl. Phys. Lett. 79 3302 http://dx.doi.org/10.1063/1.1419048
- FujitaAFujiedaSFukamichiKYamazakiYIijimaY 2002 Mater. Trans. 43 1202 http://dx.doi.org/10.2320/matertrans.43.1202
- UllakkoKHuangJ KKantnerCO'HandleyR C 1996 Appl. Phys. Lett. 69 1966 http://dx.doi.org/10.1063/1.117637
- KainumaR et al 2006 Nature 439 957 http://dx.doi.org/10.1038/nature04493
- IshikawaFKoyamaKWatanabeKAsanoTWadaH 2006 J. Phys. Soc. Japan 75 084604 http://dx.doi.org/10.1143/JPSJ.75.084604
- KoyamaKOkadaHWatanabeKKanomataTKainumaRItoWOikawaKIshidaK 2006 Appl. Phys. Lett. 89 182510 http://dx.doi.org/10.1063/1.2374868
- YimW MPaffR J 1973 J. Appl. Phys. 45 1456 http://dx.doi.org/10.1063/1.1663432
- KainumaRUmetsuRItoWOikawaIshidaK 2008 Appl. Phys. Lett. 93 091906 http://dx.doi.org/10.1063/1.2965811