Abstract
An environment-friendly hydrothermal method was used to prepare TiO2@C core–shell composite using TiO2 as core and sucrose as carbon source. TiO2@C served as a support for the immobilization of Ag by impregnation in silver nitrate aqueous solution. The chemical structures and morphologies of TiO2@C and TiO2@C/Ag composite were characterized by x-ray diffraction, transmission electron microscopy, Fourier transform infrared spectroscopy, energy dispersive x-ray spectroscopy and Brunauer–Emmett–Teller (BET) analysis. The antibacterial properties of the TiO2@C/Ag core–shell composite against Escherichia coli (E. coli) and Staphylococcus aureus (S. aureus) were examined by the viable cell counting method. The results indicate that silver supported on the surface of TiO2@C shows excellent antibacterial activity.
Introduction
Carbon materials have excellent chemical, mechanical and thermal stabilities and are therefore suitable for various applications [Citation1–4]. Novel carbon materials as supports for antibacterial composites have been produced recently [Citation1, Citation4]. Zhang et al [Citation1] prepared silver-dispersed carbon aerogels (CAs) by direct immersion of organic aerogels in AgNO3 aqueous solution followed by carbonization. Ag-dispersed CAs exhibit strong long-term antibacterial activity. Carbon monolith samples with a silver coating showed good antimicrobial activity against Escherichia coli, Staphylococcus aureus and Candida albicans [Citation4]. Because of its specific shape, low silver attrition rate and good antibacterial activity, the impregnated monolith can be utilized as a personal disposable filter. Silver composites as antibacterial materials have become a hot research topic owing to the high efficiency and stability as well as broad spectrum of antimicrobial activities of silver [Citation1, Citation4–7].
Nanomaterials with core–shell structure have been extensively studied because of their multifunctional properties and various applications, such as drug delivery [Citation8] and increasing their solubility and stability [Citation9, Citation10] and altering the emission properties of quantum dots [Citation11, Citation12]. In addition to the above-mentioned characteristics, core–shell nano-oxides such as TiO2@X and ZnO@X also have unique antibacterial properties that have attracted attention to this area in recent years [Citation13–16]. It was found that the antibacterial activity of ZnO@C core–shell powders increases with increasing amount of ZnO and decreases with increasing carbonization temperature. The antibacterial activity towards S. aureus was found to be stronger than that towards E. coli. No activity of the carbon samples without ZnO was observed. The antibacterial activity was attributed to the generation of hydrogen peroxide from ZnO in core–shell powders [Citation13]. Apatite-coated TiO2 was also prepared and fixed on cotton textiles by dip coating, and the antimicrobial properties of the produced fabrics were then investigated [Citation14]. The findings suggest that the presence of apatite-coated TiO2 induces antibacterial activity in the presence of black light or visible light, suggesting a potential use in reducing the risk of microorganism transmission for textile application. However, the applications of the core–shell nano-oxides were limited owing to their relatively poor antibacterial activity. This activity can possibly be improved using silver ions. TiO2@C core–shell composite has wide applications in photocatalysts [Citation17, Citation18] and anode materials of lithium batteries [Citation19] owing to the excellent performance of TiO2 and the unique structure of carbon. It also possesses some antibacterial activity and can be used as a carrier for antibacterial materials. However, to the best of our knowledge, there have been few reports on its antibacterial activity.
TiO2 has three crystalline phases: anatase, rutile and brookite. Thermodynamically, rutile is the most stable form. Anatase has attracted much attention owing to its application in photovoltaic cells [Citation20] and photocatalysts [Citation21] and for its antimicrobial properties [Citation22]. In this study, we synthesized TiO2@C core–shell composite using TiO2 as the core and sucrose carbonization as the shell via a simple hydrothermal method. Sucrose is a cheap and environment-friendly carbon precursor [Citation23]. Silver-supported TiO2@C core–shell composite was obtained by direct immersion of TiO2@C in AgNO3 aqueous solution. The chemical structures and morphologies of TiO2@C and TiO2@C/Ag composite were investigated, and the antibacterial activities of TiO2@C/Ag composite were examined by the viable cell counting method.
