Abstract
Various inorganic nanoparticles have been used for drug delivery, magnetic resonance and fluorescence imaging, and cell targeting owing to their unique properties, such as large surface area and efficient contrasting effect. In this review, we focus on the surface functionalization of inorganic nanoparticles via immobilization of biomolecules and the corresponding surface interactions with biocomponents. Applications of surface-modified inorganic nanoparticles in biomedical fields are also outlined.
Introduction
Progress in utilizing inorganic nanoparticles for biomedical applications has advanced rapidly owing to extensive research on the synthesis and modification of materials [Citation1–6]. These inorganic nanoparticles provide a robust framework, in which two or more components can be incorporated to provide multifunctional capabilities. Biomacromolecules, such as proteins, lipids and polysaccharides, fluorescent molecules and anticancer drugs, can be conjugated to the surface of inorganic nanoparticles. The resulting nanoparticles can not only target human cancers, but also be visualized inside the body by both magnetic resonance (MR) and fluorescence imaging [Citation7, Citation8]. The properties of the inorganic nanoparticles are very different from those of their bulk counterparts for two main reasons: the increased relative surface area and the quantum confinement effects. The marked increase in the surface area also increases the nanoparticles’ chemical reactivity and ability to interact with added functional materials.
For many biomedical applications, particularly for in vivo imaging, inorganic nanoparticles possess good colloidal stability and low toxicity in a biological environment. Although high-quality nanoparticles with uniform size and high crystallinity have been synthesized, they are hydrophobic, and consequently, are not sufficiently stable in aqueous media for successful biomedical applications [Citation9–17]. Therefore, the surface modification of these inorganic nanoparticles is essential to endow them with hydrophilic properties, so that they can be extensively used for various biomedical applications [Citation18, Citation19]. Furthermore, surface modification is important for the nanoparticles to impart additional functions, because bioactive materials are conjugated through the reactive groups on the nanoparticle surface. Recently, various surface modification methods have been developed; two representative strategies are ligand exchange with water-dispersible ligands and encapsulation with biocompatible shells. Selim et al [Citation20] were able to form water-dispersible iron oxide nanoparticles by a silanization reaction using 3-aminopropyl triethoxysilane (APTES). The resulting iron oxide nanoparticles were dispersible in water and successfully used for in vivo MRI. Ying and co-workers reported on silica-coated nanoparticles produced by base-catalyzed silica formation in a reverse microemulsion [Citation21, Citation22]. More recently, an additional biocompatible polymer (e.g. polyethylene glycol, PEG) has been impregnated onto the surface of the silica shell to improve colloidal stability [Citation23].
In this article, we summarized the immobilization of biomolecules such as lactobionic acid, peptide and DNA, on the surface of inorganic nanoparticles. We also reviewed their interaction with cells or other biocomponents for MR imaging, drug delivery and targeting of cancer cells.
Inorganic nanoparticles
The materials used for biomedical applications include calcium nanoparticles, iron nanoparticles, carbon nanotubes, double hydroxides/clays, silica, calcium phosphate and quantum dots. Although inorganic nanoparticles show only moderate transfection efficiencies, they possess some advantages over organic nanoparticles. They are not subject to microbial attack, can be easily prepared, often have a low toxicity, and exhibit good storage stability.
Metallic nanoparticles
The chemistry of metallic nanoparticles is well studied, particularly with respect to nanoparticles of the noble metals such as gold, silver, palladium and platinum [Citation24]. Usually, they are prepared by reduction of the corresponding metal salts in the presence of a suitable protecting group that prevents further aggregation [Citation25].
Gold nanoparticles (AuNPs, typical size: 10–20 nm) are easily taken up by cells [Citation26–29]. Huang et al have shown recently [Citation30] that AuNPs are useful for the identification of some types of cancer cells. Plate-derived growth factor induced the aggregation of Apt-AuNPs (nucleic acid ligand; Aptamer, Apt). This aggregation strongly increased the scattering of light, with a color different from that scattered from the original Apt-AuNPs and cell organelles. Salmaso et al [Citation31] reported on AuNPs that were surface-coated with thermoresponsive thiol-terminated poly-N-isopropylacrylamide-co-acrylamide copolymer. The cell uptake of polymer-coated AuNPs can be temperature-controlled; this opens new perspectives for in vivo testing aimed at thermally controlled targeting of tumors or inflamed tissues. Salem et al reported on bimetallic nanorods consisting of gold and nickel as a non-viral gene delivery system [Citation32]. Tkachenko et al [Citation33] studied the pathway of gold-peptide nanoparticles inside cells.
Iron oxides
Iron oxide nanoparticles having magnetic properties can be used for cell sorting, magnetic guidance in the body and tumor thermotherapy [Citation34–37]. If the particles are subjected to a rapidly changing magnetic field, they can destroy tumor tissue by hyperthermia [Citation35, Citation38]. Another approach is the magnetic guidance of a particle to a selected part of the body, for example, into a tumor.
Selim et al [Citation20] reported on iron oxide particles with an average diameter of 10 nm. When modified with lactobionic acid, these nanoparticles were selectively accumulated in hepatocytes, as confirmed by MR imaging. Zhang et al [Citation39] investigated the different cellular uptake behaviors of tumor-homing F3 peptide-conjugated iron oxide nanoparticles. Their experimental results showed a distinct pattern of Zeta potential change that allows the discrimination of normal human breast epithelial cells from breast cancer epithelial cells, where the tumor-homing F3 peptide was specifically bound to nucleolin receptors that are overexpressed in breast cancer cells. Cengelli et al [Citation40] presented the linkage of therapeutic drugs to iron oxide nanoparticles, allowing the intracellular release of the active drug via cell-specific mechanisms. The drug-conjugated nanoparticles exhibited antiproliferative activity in vitro against human melanoma cells and resulted in tumor-selective and magnetically enhanced drug delivery.
Carbon nanotubes
Following the discovery of carbon nanotubes (CNTs) by Iijima in 1991 [Citation41], CNTs were the subject of many studies because of their special structural, mechanical, electrical and chemical properties. CNTs can be classified into single-walled carbon nanotubes (SWCNTs) and multiwalled carbon nanotubes (MWCNTs) [Citation42], with diameters of close to 1 nm and lengths up to 1 mm [Citation43, Citation44]. They have been applied as composite materials [Citation45], nanoelectronic components [Citation46, Citation47], field-effect emitters [Citation48] and hydrogen storage materials [Citation49]. The biological applications of CNTs have also been explored recently [Citation50–53]. CNT-based sensors have been developed to detect biological species including proteins and DNA [Citation50, Citation54, Citation55]. By relying on their optical properties, SWCNTs can be utilized as optical tags or contrast agents for various biological imaging techniques [Citation53, Citation56–58].
Double hydroxides/clays
Layered double hydroxides (LDHs; also known as anionic clays or hydrotalcites) constitute a class of clays that contain positively charged layers. Interlayer anions and water molecules are present in the interlayer space and can be replaced by other molecules [Citation59–61]. LDHs with high anion exchange capacity have attracted particular attention in the field of bio-hybrid nanomaterials owing to their high biocompatibility, high chemical stability and controlled release rate. Choy et al [Citation62] reported that adenosine 5′-triphosphate (ATP)-intercalated LDH resulted in a high transfer efficiency of ATP into target cells, by decreasing the electrical repulsion at the cell walls. The high transfer efficiency originated from the neutralization of the negative charge of the phosphates with the positive hydroxide layers. Choy et al [Citation63] also showed that folinic acid and methotrexate (MTX) can be successfully hybridized with LDH by ion-exchange reactions. Furthermore, the MTX–LDH hybrid is more efficient in suppressing tumor cells. Nalawade et al reviewed the use of LDH for tissue engineering, and concluded with the following sentences that highlight the present state and possible problems [Citation64]: ‘Hybridization of a drug or a biomolecule with LDH results in high transfer efficiency and stability. Thus, LDH hybrids can be useful as reservoirs and carriers of genes, drugs, and other functional molecules’.
