Abstract
Nano-sized hydroxyapatite (nHA) and carbonate-substituted hydroxyapatite (nCHA) particles were incorporated into a poly-2-hydroxyethylmethacrylate/polycaprolactone (PHEMA/PCL) hydrogel at a filler content of 10 wt%. Fourier transform infrared absorption, transmission electron microscopy, x-ray diffraction and scanning electron microscopy were used to analyse the physical and chemical characteristics of the calcium phosphate fillers and resultant composites. Nano-sized calcium phosphate particles were produced with a needle-like morphology, average length of 50 nm and an aspect ratio of 3. The nanoparticles were uniformly distributed in the polymer matrix. The addition of both HA and CHA in nano-form enhanced the bioactivity and biocompatibility of the PHEMA/PCL matrix. The carbonate-substitution has allowed for improved bioactivity and biocompatibility of the resultant composite, indicating the potential of this material for use in bone tissue engineering.
Introduction
Hydrogels can be natural (e.g. collagen) or synthetic (e.g. poly-2-hydroxyethylmethacrylate, PHEMA). They are highly hydrophilic 3D cross-linked networks and are able to swell in water without dissolution [Citation1]. In the body environment, the hydrophilic nature of these polymers enables them to interact well with cells and have been used for a variety of applications such as opthalmics and drug delivery [Citation2, Citation3].
One of the most widely studied hydrogels is PHEMA [Citation4] that has been used as a biomaterial since 1960 for many medical applications [Citation5]. It is non-degradable [Citation6] and has excellent biocompatibility, but owing to its highly hydrophilic nature it is very soft in the wet state making it inadequate for medical applications where mechanical strength is of importance [Citation7]. The addition of a second phase can help to improve these mechanical properties, including tear resistance. Polycaprolactone (PCL) is a degradable, hydrophobic polymer that has been used in combination with PHEMA to make composites that retained the hydrophilic nature of PHEMA and incorporated the mechanical properties of PCL [Citation8]. Ambrosio et al [Citation9] found that with increasing amounts of PCL, the composite imbibed less water. They also found that the compressive strength and stiffness of the composite increased with higher additions of PCL [Citation10]. Hence, the incorporation of PCL into the PHEMA matrix led to improved mechanical properties and allowed better cell adhesion in part, because of the voids that were created following the degradation of PCL.
In recent times, attention has been focused on the incorporation of hydroxyapatite (HA) particles into biocompatible PHEMA/PCL hydrogels that could act as bone fillers and temporary scaffolds [Citation11–13]. The inclusion of the hydroxyapatite particles, both micro and nano-sized, helped improvements of both the mechanical stability and bioactivity of the PHEMA/PCL hydrogels. Comparisons of micron- and nano-sized HA in a polymer matrix have indicated better mechanical and biological properties when nano-sized particles were used [Citation14, Citation15]. The nano-hydroxyapatite (nanoHA or nHA) particles were more evenly distributed and provided a greater surface area for polymer anchorage and at the surface of the composite, a larger surface area for cell interaction.
No other form of calcium phosphate has been attempted as a filler for these PHEMA/PCL composite scaffolds. Hence, in this study, nano-sized hydroxyapatite and carbonate-substituted hydroxyapatite have been incorporated. Carbonate-substituted hydroxyapatite has been demonstrated to have better bioactivity in vitro and in vivo than stoichiometric hydroxyapatite [Citation16–21]. Hence, its potential of providing the PHEMA/PCL composite with greater biologically suitable properties. Following composite characterization, simulated body fluid (SBF) and human osteoblast-like (HOB) cells will be used to observe the effect of and any differences due to the addition of nano-sized carbonate-substituted hydroxyapatite versus stoichiometric hydroxyapatite.
Materials and methods
Production and characterization of nHA and nCHA
NanoHA and nanoCHA were produced using the conventional aqueous precipitation techniques stated by Aoki [Citation22] and Gibson and Bonfield [Citation17], respectively.
NanoHA with a Ca/P ratio of 1.67 was produced by adding dropwise a solution of 0.3 M orthophosphoric acid (H3PO4, AnalaR grade, BDH, UK) to 0.5 M calcium hydroxide (Ca(OH)2, AnalaR grade, BDH, UK) whilst continuously stirring the solutions as room temperature. Throughout, the pH was kept above 10.5 by the addition of ammonia solution. After all the H3PO4 solution had been added, the solution was stirred for a further two hours. The precipitant was then left to age overnight.