Experimental details
Synthesis of TiO2@C/Ag core–shell composite
All chemical reagents were of analytical-grade purity and used as received. A certain amount of commercial TiO2 (P25, Degussa, Germany) and 15.0 g of sucrose were dispersed in 40 ml of distilled water, then added to a 60-ml stainless steel autoclave. The autoclave was maintained at 600 °C for 16 h to complete the reaction and then allowed to cool to room temperature. A dark precipitate was collected and washed with distilled water and absolute ethanol, and then dried in vacuum at 60 °C for 6 h. To deposit silver on the TiO2@C surface, we used the synthesized TiO2@C as a support for the immobilization of silver. Silver was deposited by impregnation in the AgNO3 aqueous solution with different concentrations for 15 min under ultrasonic agitation. TiO2@C containing silver is labeled here as TiO2@C/Ag.
Characterization of TiO2@C and TiO2@C/Ag
The structure of as-prepared samples was analyzed with an MSAL-XD2 x-ray diffractometer (CuKα, 40 kV, 20 mA, λ=1.5406 Å). The morphologies were observed using a Philips Tecnai-10 transmission electron microscope (TEM) at an accelerating voltage of 100 kV. The functional groups on the surface of TiO2@C were detected through Fourier transform infrared (FTIR) absorption spectra recorded with a Nicolet 6700 spectrometer. The specific surface area was measured using a Micromeritics TriStar 3000 analyzer. The chemical composition of the as-prepared TiO2@C/Ag composite was analyzed with an Oxford Inca 350 energy dispersive x-ray spectroscope (EDS).
TiO2@C/Ag for tests of antibacterial activity
For antibacterial tests, E. coli (ATCC25922) and S. aureus (ATCC6538) were selected as indicators, following the method of viable cell counts [Citation24, Citation25]. All glassware and materials were sterilized in an autoclave at 120 °C for 25 min prior to the experiments. A set of test tubes containing 0.1 mg (against E. coli) or 0.2 mg (against S. aureus) of the samples were weighed. Each sample was dispersed in 10 ml of the 0.9 wt.% saline water containing about 105 colony forming units (cfu) ml−1 of E. coli or S. aureus, and then shaken at 37 °C for 6 h. One milliliter of the treated solution was obtained from the test tube at the prescribed time, centrifuged and diluted with distilled water to a certain volume. To ensure that the grown bacterial colonies could be counted easily and correctly, tenfold dilution was applied. The final diluted solution was plated on agar in triplicate, and then the obtained plates were cultivated at 37 °C for 24 h. The number of bacterial colonies on each plate was counted and the obtained values were averaged to obtain the final data.
Results and discussion
Characterization of structure and morphology
Commercial TiO2 powder with the particle diameter of 30–40 nm was used as a core in our experiment. Reaction time and temperature are critical for the preparation of carbon materials. The reaction system was maintained at 500–700 °C for 12–20 h and an optimized synthesis condition was identified at 600 °C for 16 h. The as-prepared products under the optimum synthesis condition were characterized by x-ray diffraction (XRD). Figure (a) shows the XRD patterns of P25 and TiO2@C samples synthesized under different gravimetric ratios of sucrose/TiO2, illustrating that P25 and TiO2@C series products are composed of anatase and rutile. It is well known that Degussa P25 is a mixture of anatase (70%) and rutile (30%) and has a surface area of 50 m2 g−1 [Citation26]. The two phases of TiO2 were confirmed from the XRD pattern shown in figure (a), curve 1. The intensity of anatase peaks in all TiO2@C samples was lower than that in the case of P25. This indicates that some anatase transformed into rutile upon calcination. Furthermore, the anatase peaks strengthened and the rutile peaks weakened with increasing ratio of sucrose/TiO2, indicating that the carbon layer suppressed the phase transformation from anatase to rutile. With the increase in the sucrose/TiO2 ratio, the carbon shell became thicker and the anatase TiO2 phase became more stable. It is known that the phase transformation of anatase to rutile occurs at approximately 600 °C [Citation27], but by coating anatase TiO2 with carbon, the temperature of crystal transformation becomes higher. Similar results were reported by Tsumura et al [Citation28] and Shanmugam et al [Citation17]. Figure (b) shows the XRD patterns of TiO2@C/Ag prepared at different concentrations of AgNO3 aqueous solution. TiO2@C (sucrose/TiO2=28) was immersed in 0.01, 0.02 and 0.05 mol l−1AgNO3 solution for 15 min under ultrasonic agitation. The thus produced samples were denoted as TCA-1, TCA-2 and TCA-3 (T from TiO2, C-carbon and A-Ag), respectively. The weak diffraction peaks of silver crystal in curves 2–4 of figure (b) indicate that a redox reaction between Ag+ and reductive carbon occurred during the adsorption [Citation1]. Three characteristic peaks are observed at 2θ values of 38.1°, 44.2° and 64.5°, respectively, corresponding to the (111), (200) and (220) crystalline planes of Ag crystal. As shown in the XRD pattern, TiO2 is mainly present in the form of anatase existing in the TiO2@C sample (sucrose@TiO2=28/1) and all the TiO2@C/Ag samples.