Silica
The preparation of silica nanoparticles by suitable sol–gel processing routes is well established [Citation65]. The presence of silanol groups on the surface allows easy functionalization, for example, by attaching functionalized chlorosilanes. This property, together with the high biocompatibility of silica, has inspired many researchers to use silica nanoparticles as carriers for drug release or transfection. Probe oligonucleotides were covalently immobilized on the surface of silica nanoparticles for the detection of DNA hybridization [Citation66]. Surface modification of silica nanoparticles leads to a strong interaction toward probe oligonucleotides; hence, it offers enhanced detection of DNA hybridization. Qian et al [Citation67] investigated biomolecule-conjugated silica nanoparticles as optical probes for cancer cell imaging. The results of the in vitro experiments revealed that apo-transferrin and folic acid functionalized nanoparticles can serve as effective optical probes for the specific targeting of cancer cells. The nanoparticles were further co-conjugated with PEG and apo-transferrin. These conjugates were also very good for in vitro targeting of cancer cells. Knop et al [Citation68] reviewed the bioanalytical applications of silica particles and concluded that the biomolecule-functionalized silica nanoparticles will become increasingly accepted as labeling reagents in bioanalysis and bioimaging because of their excellent intrinsic optical and chemical properties.
Calcium phosphate
Calcium phosphates are the inorganic components of biological hard tissues, such as, bone, teeth and tendons, where they occur as carbonated hydroxyapatite. With the exception of enamel, they are always found as nanoparticles [Citation69–71]. Sokolova et al [Citation72] reported that DNA-coated calcium phosphate nanoparticles could be stored for weeks without loss of their transfection efficiency. The DNA is still intact in the single- and multishell particles as shown by the high transfection efficiency. The accumulation of DNA-loaded calcium phosphate nanoparticles inside a cell and its nucleus was observed by fluorescence microscopy [Citation73]; the particles contained red-fluorescing tetramethylrhodamin isothiocyanate (TRITC)-bovine serum albumin (BSA). Neumann et al [Citation74] reported that cells were able to cope with the associated calcium uptake after transfection with size-defined DNA-functionalized calcium nanoparticles, and therefore proved that the calcium phosphate-based transfection method was superior.
Quantum dots
Quantum dots (QD) are small nanoparticles of II–VI or III–V semiconductors (e.g. CdS, CdSe, ZnS, ZnSe, ZnO, GaAs, InAs; sometimes in a core–shell structure) with typical diameters of a few nanometers [Citation75]. The QDs are protected against aggregation by suitable capping agents that can also be functionalized. They show favorable optical properties (highly efficient fluorescence owing to quantum confinement effects and good resistance towards photobleaching) that are exploited, for example, for biomedical imaging [Citation76, Citation77]. Zhou and Ghosh [Citation78] reviewed the incorporation of cell-penetrating peptides on QD surfaces that allowed for the translocation of functionalized QDs into cells for intracellular imaging applications. Moreover, QD-labeled peptides that serve as ligands for cellular receptors provide an alternative to antibody mediated imaging at the whole-cell and single-molecule levels for studying receptor distribution and trafficking. Yong et al [Citation79] reported that the indium phosphide (core)/zinc sulfide (ZnS) (InP/ZnS) QDs not only offer insights into the receptor mediated delivery mechanism but also help the design and development of future nanoprobes for in vivo cancer detection and therapeutic applications. These QDs have emerged as an alternative to organic dyes and fluorescent proteins, and are brighter and more stable against photobleaching than standard fluorescent indicators. QDs have tunable optical properties that have proved useful in a wide range of applications from multiplexed analysis, such as DNA detection and cell sorting and tracking, to the most recently demonstrated potential for in vivo imaging and diagnostics [Citation80].
Biomolecule immobilization
Many researchers have focused on the immobilization of inorganic nanoparticles with biomolecules such as lipids, saccharides, peptides, proteins and nucleic acids. These surface-modified nanoparticles have been examined for their uses as new tools not only for the investigation of biological processes but also for sensing and treating diseases. Here, we review a few immobilization processes and show the reaction paths for a better understanding of biomolecule immobilization systems.
Immobilization of thermoresponsive polymer on gold nanoparticles [Citation31]
Synthesis of PNIPAm-co-Am-SH.
PNIPAm-co-Am-COOH (97.3 mg, 16.2 μmol) was dissolved in 0.75 ml of anhydrous DMSO and N-hydroxysuccinimide (HOSu) (19.7 mg, 171 μmol) and dicyclohexylcarbodiimide (DCC) (29 mg, 140.7 μmol) were added (figure ). The reaction mixture was stirred overnight, filtered through a sintered funnel and dropped into cold diethyl ether (15 ml). The precipitate was washed with cold ether (5×10 ml) and finally desiccated under vacuum. The degree of polymer activation (PNIPAm-co-Am-COOSu/PNIPAm-co-Am-COOH molar ratio, %) was 93.6%.
Figure 1 Schematic showing the synthesis of the thermoresponsive polymer with thiol end group (PNIPAm-co-Am-SH) (reproduced with permission from [Citation31], ©2009, The Royal Society of Chemistry).

PNIPAm-co-Am-OSu (90 mg, 15 μmol) was added to 1.15 ml of 50 mM cysteine solution in 50 mM phosphate buffer, 10 mM EDTA, pH 8.0 (figure ). After 2 h of stirring at room temperature, 100 ml of 2 M dithiothreitol (DTT) in the same buffer was added to the solution, which was then maintained under stirring overnight. The pH of the mixture was adjusted to 3 by adding HCl, and the solution was purified by size exclusion chromatography using a 50 cm-long Sephadex G25 superfine prepacked column eluted with 1 mM HCl. The dry product was dissolved in water, and the polymer concentration and activation were determined as above. The degree of polymer activation (PNIPAm-co-Am-SH/PNIPAmco-Am-COOH molar ratio, %) was 68.7%. The final product recovery yield was 92% (w/w).
Preparation of PNIPAm-co-Am-SH-modified AuNP.
PNIPAm-co-Am-SH (0.31 mg, 51.6 nmol) was added to 5 ml of water containing 1.90 nM suspended AuNP to yield a PNIPAm-co-Am-SH/particle molar ratio of 5000 : 1 (figure ). After overnight incubation at room temperature, excess polymer was removed by gel filtration chromatography using a Sephadex G75 column (50 cm length) prepacked with fine resin and eluted with distilled water. Fractions containing the polymer-coated AuNP were pooled and the volume was reduced under vacuum.
Figure 2 Schematic showing the preparation of PNIPAm-co-Am-SH modified AuNP (reproduced with permission from [Citation31], ©2009, The Royal Society of Chemistry).

Immobilization of lactobionic acid on magnetite nanoparticles
The modification of magnetite nanoparticles (MNPs) with lactobionic acid (LA) involves several steps. First, MNPs of approximately 10 nm diameter were precipitated in alkali solution from a solution of Fe (II) and Fe (III) chloride by the standard co-precipitation technique. Then, MNPs were silane-coated with (3-aminopropyl) triethoxysilane (APTES) by a silanization reaction. LA, which had been prepared by ring opening reaction of lactose l-hydrate, was then attached to the aminosilane-coated MNPs (figure ) as follows: LA (0.032 g, 9.2×10−4 m) was dissolved in 100 ml of phosphate buffer solution (pH 7) containing water-soluble carbodiimide (WSC) and incubated for 2 h. This solution was then mixed with 1 g of aminosilane-coated magnetite and stirred for 48 h at room temperature. The reaction solution was dialyzed for 2 days using sodium bicarbonate buffer [Citation20].
Figure 3 Schematic showing the synthesis of lactobionic acid (reproduced with permission from [Citation20], ©2009, Elsevier, Ltd).
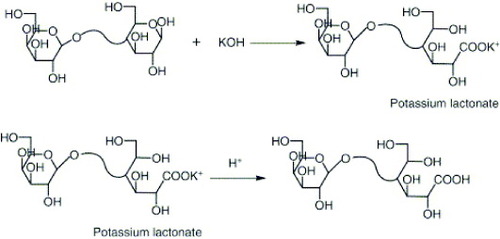
Figure 4 Schematic showing the immobilization of lactobionic acid on magnetite nanoparticles (reproduced with permission from [Citation20], ©2009, Elsevier Ltd).
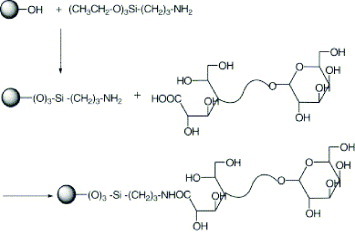
Immobilization of oligonucleotides on silica nanoparticles [Citation66]
The silica nanoparticles used in the study were synthesized by the optimized procedure [Citation81]. The surface modification was carried out in two stages (figure ). In the first step, the silica nanoparticles were immersed in a solution consisting of 1 g of pure ZrCl4 in 50 ml of dry ethanol. The mixture was refluxed for 8 h at 80 °C and the resulting material was centrifuged and washed by decantation. The modified nanoparticles were thoroughly washed with water on an ultrasonicator to remove all chloride ions and then dried. In the second step, 10 ml of 50 mM phosphoric acid was added to the Zr4+-modified silica nanoparticles and the mixture was kept in an ultrasonicator overnight. Later, the phosphate-modified silica nanoparticles were purified by washing thoroughly with double-distilled water in an ultrasonicator.