NanoCHA was prepared using the same chemicals as those used to make nHA. However, the Ca/P ratio was set at 1.76 to ensure an excess of calcium in the system. Owing to this higher Ca/P ratio, there was no need to use ammonia to adjust the pH. CO2 gas was bubbled into the phosphate solution during its addition to the calcium hydroxide solution to deliver the carbonate ions.
A Siemens D5000 diffractometer with CuKα radiation was used to perform x-ray diffractometry (XRD) on the composite coatings; the x-ray generator was operated at 40 kV and 40 mA. Data were collected over 2θ range of 18–42°, with a step of 0.02° and a count time of 2 s.
Transmission electron microscopy (JEOL 200CX) was used to evaluate the morphology of the calcium phosphate nanoparticles. An accelerating voltage of 200 kV was used during the procedure.
Fourier transform infra-red spectroscopy (FTIR: BioRad FTS6000) was used to characterize the composites. Data were collected over a range of 600–4000 cm−1 with a resolution of 4 cm−1, scanning over 36 times to increase the signal-to-noise ratio.
X-ray fluorescence (XRF) analysis was performed to determine the calcium to phosphorus (Ca/P) molar ratio of both nHA and nCHA. Carbon-hydrogen-nitrogen elemental analysis (CHN) was used to detect the carbon content in the nCHA sample. All analyses were performed by the London and Scandinavian Metallurgical Company Ltd.
Preparation and characterization of nano-calcium phosphate – reinforced PHEMA/PCL hydrogel composites
To produce the nano calcium phosphate-PHEMA/PCL composites (PCL, Aldrich) pellets were melted in 2-hydroxyethyl-methacrylate (HEMA, Aldrich) at 60 °C at a weight ratio of 1:4, respectively. Filtered slurries of nHA/nCHA were then added to create a final composite with a 10 wt% filler content. Benzoyl peroxide (0.5 wt%, Fluka) was added as the initiator, and ethylene glycol dimethacrylate (0.5 wt% EGDMA, Aldrich) was used as the cross-linking agent. The mixtures were then stirred for a further 2 h, during which excess water could be evaporated. Hydrogel without a calcium phosphate filler was produced as the control.
It was found that in order to be assured of the final wt% of HA/CHA in the hydrogel composite and to assure only nanoparticles were present in the mixture, the quantities of Ca(OH2) and H3PO4 had to be down-scaled. Using controlled quantities of each chemical allowed reproducible amounts of nHA/nCHA to be precipitated. Upon gentle filtration to remove the majority of water, the quantity of HA/CHA retained and added to the hydrogel would always be the same. Hence, by altering the amount of hydrogel produced, and adding the same wt% of nHA/nCHA, the desired filler content could be achieved.
Scanning electron microscopy (SEM) was conducted on all coating samples to observe particle distribution and size within the hydrogel matrix. Palladium was used as the coating material prior to observation using a working distance of 10 mm and an accelerating voltage of 15 kV. The samples were also characterized by XRD and FTIR spectroscopy.
Simulated body fluid
Simulated body fluid (SBF) testing was performed to observe the level of bioactivity of the composites. SBF testing was first described by Kokubo et al in 1982 [Citation23]. They devised a technique which mimicked the behaviour of a material in vivo by use of a solution with an ionic composition similar to that of human blood plasma (table ). This could be used to observe the bioactivity of a material in vitro. SBF was made following the method described by Kokubo et al [Citation23]. Disc-shaped samples of 10 mm diameter and 2 mm thickness were placed, in triplicate, into 15 ml of SBF and kept at 37 °C and pH 7.40. The SBF was not changed during this study. Once samples were removed they were washed with deionized water to remove excess SBF and allowed to air dry. The SBF from which samples were removed, was kept at 4 °C until ion analysis was performed using inductively coupled plasma-optical emission spectroscopy (ICP-OES; Jobin-Yvon JY2000-2). ICP-OES was used to determine the levels of calcium and phosphorus ions in the SBF after different sample immersion times.
Table 1 Composition of SBF and human blood plasma.