Figure 1 (a) XRD patterns of P25 and the as-prepared samples under different gravimetric ratios of sucrose/TiO2 at 600 °C for 16 h. (1: P25; 2: 10/1; 3: 19/1; 4: 28/1); (b) XRD patterns of TiO2@C and TiO2@C immersed in different concentrations of AgNO3 solution, 1: TiO2@C; 2: 0.01 mol l−1 (TCA-1); 3: 0.02 mol l−1 (TCA-2); 4: 0.05 mol l−1 (TCA-3).
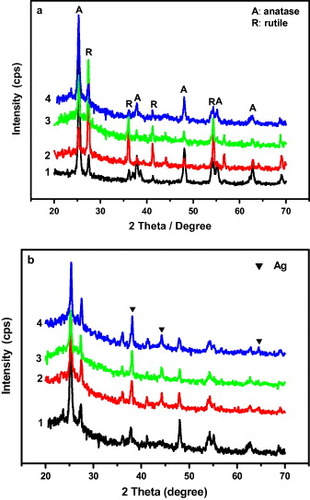
The amount of deposited silver depends on the initial concentration of Ag+ as well as the surface area, pore structure and quantity of acidic functional groups that can act as initial active sites for adsorption [Citation4, Citation29]. Yue et al [Citation29] explained that there are two types of reaction that might occur during Ag+ adsorption: (i) an ion-exchange reaction between silver ions and surface carboxylic groups and (ii) redox adsorption, which is confirmed by XRD. The XRD patterns indicate that some silver ions were reduced to silver crystals.
Figure shows TEM images of the TiO2@C and TCA-3 samples obtained under the optimum synthesis condition. Figure (a) is a low-magnification TEM image of TiO2@C (sucrose/TiO2=28); it reveals TiO2 nanoparticles wrapped in a carbon film. A core–shell TiO2@C structure is shown in detail in a high-magnification TEM image (figure (b)); the thickness of the carbon shell is approximately 20 nm. Figure (c) shows a high-magnification TEM image of TCA-3. The silver particles in TCA-3 are round and are dispersed separately on the surface of the carbon shell.
Figure 2 Low-magnification (a) and high-magnification, (b) TEM images of TiO2@C and (c) TEM image of TCA-3.
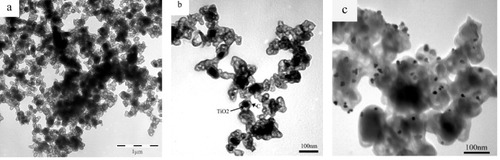
Figure shows the isotherms of the nitrogen adsorption–desorption measurements of the TiO2@C and TCA-3 samples. By the Brunauer–Deming–Deming-Teller (BDDT) method, both of them were found to yield a type-I nitrogen isotherm with an H4 hysteresis loop, which indicates a micropore structure and single-molecule chemical adsorption [Citation30]. Micropores in the sample are mainly distributed on the surface of the carbon film. However, in the desorption branch, a clear hysteresis loop at the relative pressure of 0.45 indicates the presence of mesopores. Mesoporous structure is also supported by TEM observations. These results illustrate that the pore structure in TiO2@C and TCA-3 samples consists of micropores and mesopores. The surface areas, pore diameters and pore volumes were calculated from the isotherms and are summarized in table . The pore size distributions were measured on the basis of the Barrett–Joyner–Halenda (BJH) adsorption isotherms. The SBET surface areas of TiO2@C and TCA-3 samples are higher than that of P25. This result indicates that coating TiO2 with carbon can considerably increase the surface area of pure TiO2. Thus, the TiO2@C core–shell structure is advantageous for loading silver. Compared with those of the TiO2@C sample, the SBET and Vmic of the TCA-3 sample were markedly lower. This is due to the blockage of pores by silver particles, as shown in figure (c). The result is similar to that reported for Ag/CAs [Citation1].