Figure 5 Schematic showing the immobilization of 5′-amine modified oligonucleotides onto Zr-phosphate-modified silica nanoparticles and hybridization with target oligonucleotides (reproduced with permission from [Citation66], ©2009, Elsevier Ltd).
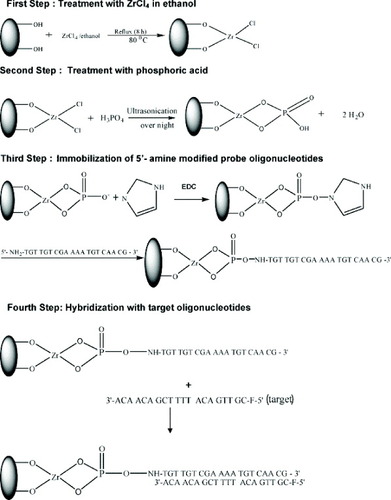
The immobilization of 5′-amine-modified oligonucleotide probes on phosphate-modified silica nanoparticles was carried out by modified methods [Citation82]. A reaction mixture was prepared by mixing 100 μl of probe oligonucleotide solution with 20 μl of 0.1 M N-ethyl-N′-(3-dimethylaminopropyl) carbodiimide (EDC) and 50 μl of 0.1 M imidazole solution and followed by dilution to 2 ml with 10 mM Tris–HCl buffer. The 0.1 M imidazole solution was prepared by dissolving imidazole in deionized water and the pH was adjusted to 10. This reaction mixture was mixed with 0.1 g of particles for DNA immobilization at 38 °C for 5 h. In the presence of EDC, phosphoriimidazolide derivatives of silica nanoparticles were generated by reacting the Zr(PO4)-modified silica nanoparticles with imidazole. In the next process, the phosphoriimidazolide derivatives of silica nanoparticles strongly bind with 5′-amine-modified probe oligonucleotides (figure ).
Immobilization of DNA on calcium phosphate nanoparticles
DNA-coated calcium phosphate nanoparticles were prepared as follows (figure ): an aqueous solution of calcium nitrate (18 mM) was mixed with an aqueous solution of diammonium hydrogen phosphate (10.8 mM). The pH of both solutions was adjusted beforehand to 9 with NaOH (0.1 M). Mixing was accomplished by rapidly pumping both solutions into a glass vessel. After about 30 s, 1 ml of the dispersion was taken with a syringe and rapidly mixed with 0.2 ml of an aqueous solution of DNA (1 mg ml−1) in a sterile Eppendorf tube. This dispersion had a concentration of 167 μg DNA ml−1.
Figure 6 Schematic showing the preparation of DNA-functionalized calcium phosphate nanoparticles (reproduced with permission from [Citation72], ©2009, Elsevier Ltd).
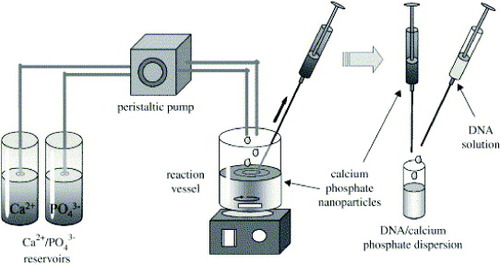
Multishell nanoparticles were prepared as follows: first, DNA-coated calcium phosphate nanoparticles were prepared as described above. To this dispersion, 0.5 ml of calcium nitrate (18 mM) and then 0.5 ml of diammonium hydrogen phosphate (10.8 mM) were added. This resulted in the crystallization of calcium phosphate on the surface of the particles. A colloidal stabilization was then accomplished by adding 0.2 ml of DNA solution (1 mg ml−1). In principle, this procedure can be repeated to add more layers of DNA and calcium phosphate. These dispersions had concentrations of 91 μg DNA ml−1 (double-shell) and 167 μg DNA ml−1 [Citation72].
Immobilization of peptides on quantum dots
Commercial core/shell CdSe/ZnS QDs were precipitated from an initial toluene solution with methanol and dried under vacuum and then redispersed in a pyridine solution (figure ). Peptides were dissolved in anhydrous 99.8% dimethyl sulfoxide (DMSO) at a concentration between 10 and 30 mM. The QDs in pyridine and the peptide solution in DMSO were mixed together. After the addition of tetraethylammonium hydroxide, aggregates of fluorescent QD-peptide were isolated by centrifugation for 30 min at 15 000 g (figure ). After removing the supernatant, the QDs were dried for 2 h and then redispersed in water. To remove the aggregated nanocrystals, this solution was ultracentrifuged at 100 000 g for 30 min on a 50% sucrose cushion. The resulting sample was passed through a G25 spin column and dialyzed against pure water to remove sucrose and the excess free peptide. Before use, the QD-peptide solution was filtered through a 0.22 μm millipore filter (Millex HV, Sigma-Aldrich) [Citation83].
Figure 7 Schematic showing the synthetic route for the PEGylated peptide ND (reproduced with permission from [Citation83], ©2009, American Chemical Society).
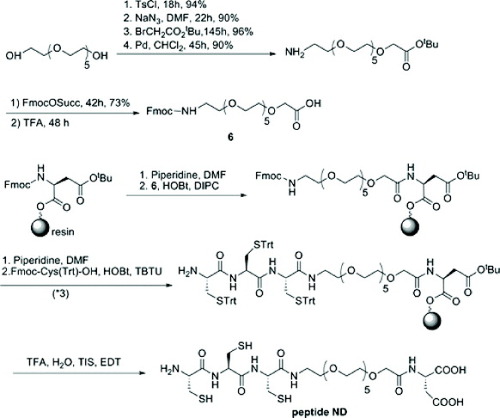
Inorganic nanoparticles for MR imaging, drug delivery and targeting of cancer cells
Inorganic nanoparticles for MR imaging
Selim et al [Citation20] studied MR images of the front and middle parts of rabbit liver before and after injection of LA-modified MNPs through the ear vein (figure ). From figure , it is clearly seen that the color of the liver cells (hepatocytes) became dark after the injection of the modified MNPs (figure (c)). This phenomenon provides strong evidence of the accumulation of LA-modified nanoparticles into the liver cells (hepatocytes). Furthermore, figure (d) reveals that bile canaliculi were not darkened after the injection of the modified MNPs, which indicates that hepatocytes are absent in this area of the biliary tree. This result indicates that the LA-modified magnetites have a considerable potential to be used as a specific recognition marker for hepatocytes.
Figure 8 MR images of rabbit liver: (a) front part, (b) middle part of liver before injection and (c) front part, (d) middle part of liver after injection of lactobionic acid-coated magnetite nanoparticle solution (reproduced with permission from [Citation20], ©2009, Elsevier Ltd).
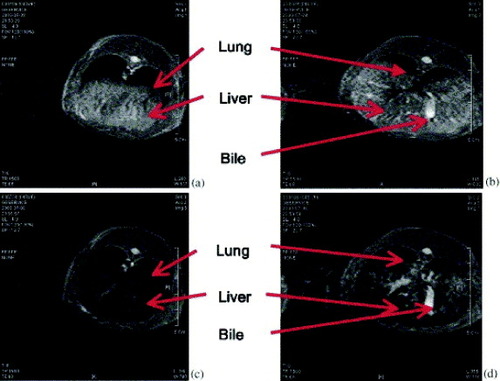
Gilad et al reported the labeling and tracking of rat glioma cells with either MnO nanoparticles or SPIO (figure ) [Citation84]. Cells labeled with MnO nanoparticles and SPIO were transplanted in contralateral brain hemispheres of a rat and tracked by in vivo MRI. SPIO-labeled cells showed a strong negative contrast in T2/T2∗-weighted MRI. However, it is difficult to distinguish them from blood/hemosiderin-associated hypointense regions. MnO-labeled transplanted cells had a higher R1 than the brain tissue surrounding the transplanted cells. Therefore, MnO-labeled cells could be successfully detected and distinguished with positive contrast for in vivo T1-weighted MRI. The authors showed an MR ‘double labeling’ technique using opposite contrasts simultaneously on two sites, where one is labeled with MnO and the other with SPIO nanoparticles. This labeling method can be applied to the tracking of cell populations that are injected of different locations or at different time points, by complementing and amplifying two types of contrasting effect.
Figure 9 In vivo MRI of labeled 9L cells 24 h after transplantation in the striata of rat brain. (a–c): Spin echo image (TR=1000 ms, TE=14.1 ms). (d–f): R1 maps. (g–i): R2 maps. (j–l): R1/R2 merged maps. Shown are representative images of three out of seven rats, two injected with MnO- and FeO-labeled cells (a,b), and one with FeO-labeled cells and unlabeled cells (c; control). Note the simultaneous double contrast in (j–l) (reproduced with permission from [Citation84], ©2009, John Wiley and Sons).
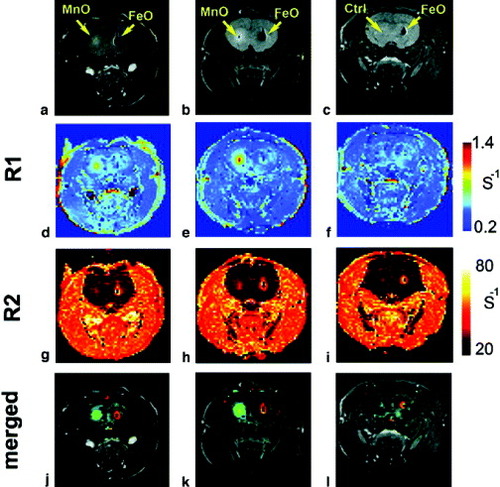
Oostendorp et al [Citation85] evaluated the ability of cyclic Asn-Gly-Arg (cNGR) to target angiogenic tumor ECs in tumor-bearing nude mice by injecting them with cNGR-labeled or unlabeled paramagnetic quantum dots (pQDs). Tumor volumes of cNGR and control groups did not differ in MR images (mean±SD, 1.0±0.7 cm3 and 1.0±0.6 cm3, respectively). For both cNGR-labeled and unlabeled pQDs, changes in R1 (ΔR1) were spatially heterogeneous throughout the tumor and were most pronounced at the tumor rim (figure (A)). Averaged over all mice, the ΔR1 induced by cNGR-pQDs ranged up to ∼0.3 s−1, which was considerably larger than the intrinsic variation in precontrast tumor R1 of 0.1 s−1. Furthermore, the range in ΔR1 was relatively large compared with the precontrast tumor R1 of 0.8 s−1. Administration of unlabeled pQDs resulted in threefold lower response range (ΔR1<0.1 s−1) than in the case of cNGR-pQDs administration. Taken together, the presented results show that cNGR-labeled paramagnetic quantum dots are suitable for the noninvasive visualization and quantification of tumor angiogenic activity by in vivo molecular MRI. Their results provide a promising basis for further developments in contrast agent design and synthesis, data acquisition, and post-processing techniques, which may be valuable for future clinical applications to pathologies in which abnormal vessel growth plays a pivotal role.
Figure 10 T2-weighted anatomic images with color overlay of ΔR1 (A) and ΔS0 (B) for tumor (T) and muscle (M) tissue of mice injected with cNGR-labeled or unlabeled pQDs (n=7 for both groups). Changes in R1 were most pronounced at the tumor rim for cNGR-pQDs. Although an R1 increase in the tumor rim was also observed for unlabeled pQDs, the average response was threefold lower than in the case of cNGR-pQDs, indicating a high specificity of cNGR for angiogenic tumor endothelium. This is further supported by the minimal changes in R1 found in muscle tissue. Changes in S0 (B) colocalized almost completely with changes in R1 (A) (reproduced with permission from [Citation85], ©2009, Waverly Press).
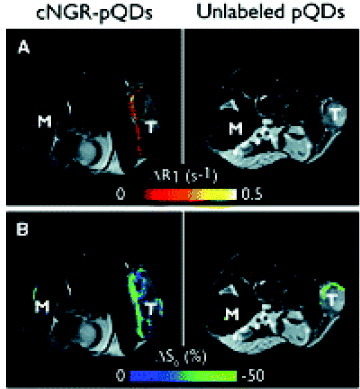
Inorganic nanoparticles for drug delivery
Cengelli et al [Citation40] demonstrated that a hierarchical building of a covalent drug–USPIO assembly is possible, even for highly hydrophobic drugs like camptothecin (CPT). The amount of CPT-USPIO taken up by human Me300 melanoma cells after 16 and 36 h as a function of the number of added nanoparticles was determined from the amount of cell-associated iron and cell-associated CPT fluorescence (figures (a) and (b)) as compared with that of the previously described aminoPVA-USPIOs [Citation86]. After 16 h, the uptakes of aminoPVA-USPIOs and CPT-USPIO were comparable (figure (a)). However, after 36 h, the uptake of CPT-USPIO continued to increase in the still-viable cells, whereas the uptake of non-cytotoxic aminoPVA-USPIOs had saturated (figure (b)). The histological determination of cell-associated iron (figure (c)) and CPT fluorescence (figure (d)) showed the incorporation of CPT-USPIO in the melanoma cells. The intracellular localization of the iron oxide in cell organelles was further demonstrated by transmission electron microscopy (TEM, figure (e)). The appearance of the vesicles was suggestive of lipid vesicles. To confirm the localization of CPT-USPIO in melanoma cells, histological staining of lipids (figures (a) and (b)), TEM imaging (figure (c)) and elemental analysis of USPIO-containing granules (figure (d)) were performed, the results of which strongly suggesting the localization of CPT-USPIO in lipid vesicles. Therefore, the cell uptake mechanisms, accessibility of the conjugate to esterolytic enzymes for drug release, and intracellular routing of these CPT-USPIO assemblies are of utmost importance and must be collectively considered for efficient drug delivery. Further studies in this fascinating area of magnetically directed drug delivery are being actively carried out and the results will be reported in the future.
Figure 11 Human Me300 melanoma cells were exposed for (a) 16 or (b) 36 h to increasing concentrations of CPT-USPIOs 4 or aminoPVA-USPIOs and the cell iron content was determined. CPT-USPIOs 4 (▪); aminoPVA-USPIOs (×). The uptake by cells of CPT associated with the USPIOs was determined on the basis of its specific cell-associated fluorescence in the intact cell layer (▪, inserts). The uptake of CPT-USPIOs (0.7 μM CPT in CPT-USPIOs, 8 μg Fe ml−1) after 36 h was also examined in parallel cultures of Me300 cells grown on histological slides: (c) histological Prussian blue reaction; (d) CPT cell-associated characteristic fluorescence; and (e) transmission electron microscopy (TEM) image demonstrating the iron oxide core of the CPT-USPIOs in intracellular organelles (↗) (reproduced with permission from [Citation40], ©2009, Wiley Inter Science).
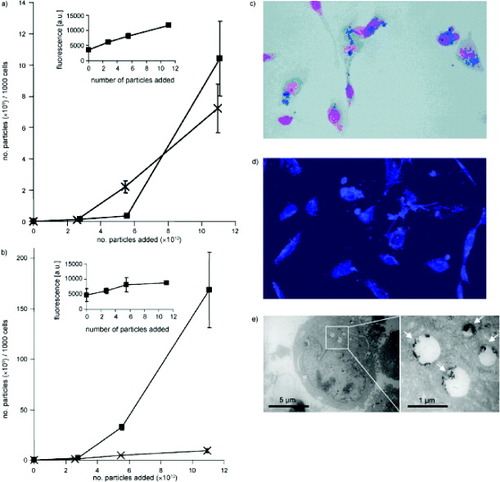
Figure 12 Human Me300 melanoma cells were exposed to CPT-USPIOs (8 μg Fe ml−1, 0.7 μM CPT) for 36 h, and (a) stained with Sudan for lipid visualization (brownish spots, see (b) for magnification) or (c) examined by TEM. (d) Elemental analysis of iron was also performed (reproduced with permission from [Citation40], ©2009, Wiley Inter Science).