Table 1 Surface and pore characteristics of TiO2@C and TCA-3.
The functional groups of the TiO2 and TiO2@C materials were characterized by FTIR transmittance, as shown in figure . Figure (a) shows a broad band around 3440 cm–1 and another around 1640 cm−1, which are attributed to the surface-adsorbed H2O and the group of TiO2 [Citation31]. A broad band in the range of 400–900 cm−1 is ascribed to the stretching vibration of the
bond. The IR spectrum of TiO2@C dried under vacuum is shown in figure (b). The strong characteristic peak at 3434 cm−1 is assigned to the stretching vibration of the
bond at the carbon surface, and the peaks at 2919 and 2847 cm−1 originate from the stretching vibration of
bonds. The peak at 1389 cm−1 corresponds to the
stretching [Citation32]. Compared with that shown in figure (a), the additional peak at 1740 cm−1 is due to the stretching vibration of the carboxyl group
. The weakening of the peak at around 1640 cm−1 indicates the loss of H2O adsorbed on the TiO2@C surface under vacuum. Furthermore, owing to the carbon shell on the TiO2 core, the stretching vibration of the
bond becomes visibly weak. The FTIR spectrum reveals many functional groups on the surface of the TiO2@C composite. The retained functional groups provide a potential avenue to load other functional groups, molecules, ions or nanoparticles [Citation33]. In our experiment, sucrose acts not only as a cheap and environment-friendly carbon source, but also retains many hydrophilic functional groups, such as
and
, on the carbon surface after calcination.
The elemental content in TCA samples was measured by EDS. The loadings of silver onto TCA-1, TCA-2 and TCA-3 samples were 0.81, 1.52 and 1.72 wt.%, respectively. The silver content in the TiO2@C composite increased with the increase in the initial AgNO3 concentration.
Antibacterial properties
TiO2@C/Ag could be a good antibacterial material. Table lists the antibacterial activity of different samples against E. coli and S. aureus after 6 h of contact. The sample concentration was 10.0 mg l−1 against E. coli and 20.0 mg l−1 against S. aureus. The commercial carbon black (C) has no antibacterial activity because of the increase in the number of viable bacterial colonies after 6 h of contact. TiO2@C exhibits weak antibacterial activity as shown in the decrease in the number of viable bacterial colonies, which could be attributed to the antimicrobial properties of TiO2. TiO2, particularly anatase TiO2, has an excellent photocatalytic performance. It can generate strong oxidizing power under certain conditions. After carbon-coated TiO2 suspensions are irradiated and activated, reactive oxygen species such as OH, O2−, and H2O2 might be generated on the irradiated TiO2 surface; those species might attack and decompose polyunsaturated phospholipids in bacteria [Citation34]. However, three materials, TiO2@C/Ag (TCA-1, TCA-2 and TCA-3), showed excellent antibacterial activity, and the number of viable colonies of bacteria visibly decreased. It is clear that the antibacterial activity increased with the increase in silver content. As for TCA-2 and TCA-3 samples, all the E. coli and more than 99.99% of the S. aureus bacteria were killed within 6 h of contact. Moreover, TCAs exhibit higher activity against E. coli than against S. aureus under the same test conditions.
Table 2 Antibacterial activity of the samplesa.