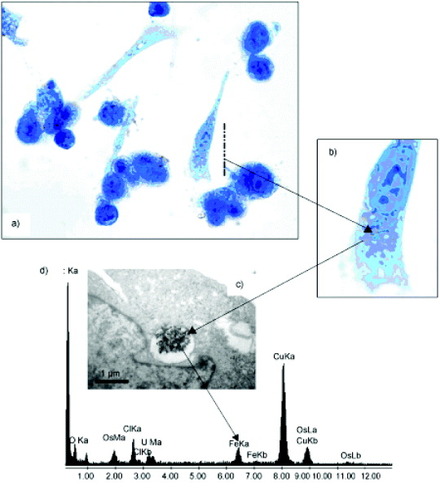
Cheng et al [Citation87] identified the mechanism of how a hydrophobic photodynamic therapy (PDT) drug such as silicon phthalocyanine 4 (Pc 4) is delivered to HeLa cells by PEGylated Au nanoparticle (NP) vectors. Inductively coupled plasma−mass spectroscopy (ICP–MS, figure (a)), TEM (figures (b) and (c)), and confocal fluorescence images (figure ) indicated that there are only very few AuNPs taken up into the cells. After quantifying the AuNPs on the basis of ICP analysis and the amount of Pc 4 in the HeLa cells, the final ratio of Pc 4 to AuNPs in the cells, incubated with the Au NP−Pc 4 conjugates for 24 h, was ∼2.4×103:1. For the as-synthesized conjugates, the initial Pc 4/AuNP ratio is ∼30:1. This implies that most (∼99%) Pc 4 molecules were released from the NP before cellular uptake and were delivered to the cells without the NPs. They concluded that the drug delivery mechanism of Pc 4 to HeLa cells is therefore a combination of the release of Pc 4 from NPs outside the cell (∼99%, path A) and the release from NPs inside the cells (1%, path B), as illustrated in scheme . This research work showed that varying the bond strength between the drug and AuNP surface could aid in timing and targeting the drug delivery as well as in optimizing the therapeutic outcome.
Figure 13 (A) ICP analysis of the uptake of AuNPs by HeLa cells after incubation with PEGylated AuNP−Pc 4 conjugates ([AuNP]=33 nM) for 24 h (∼6×106 cells per dish). (B, C) TEM images of 70 nm-thick slices of HeLa cells after incubation with PEGylated AuNP−Pc 4 conjugates ([AuNP]=330 nM) for 24 h. The arrows indicate the positions of AuNPs. The scale bar is 200 nm (reproduced with permission from [Citation87], ©2009, American Chemical Society).
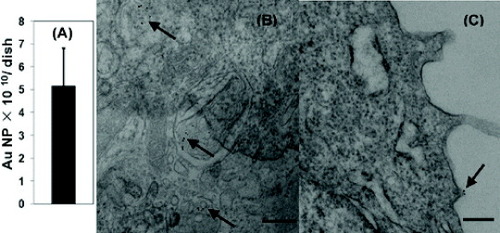
Figure 14 (A) Confocal images of live HeLa cells after incubation with Pc 4, AuNP−Pc 4, and AuNP−Pc 219 conjugates at 37 °C for 24 h and colocalization with NAO in HeLa cells. The scale bar in the images is 10 μm. (B) The fluorescence colocalization of Pc and NAO was analyzed using ImageJ and by calculating the correlation coefficient (Pearson's r) of the red and green pixels in the overlay images. Six independent images were analyzed for each condition (reproduced with permission from [Citation87], ©2009, American Chemical Society).
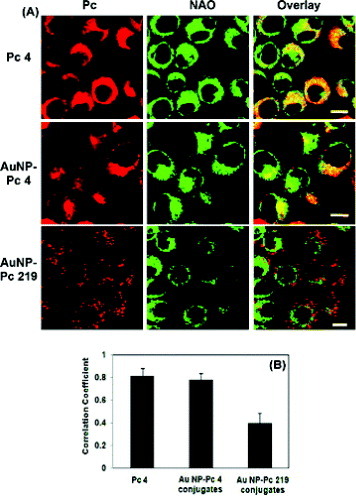
Scheme 1 Observed cellular uptake of phthalocyanines delivered as AuNP conjugates. (A) For the amino-bound Pc 4, a release of the PDT drug into the cell by diffusion leads to localization in mitochondria. (B) Incubation of covalent thiol-bound Pc 219-NP conjugates leads to endocytosis and drug release within the vesicles (reproduced with permission from [Citation87], ©2009, American Chemical Society).
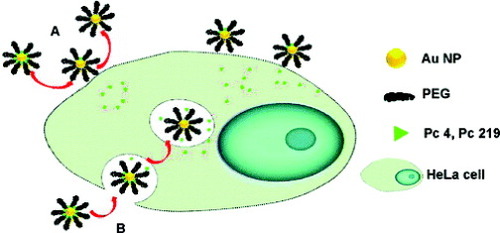
Inorganic nanoparticles for targeting cancer cells
Huang et al [Citation30] demonstrated the potential use of nucleic acid ligand (Aptamer)-conjugated gold nanoparticles (AuNPs) for cancer cell detection. After incubation with the Apt-AuNPs, the incorporated NPs led to the appearance of spotted patterns within the cytoplasms of the MDA-MB-231 and Hs578 T cancer cells. Aggregation of the Apt-AuNPs resulted in bright yellowish scattered spots that allowed easy identification of individual cells (figure (b)). In contrast, only small and faintly reddish dots were observed within the MCF-7 (cancer) and H184B5F5/M10 cells, indicating less aggregation in those cells. Aggregation of the Apt-AuNPs occurred mainly as a result of specific binding through cross-linking with the platelet-derived growth factor (PDGF) [Citation88] present in the cytoplasm of the cells; this result is supported by the fact that only a slight degree of aggregation occurred in the MCF-7 (cancer) and H184B5F5/M10 cells, which have lower amounts of PDGF. In contrast, for the control experiment, they observed no significant differences between the scattering images of the four types of cells in the absence and presence of MH-AuNPs (figures (a) and (c)). This finding suggests that specific interactions occurred between the Apt-AuNPs and PDGF.
Figure 15 Light scattering images of three sets of cancer cells (MDA-MB-231, Hs578 T and MCF-7) and one set of normal cells (H184B5F5/M10) cultured (a) in the absence of Apt-AuNPs, and in the presence of (b) Apt-AuNPs and (c) MH-AuNPs (control) for 3 h at 37 °C in a humidified atmosphere with 5% CO2 (in air) (reproduced with permission from [Citation30], ©2009, Springer).
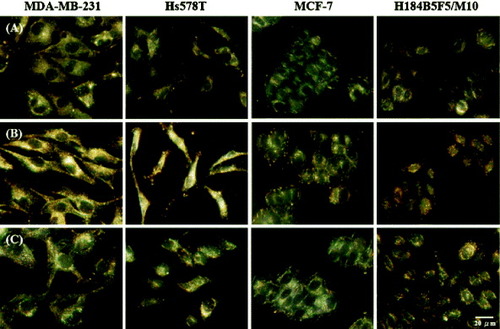
The TEM images further showed evidence of the PDGF-induced aggregation of Apt-AuNPs within the MDA-MB-231 (cancer) and H184B5F5/M10 cells. The TEM images (figure ) reveal that Apt-AuNPs accumulated predominantly within the random area of the cytoplasm in these two cells. Because the NPs used in this study were too large to cross the nuclear membrane through nuclear pores (<30 nm), they observed no light scattering from the nucleus [Citation89]. The TEM images also suggested that the aggregation of the Apt-AuNPs inside the MDA-MB-231 cancer cells (figure (a)) occurred to a greater extent than inside the H184B5F5/M10 cells (figure (b)). This finding is consistent with the results of the light scattering measurements shown in figure (b); larger aggregates scattered light more strongly.
Figure 16 TEM images of Apt-AuNPs in (a) MDA-MB-231 cancer cells and (b) H184B5F5/M10 cells. The Apt-AuNP aggregates are marked with black arrows. The cells were exposed to the Apt-AuNPs for 3 h, fixed with osmium tetroxide, sectioned, and then visualized using a Hitachi H-7100 electron microscope (reproduced with permission from [Citation30], ©2009, Springer).
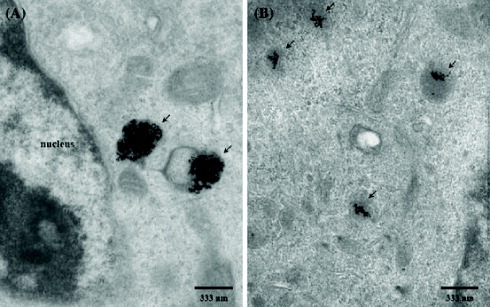
To test the ability of the Apt-AuNPs to differentiate normal cells from some types of cancer cells, Huang et al cultured MDA-MB-231 cancer cells in the presence of H184B5F5/M10 cells and then analyzed these mixtures by dark field microscopy (DFM). One representative image (figure (a)) shows that the combination of DFM and Apt-AuNPs is practical for the identification of cancer cells. The bright cells that are highlighted by red arrows are MDA-MB-231 cancer cells; the others are H184B5F5/M10 cells. They were able to differentiate cancer cells from normal ones even at a cell number ratio of 1 : 100 (figure (b)). This phenomenon allowed the differentiation of cancer cells from normal cells using a dark field optical microscope.
Figure 17 Dark field microscopy images of mixtures of cancer MDA-MB-231 and normal H184B5F5/M10 cells after incubation for 3 h in a medium containing Apt-AuNPs. The original ratios of the cell numbers of MDA-MB-231 and H184B5F5/M10 were (a) 1 : 1 and (b) 1 : 100. The MDA-MB-231 cancer cells are highlighted with red arrows; the other cells are H184B5F5/M10 cells (reproduced with permission from [Citation30], ©2009, Springer).
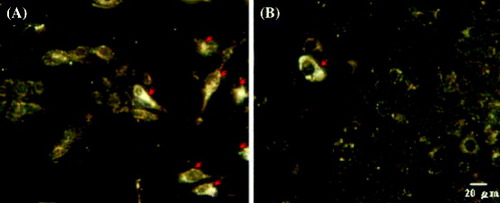
Yong et al [Citation79] reported the successful use of non-cadmium-based quantum dots (QDs) as highly efficient and non-toxic optical probes for imaging live pancreatic cancer cells. The QDs were conjugated with anticlaudin 4, whose corresponding antigen receptors are known to be overexpressed in both primary and metastatic pancreatic cancer cells [Citation90]. Figure (a) shows the labeling of pancreatic cancer cells (MiaPaCa) with the bioconjugates, anticlaudin 4-InP/ZnS QDs. Cellular uptake of the QD bioconjugates can be clearly observed from the robust optical signal of the MiaPaCa cells, whereas no or little signal is observed in the case of cells treated with non-bioconjugated QDs (figure (b)), which demonstrates the specific nature of the antibody-mediated targeting of the pancreatic cancer cells. Local spectral analysis of the overall cell staining by the bioconjugated QDs confirms the origin of the cellular luminescence signal from the InP/ZnS QDs. More importantly, no signs of morphological damage to the cells were observed upon treatment with various QDs, thereby illustrating their low cytotoxicity. The observed staining pattern is indeed due to the functionalized QDs, which have been demonstrated in several reports [Citation91–95]. In addition to monoclonal antibody conjugation, QDs were also conjugated with a polyclonal antibody, anti-PSCA, for the specific targeting of human pancreatic cancer cells. Figure (c) shows confocal images of MiaPaCa cells labeled with anti-PSCA-QD bioconjugates. A strong uptake of the anti-PSCA-QD bioconjugates was also observed. These prepared QD bioconjugates (both monoclonal and polyclonal antibodies) were also further tested in a low-passage pancreatic cancer cell line XPA3, and a similar labeling trend was observed (figure (d)). The results clearly show that the engineered InP/ZnS bioconjugates can serve as potential biocompatible-targeted nanoprobes to specifically diagnose human pancreatic cancer cells.
Figure 18 Confocal microscopy images of (a) MiaPaCa cells treated with anticlaudin 4-conjugated InP/ZnS QDs; (b) MiaPaCa cells treated with unconjugated InP/ZnS QDs; (c) MiaPaCa cells treated with anti-PSCA-conjugated InP/ZnS QDs; (d) XPA3 cells treated with anticlaudin 4-conjugated InP/ZnS QDs; and (e) KB (human nasopharyngeal epidermal carcinoma cell line) cells treated with anticlaudin 4-conjugated InP/ZnS QDs. In all cases, blue represents emission from Hoechst 33342 and red represents emission from InP/ZnS QDs (reproduced with permission from [Citation79], ©2009, American Chemical Society).
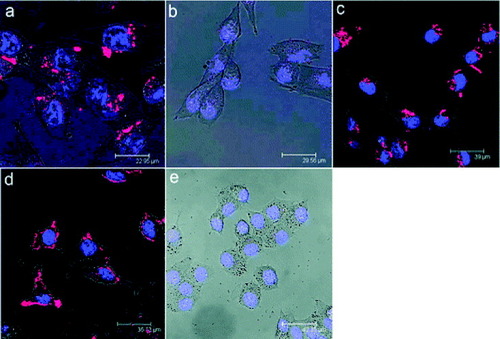
Rosenholm et al [Citation96] developed a selective nanoparticulate system for cancer cell targeting based on fluorescently labeled, poly (ethylene imine) (PEI)-functionalized and folic acid (FA)-conjugated mesoporous silica nanoparticles. To identify the ability to target the FITC/PEI/FA-functionalized silica particles to HeLa cancer cells, they compared the nanoparticle uptake by HeLa cervical carcinoma cells (HeLa) and human embryonic kidney cells (293) under identical conditions. A clearly higher number of the green fluorescent FITC/PEI/FA-functionalized silica particles were detected in the HeLa cells (figure (b)) than in the 293 cells (figure (a)). Even more importantly, the mean fluorescence (MFI) values measured for the two cell types indicated that the FITC-positive HeLa cells had internalized 5–6 times more of the FITC/PEI/FA-functionalized silica nanoparticles than had the 293 cells, as shown in figure (c). The total number of particles internalized by the HeLa cancer cells was therefore about an order of magnitude higher than that internalized by 293 cells, a difference high enough to be of significant therapeutic importance. The selective uptake of FITC/PEI/FA particles by HeLa cells was additionally verified in co-culture experiments, that is, where both HeLa cells and 293 cells were incubated together, as shown in figure . The fact that green emission due to FITC was observed almost exclusively within HeLa cells confirms the preferential uptake of the silica particles by the HeLa cancer cells with elevated folate receptor expression as compared with the non-cancerous 293 cells, positively proving successful targeting of hybrid silica particles also under co-culture conditions.
Figure 19 Specific particle endocytosis of FITC/PEI/FA-functionalized silica nanoparticles. 293 cells (A) or HeLa cells (B) were left untreated (control) or incubated with nanoparticles (10 μg ml−1) for 4 h. Then, the extracellular fluorescence was quenched with trypan blue stain. The endocytosed particles with FITC label (green) inside CMAC-labeled (blue) cells were detected by confocal microscopy (A and B) or flow cytometry (C). The mean fluorescence intensity (MFI) of FITC in the cells was measured and the values were normalized to particle endocytosis in HeLa cells (n=3; mean±SEM) (reproduced with permission from [Citation96], ©2009, American Chemical Society).
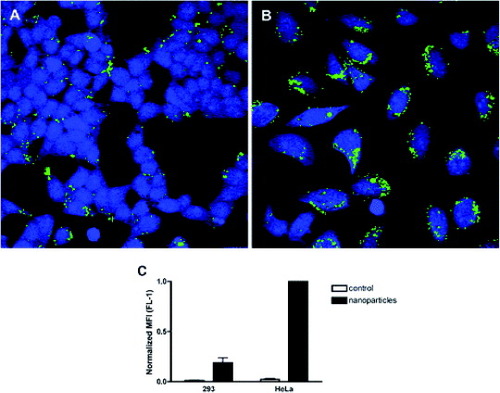
Figure 20 Specific endocytosis of FITC/PEI/FA-functionalized silica nanoparticles in coculture of HeLa and 293 cells. The cells were labeled with blue CMAC (HeLa) or Cell Tracker Red (293) and plated together overnight prior to incubation with the particles for 4 h. After incubation, the extracellular fluorescence was quenched with trypan blue, and the endocytosed particles with FITC label (green) inside blue-labeled or red-labeled cells were detected by confocal microscopy. Scale bar is 10 μm. The results are representative of two independent experiments (reproduced with permission from [Citation96], ©2009, American Chemical Society).