Silver ions are highly toxic to microorganisms and have a strong biocidal effect on many bacterial species. Silver nanoparticles also have inhibitory and bactericidal effects [Citation35]. However, the actual bactericidal mechanism of silver nanoparticles is yet unknown. Some researchers support the idea that silver species release Ag+ ions, which interact with the thiol groups in bacterial proteins, affecting the replication of DNA [Citation36]. In our experiment, there are two forms of silver existing in the samples of TCAs. One is silver ion obtained by an ion-exchange reaction, the other is silver metal that is formed by a redox adsorption. Therefore, it could be concluded that silver particles in the TiO2@C composite were gradually oxidized into silver oxide and then released silver ions into the solution [Citation37]. It may be reasonably presumed that such pore structures and functional groups of the TiO2@C composite will prolong the release time of Ag+ ions and preserve the sustained antibacterial behavior. Because the cell wall is thicker in S. aureus than in E. coli [Citation38], the antibacterial efficiency of TCAs is lower for S. aureus than for E. coli.
Conclusions
TiO2@C core–shell composite was successfully synthesized via a simple hydrothermal route. TiO2@C/Ag composite obtained by impregnation of TiO2@C in AgNO3 aqueous solution was used to kill E. coli and S. aureus. The silver content of the TiO2@C material can be controlled by adjusting the initial AgNO3 concentration. The characterization by TEM, BET and FTIR techniques shows that TiO2@C is an excellent carrier for the loading and adsorption of silver on the surface owing to the carbon layer that has a high BET surface area, porous structure and hydrophilic functional groups. TiO2@C/Ag displays excellent antibacterial activity that increases with increasing silver content of the samples. Furthermore, the antibacterial activity towards E. coli was stronger than that towards S. aureus. Such core–shell structure composite could have promising applications as antibacterial materials for microbiocides and water treatment.
Acknowledgments
This work is financially supported by the National Natural Science Foundation of China (Grant Nos. 20676049 and 20876067), Foundation of Enterprise-University-Research Institute Cooperation of Guangdong Province, Ministry of Education of China (Grant No. 2007B090400105) and Young Teacher Fund of Jinan University (51208023).
References
- ZhangS TFuR WWuD CXuWYeQ WChenZ L 2004 Carbon 42 3209 http://dx.doi.org/10.1016/j.carbon.2004.08.004
- YuanD STanS ZLiuY LZengJ HHuF PWangXShenP K 2008 Carbon 46 531 http://dx.doi.org/10.1016/j.carbon.2008.01.004
- XiaoS JLiY FHuangC Z 2007 Chem. Lett. 36 798 http://dx.doi.org/10.1246/cl.2007.798
- VukčevićMKalijadisADimitrijević-BrankovićSLauševićZLauševićM 2008 Sci. Technol. Adv. Mater. 9 015006 http://dx.doi.org/10.1088/1468-6996/9/1/015006
- ZhangS TWuD CWanLTanH BFuR W 2006 J. Appl. Polym. Sci. 102 1030 http://dx.doi.org/10.1002/app.24124
- DammCMünstedtHRöschA 2008 Mater. Chem. Phys. 108 61 http://dx.doi.org/10.1016/j.matchemphys.2007.09.002
- XuKWangJ XKangX LChenJ F 2009 Mater. Lett. 63 31 http://dx.doi.org/10.1016/j.matlet.2008.08.039