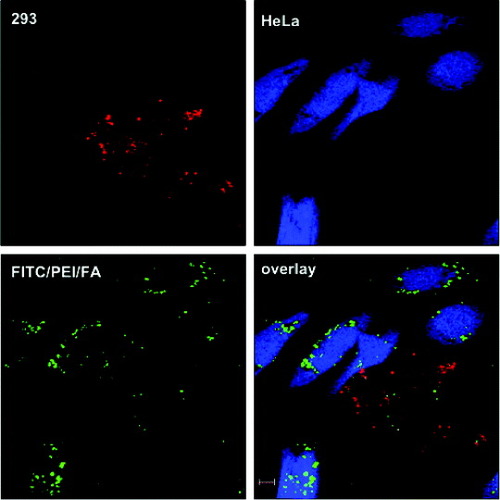
Conclusions
We have provided a brief overview of the immobilization of biomolecules on inorganic nanoparticles. It is clear from the literature that such immobilization is very effective and user-friendly. Moreover, experimental results demonstrate that inorganic nanoparticles can be used for MR imaging, drug delivery and targeting of cancer cells after biomolecules are immobilized on their surfaces. It is expected that the surface modification of inorganic nanoparticles will provide a wide range of applications in the fields of life sciences, environmental science and other areas in the near future.
Acknowledgments
This work was supported by the Korea Ministry of Education, Science and Technology (Contract No. 2009-0073282).
References
- GeorganopoulouD GChangLNamJ MThaxtonC SMufsonE JKleinW LMirkinC A 2005 Proc. Natl Acad. Sci. USA 102 2273 http://dx.doi.org/10.1073/pnas.0409336102
- GaoXCuiYLevensonR MChungL W KNieS 2004 Nat. Biotechnol. 22 969 http://dx.doi.org/10.1038/nbt994
- WuXLiuHLiuJHaleyK NTreadwayJ ALarsonJ PGeNPealeFBruchezM P 2002 Nat. Biotechnol. 21 41 http://dx.doi.org/10.1038/nbt764
- LeeJ H et al 2007 Nat. Med. 13 95 http://dx.doi.org/10.1038/nm1467
- NaH B et al 2007 Angew. Chem., Int. Edn. Engl. 46 5397 http://dx.doi.org/10.1002/anie.200604775
- SlowingI ITrewynB GLinV S Y 2007 J. Am. Chem. Soc. 129 8845 http://dx.doi.org/10.1021/ja0719780
- KohlerNSunCFichtenholtzAGunnJFangCZhangM 2006 Small 2 785 http://dx.doi.org/10.1002/smll.200600009
- MedarovaZPhamWFarrarCPetkovaVMooreA 2007 Nat. Med. 13 372 http://dx.doi.org/10.1038/nm1486
- BulteJ W MModoM M J 2008 Nanoparticles in Biomedical Imaging–Emerging Technologies and Applications New York Springer
- ElabundeK J 2001 Nanoscale Materials in Chemistry New York Wiley-Interscience
- AlivisatosA P 2004 Nat. Biotechnol. 22 47 http://dx.doi.org/10.1038/nbt927
- WangJ 2005 Small 1 1036 http://dx.doi.org/10.1002/smll.200500214
- RosiN LMirkinC A 2005 Chem. Rev. 105 1547 http://dx.doi.org/10.1021/cr030067f
- KumarC S S R 2007 Nanomaterials for Medical Diagnosis and Therapy Weinheim Wiley-VCH
- SchmidG 2004 Nanoparticles: From Theory to Application Weinheim Wiley-VCH
- NiemeyerC MMirkinC A 2004 Nanobiotechnology: Concepts, Applications and Perspectives Weinheim Wiley-VCH
- LuA HSalabasE LSchüthF 2007 Angew. Chem. Int. Edn. Engl. 46 1222 http://dx.doi.org/10.1002/anie.200602866
- KumarS S RHormesJLeuschner 2005 Nanofabrication Towards Biomedical Applications: Techniques, Tools, Applications, and Impact Weinheim Wiley-VCH
- KumarC 2005 Biofunctionalization of Nanomaterials Weinheim Wiley-VCH
- SelimK M KHaY SKimS JChangYKimT JLeeG HKangI K 2007 Biomaterials 28 710 http://dx.doi.org/10.1016/j.biomaterials.2006.09.014
- YiD KSelvanS TLeeS SPapaefthymiouG CKundaliyaDYingJ Y 2005 J. Am. Chem. Soc. 127 4990 http://dx.doi.org/10.1021/ja0428863
- SelvanS TPatraP KAngC YYingJ Y 2007 Angew. Chem. Int. Edn. Engl. 46 2448 http://dx.doi.org/10.1002/anie.200604245
- YoonT JKimJ SKimB GYuK NChoM HLeeJ K 2005 Angew. Chem. Int. Edn. Engl. 44 1068 http://dx.doi.org/10.1002/anie.200461910
- SchmidG 2004 Nanoparticles. From Theory to Application Weinheim Wiley-VCH
- SchmidG 1992 Chem. Rev. 92 1709 http://dx.doi.org/10.1021/cr00016a002
- FynanE FWebsterR GFullerD HSantoroJ CRobinsonH L 1993 Proc. Natl Acad. Sci. USA 90 11478 http://dx.doi.org/10.1073/pnas.90.24.11478
- SandhuK KMcIntoshC MSimardJ MSmithS WRotelloV M 2002 Bioconjugate Chem. 13 3 http://dx.doi.org/10.1021/bc015545c
- ThomasMKlibanovA M 2003 Proc. Natl Acad. Sci. USA 100 9138 http://dx.doi.org/10.1073/pnas.1233634100
- JenC PChenY HFanC SYehC SLinY CShiehD BWuC LChenD HChouC H 2004 Langmuir 20 1369 http://dx.doi.org/10.1021/la036154k
- HuangY FLinY WLinZ HChangH T 2009 J. Nanopart. Res. 11 775 http://dx.doi.org/10.1007/s11051-008-9424-x
- SalmasoSCalicetiPAmendolaVMeneghettiMMagnussonJ PPasparakisGAlexanderC 2009 J. Mater. Chem. 19 1608 http://dx.doi.org/10.1039/b816603j
- SalemA KSearsonP CLeongK W 2003 Nat. Mater. 2 668 http://dx.doi.org/10.1038/nmat974
- TkachenkoA GXieHLiuY LColemanDRyanJGlommW RShiptonM KFranzenSFeldheimD L 2004 Bioconjugate Chem. 15 482 http://dx.doi.org/10.1021/bc034189q
- BerryC CCurtisA S G 2003 J. Phys. D: Appl. Phys. 36 R198 http://dx.doi.org/10.1088/0022-3727/36/13/203
- TartajPMoralesM PVeintemillas-VerdaguerSGonz Oles-CarrePoTSernaC J 2003 J. Phys. D: Appl. Phys. 36 R182 http://dx.doi.org/10.1088/0022-3727/36/13/202
- PankhurstQ AConnollyJJonesS KDobsonJ 2003 J. Phys. D: Appl Phys. 36 R167 http://dx.doi.org/10.1088/0022-3727/36/13/201
- SaiyedZ MTelangS DRamchandC N 2003 Biomagn. Res. Technol. 1 2 http://dx.doi.org/10.1186/1477-044X-1-2
- ChanD C FKirpotinDBunnP A 1993 J. Magn. Magn. Mater. 122 374 http://dx.doi.org/10.1016/0304-8853(93)91113-L
- ZhangYYangMParkJ HSingelynJMaHSailorM JRuoslahtiEOzkanMOzhanC 2009 Small 17 1990 http://dx.doi.org/10.1002/smll.200900520
- CengelliFGrzybJ AMontoroAHofmannHHanessianSJuillerat-JeanneretL 2009 Chem. Med. Chem. 4 988
- IijimaS 1991 Nature 354 56 http://dx.doi.org/10.1038/354056a0
- GrujicicMSunY PKoudelaK L 2007 Appl. Surf. Sci. 253 3009 http://dx.doi.org/10.1016/j.apsusc.2006.06.050
- DicksA L 2006 J. Power Sources 156 128 http://dx.doi.org/10.1016/j.jpowsour.2006.02.054
- BalaniKAndersonRLahaTAndaraMTerceroJCrumplerEAgarwalA 2007 Biomaterials 28 618 http://dx.doi.org/10.1016/j.biomaterials.2006.09.013
- AgoHPetritschKShafferM S PWindleA HFriendR H 1999 Adv. Mater. 11 1281 http://dx.doi.org/10.1002/(SICI)1521-4095(199910)11:15<1281::AID-ADMA1281>3.0.CO;2-6