- ZhuYShiJShenWDongXFengJRuanMLiY 2005 Angew. Chem., Int. Ed. Engl. 44 5083 http://dx.doi.org/10.1002/anie.200501500
- ChanYZimmerJ PStrohMSteckelJ SJainR KBawendiM G 2004 Adv. Mater. 16 2092 http://dx.doi.org/10.1002/adma.200400237
- LiuSZhangZHanM 2005 Anal. Chem. 77 2595 http://dx.doi.org/10.1021/ac0482864
- FleischhakerFZentelR 2005 Chem. Mater. 17 1346 http://dx.doi.org/10.1021/cm0481022
- SongJ HAtayTShiSUrabeHNurmikkoA V 2005 Nano Lett. 5 1557 http://dx.doi.org/10.1021/nl050813r
- YamamotoONakakoshiKSasamotoTNakagawaHMiuraK 2001 Carbon 39 1643 http://dx.doi.org/10.1016/S0008-6223(00)00289-X
- KangwansupamonkonWLauruengtanaVSurassmoSRuktanonchaiU 2009 Nanomed. Nanotechnol. Biol. Med. 5 240 http://dx.doi.org/10.1016/j.nano.2008.09.004
- WangH et al 2009 J. Mater. Sci.: Mater. Med. 20 11 http://dx.doi.org/10.1007/s10856-008-3541-z
- ChenW JTsaiP JChenY C 2008 Small 4 485 http://dx.doi.org/10.1002/smll.200701164
- ShanmugamSGabashviliAJacobD SYuJ CGedankenA 2006 Chem. Mater. 18 2275 http://dx.doi.org/10.1021/cm052790n
- ZhangL XLiuPSuZ X 2006 J. Mol. Catal. A: Chem. 248 189 http://dx.doi.org/10.1016/j.molcata.2005.12.039
- FuL JLiuHZhangH PLiCZhangTWuY PWuH Q 2006 J. Power Sources 159 219 http://dx.doi.org/10.1016/j.jpowsour.2006.04.081
- FujishimaARaoT NTrykD A 2000 J. Photochem. Photobiol. C 1 1 http://dx.doi.org/10.1016/S1389-5567(00)00002-2
- GratzelM 2001 Nature 414 338 http://dx.doi.org/10.1038/35104607
- FuGVaryP SLinC-T 2005 J. Phys. Chem. B 109 8889 http://dx.doi.org/10.1021/jp0502196
- YuanD SChenJ XZengJ HTanS X 2008 Electrochem. Commun. 10 1067 http://dx.doi.org/10.1016/j.elecom.2008.05.015
- TanS ZOuyangY SZhangL LChenY BLiuY L 2008 Mater. Lett. 62 2122 http://dx.doi.org/10.1016/j.matlet.2007.11.030
- GarzaM ROlguínM TSosaI GAlcántaraDFuentesG R 2000 Micropor. Mesopor. Mater. 39 431 http://dx.doi.org/10.1016/S1387-1811(00)00217-1
- 1990 Degussa Technical Bulletin Pigment Report vol 56 p 13
- InagakiMHiroseYMatsunagaTTsumuraTToyodaM 2003 Carbon 41 2619 http://dx.doi.org/10.1016/S0008-6223(03)00340-3
- TsumuraTKojitaniNIzumuIIwashitaNToyodaMInagakiM 2002 J. Mater. Chem. 12 1391 http://dx.doi.org/10.1039/b201942f
- YueZ RJiangWWangLToghianiHGardnerS DPittmanC U Jr 1999 Carbon 37 1607 http://dx.doi.org/10.1016/S0008-6223(99)00041-X
- SingK S WEverettD HHaulR A WMoscouLPierottiR ARouquérolJSiemieniewskaT 1985 Pure Appl. Chem. 57 603 http://dx.doi.org/10.1351/pac198557040603
- DingZLuG QGreenfieldP F 2000 J. Phys. Chem. B 104 4815 http://dx.doi.org/10.1021/jp993819b
- ChengC HLehmannJThiesJ EBurtonS DEngelhardM H 2006 Org. Geochem. 37 1477 http://dx.doi.org/10.1016/j.orggeochem.2006.06.022
- BondA MMiaoW JRastonC L 2000 Langmuir 16 6004 http://dx.doi.org/10.1021/la991699t
- ManessP CSmolinskiSBlakeD MHuangZWolfrumE JJacobyW A 1999 Appl. Environ. Microbiol. 65 4094
- ShrivastavaSBeraTRoyASinghGRamachandraraoPDashD 2007 Nanotechnology 18 225103 http://dx.doi.org/10.1088/0957-4484/18/22/225103
- MariniMDe NiederhausernNIseppiRBondiMSabiaCToselliMPilatiF 2007 Biomacromolecules 8 1246 http://dx.doi.org/10.1021/bm060721b
- BakerCPradhanAPakstisLPochan-DarrinJShahS I 2005 J. Nanosci. Nanotechnol. 5 244 http://dx.doi.org/10.1166/jnn.2005.034
- ZhaoD FZhouJLiuN 2006 Appl. Clay Sci. 33 161 http://dx.doi.org/10.1016/j.clay.2006.04.003