- JaveyAGuoJWangQLundstromMDaiH J 2003 Nature 424 654 http://dx.doi.org/10.1038/nature01797
- CaoQRogersJ 2008 Nano Res. 1 259 http://dx.doi.org/10.1007/s12274-008-8033-4
- FanS SChaplineM GFranklinN RTomblerT WCassellA MDaiH J 1999 Science 283 512 http://dx.doi.org/10.1126/science.283.5401.512
- DillonA CJonesK MBekkedahlT AKiangC HBethuneD SHebenM J 1997 Nature 386 377 http://dx.doi.org/10.1038/386377a0
- ChenR JBangsaruntipSDrouvalakisK AKamN W SShimMLiY MKimWUtzP JDaiH J 2003 Proc. Natl Acad. Sci. USA 100 4984 http://dx.doi.org/10.1073/pnas.0837064100
- KamN W SJessopT CWenderP ADaiH J 2004 J. Am. Chem. Soc. 126 6850 http://dx.doi.org/10.1021/ja0486059
- BiancoAKostarelosKPartidosC DPratoM 2005 Chem. Commun. 5 571 http://dx.doi.org/10.1039/b410943k
- CherukuriPBachiloS MLitovskyS HWeismanR B 2004 J. Am. Chem. Soc. 126 15638 http://dx.doi.org/10.1021/ja0466311
- TangX WBansaruntipSNakayamaNYenilmezEChangY LWangQ 2006 Nano Lett. 6 1632 http://dx.doi.org/10.1021/nl060613v
- ChenZ et al 2008 Nat. Biotechnol. 26 1285 http://dx.doi.org/10.1038/nbt.1501
- ZerdaA D L et al 2008 Nat. Nanotech. 3 557 http://dx.doi.org/10.1038/nnano.2008.231
- WelsherKLiuZDaranciangDDaiH 2008 Nano Lett. 8 586 http://dx.doi.org/10.1021/nl072949q
- HellerD ABaikSEurellT EStranoM S 2005 Adv. Mater. 17 2793 http://dx.doi.org/10.1002/adma.200500477
- AisawaSHiraharaHIshiyamaKOgasawaraWUmetsuYNaritaE 2003 J. Solid State Chem. 174 342 http://dx.doi.org/10.1016/S0022-4596(03)00234-2
- IglesiasA HFerreiraO PGouveiaD XFilhoA G Sde PaivaJ A CFilhoJ MAlvesO L 2005 J. Solid State Chem. 178 142 http://dx.doi.org/10.1016/j.jssc.2004.10.039
- LiBHeJEvansD GDuanX 2004 Appl. Clay Sci. 27 199 http://dx.doi.org/10.1016/j.clay.2004.07.002
- ChoyJ HKwakS YParkJ SJeongY J 2001 J. Mater. Chem. 11 1671 http://dx.doi.org/10.1039/b008680k
- ChoyJ HJungJ SOhJ MParkMJeongJKangY KHanO J 2004 Biomaterials 25 3059 http://dx.doi.org/10.1016/j.biomaterials.2003.09.083
- NalawadePAwareBKadamV JHirlekarR S 2009 J. Sci. Ind. Res. 68 267
- BrinkerC JSchererG W 1990 Sol–Gel Science: The Physics and Chemistry of Sol–Gel Processing Boston, MA Academic
- RaoK SRaniS UCharyuluD KKumarK NLeeB KLeeH YKawaiT 2006 Anal. Chim. Acta 576 177 http://dx.doi.org/10.1016/j.aca.2006.06.019
- QianJWeiMGaoXXuZHeS 2008 Opt. Express 16 19568 http://dx.doi.org/10.1364/OE.16.019568
- KnoppDTangDNiessnerR 2009 Anal. Chim. Acta 647 14 http://dx.doi.org/10.1016/j.aca.2009.05.037
- WeinerSWagnerH D 1998 Annu. Rev. Mater. Sci. 28 271 http://dx.doi.org/10.1146/annurev.matsci.28.1.271
- DorozhkinS VEppleM 2002 Angew. Chem. Int. Edn. Engl. 114 3260 http://dx.doi.org/10.1002/1521-3757(20020902)114:17<3260::AID-ANGE3260>3.0.CO;2-S
- FratzlPGuptaH SPaschalisE PRoschgerP 2004 J. Mater. Chem. 14 2115 http://dx.doi.org/10.1039/b402005g
- SokolovaV VRadtkeIHeumannREppleM 2006 Biomaterials 27 3147 http://dx.doi.org/10.1016/j.biomaterials.2005.12.030
- SokolovaVKovtunAHeumannREppleM 2007 J. Biol. Inorg. Chem. 12 174 http://dx.doi.org/10.1007/s00775-006-0177-7
- NeumannSKovtunADietzelI DEppleMHeumannR 2009 Biomaterials 30 6794 http://dx.doi.org/10.1016/j.biomaterials.2009.08.043
- YinYAlivisatosA P 2005 Nature 437 664 http://dx.doi.org/10.1038/nature04165
- PoznyakS KTalapinD VShevchenkoE VWellerH 2004 Nano Lett. 4 693 http://dx.doi.org/10.1021/nl049713w
- AlivisatosA PGuW WLarabellC 2005 Annu. Rev. Biomed. Eng. 7 55 http://dx.doi.org/10.1146/annurev.bioeng.7.060804.100432
- ZhouMGhoshI 2007 Biopolymers (Pept. Sci.) 88 325 http://dx.doi.org/10.1002/bip.20655
- YongK TDingHRoyILawW CBergeyE JMaitraAPrasadP N 2009 ACS Nano 3 502 http://dx.doi.org/10.1021/nn8008933
- WallingM ANovakJ AShepardJ R E 2009 Int. J. Mol. Sci. 10 441 http://dx.doi.org/10.3390/ijms10020441
- RaoK SEl-HamiKKodakiTMatsushigeKMakinoK 2005 J. Colloid Interface Sci. 289 125 http://dx.doi.org/10.1016/j.jcis.2005.02.019
- LeeG YChenC HWangT HLeeW C 2003 Anal. Biochem. 312 235 http://dx.doi.org/10.1016/S0003-2697(02)00507-9
- DifA et al 2009 J. Am. Chem. Soc. 131 14738 http://dx.doi.org/10.1021/ja902743u
- GiladA AWalczakPMcMahonM TNaH BLeeJ HAnKHyeonTvan ZijlP C MBulteJ W M 2008 Magn. Reson. Med. 60 1 http://dx.doi.org/10.1002/mrm.21622
- OostendorpMKoumaKHackengT MDirksenAPostM Jvan ZandvoortM A M JBackesW H 2008 Cancer Res. 68 7676 http://dx.doi.org/10.1158/0008-5472.CAN-08-0689
- Petri-FinkAChastellainMJuillerat-JeanneretLFerrariAHofmannH 2005 Biomaterials 26 2685 http://dx.doi.org/10.1016/j.biomaterials.2004.07.023
- ChengYSamiaA CLiJKenneyM EResnickABrudaC 2009 Langmuir doi: 10.1021/la902390
- HuangC CHuangY FCaoZTanWChangH T 2005 Anal. Chem. 77 5735 http://dx.doi.org/10.1021/ac050957q
- TkachenkoA GXieHColemanDGlommWRyanJAndersonM FFranzenSFeldheimD L 2003 J. Am. Chem. Soc. 125 4700 http://dx.doi.org/10.1021/ja0296935
- NicholsL SAshfaqRIacobuzio-DonahueC A 2004 Am. J. Clin. Pathol. 121 226 http://dx.doi.org/10.1309/K144PHVDDUPDD401
- YongK TRoyIPudavarH EBergeyE JTramposchK MSwihartM TPrasadP N 2008 Adv. Mater. 20 1412 http://dx.doi.org/10.1002/adma.200702462
- BharaliD JLuceyD WJayakumarHPudavarH EPrasadP N 2005 J. Am. Chem. Soc. 127 11364 http://dx.doi.org/10.1021/ja051455x
- JiangWMardyaniSFischerHChanW C W 2006 Chem. Mater. 18 872 http://dx.doi.org/10.1021/cm051393+
- JiangWSinghalAZhengJWangCChanW C W 2006 Chem. Mater. 18 4845 http://dx.doi.org/10.1021/cm061311x
- QianJYongK TRoyIOhulchanskyyT YBergeyE JLeeH HTramposchK MHeSMaitraAPrasadP N 2007 J. Phys. Chem. B 111 6969 http://dx.doi.org/10.1021/jp070620n
- RosenholmJ MMeinanderAPeuhu Em NiemiRErikssonJ ESahlgrenCLindenM 2009 ACS Nano 3 197 http://dx.doi.org/10.1021/nn800781r