Thin-film x-ray diffraction (TF-XRD) was used to analyse the apatite that had formed on the surface of each sample. Measurements were collected using a glancing angle of 0.5° over a 2θ range of 18–42°, with a step of 0.02° and a count time of 2 s.
Retrieved SBF samples were coated for 2 min with palladium, and SEM observation was performed with energy dispersive spectroscopy (JEOL 5800LV SEM) using accelerating voltage of 10 kV and a working distance of 10 mm.
AlamarBlueTM assay
Disc-shaped samples of 10 mm diameter and 2 mm thickness placed with 10 wt% nHA/nCHA-PHEMA/PCL hydrogels were prepared and sterilized using ultraviolet exposure for 1 h. PHEMA/PCL hydrogels with no calcium phosphate addition were used as the control.
Approximately 20 000 cells suspended in 50 μl of culture medium (McCoy's Medium plus inactivated Fetal Bovine Serum (10%), penicillin (100 units ml−1), streptomycin (100 μg ml−1), L-glutamine (2 mM) and vitamin C (30 mg ml−1) was gently pippetted onto the centre of the coated substrates and incubated for 4 h at 37 °C and 5% pCO2. Samples with cells, six repeats of each type of composite, were then transferred to 24-well culture plates (using only the central eight wells) and 0.85 ml of culture medium was added to each. Samples were further incubated for 18 h under the same conditions. At 18 h, 0.1 ml of AlamarBlue™ solution was added to each well and the cells were incubated for an additional 6 h. Media was removed from each sample (two repeats) and its fluorescence was quantized at an excitation wavelength of 530 nm and an emission wavelength of 590 nm. Following measurement, the coated samples were gently washed twice with 1 ml phosphate buffer solution (PBS), and 0.9 ml of fresh culture medium was added and the incubation continued for 16 h before the AlamarBlue™ assay repeated. The AlamarBlue™ test was performed for 4 consecutive days. Two cell-free coated samples were used as a blank and the average signal was subtracted from the signal of these samples.
Scanning electron microscopy
To observe cell growth on the substrates, the cells had to be fixed. The fixative was a mixture of 4% gluteraldehyde to 0.1 M PIPES and containing 2 mM CaCl2 kept at 4 °C. The PIPES was kept at pH 7 to be soluble, and therefore NaOH was added to increase the pH of the solution.
Following the cell fixation process, the samples were coated for 30–40 s with palladium in preparation for microscopical analysis. An accelerating voltage of 8 kV was used and a working distance of 8 mm.
Results and discussion
Characterization of nHA and nCHA
XRD analysis of both calcium phosphates indicated no secondary phases, such as calcium oxide, being present (figure ). The nHA particles were slightly longer than the nCHA particles and were more needle-like in appearance (figure ). This finding is reinforced by previous work which illustrated that the substitution of carbonate ions into the hydroxyapatite structure causes the crystallite size to be reduced and for there to be a change from a needle-like structure to an equiaxed form [Citation14–19]. The selected area diffraction pattern (SAD) shows the typical pattern of a calcium phosphate polycrystal.
Figure shows the FTIR spectrum of the nanoparticles of HA and CHA to be used in the hydrogel composites. The stretching and bending motion of phosphate in both nHA and nCHA were assigned to the bands at 563, 601, 1054 and 1091 cm−1. Peaks at 1643 and 3360 cm−1 were assigned to H2O in the calcium phosphates. In the nCHA trace, the absorption band at 1468 cm−1 is related to the carbonate group substitution for a phosphate group. No shoulder was observed at 872 cm−1 indicating no contribution of the carbonate group for the hydroxyl group in the apatite structure. Hence, the nCHA formed in this study was a Type B carbonate group substitution [Citation24].
XRF analysis of the nCHA found the carbonate content to be 4.6 wt%. This is within the range for natural bone of 4–8 wt% [Citation25].
Characterization of nHA/nCHA-hydrogel composite coatings
Figure shows the XRD patterns for the nHA/nCHA- PHEMA/PCL composites. The peaks due to the addition of nHA and nCHA to the PHEMA/PCL matrix are apparent and shown on the patterns.
FTIR measurements on these composites (not included here) showed the HEMA and PCL to be well mixed, forming an interpenetrating network (IPN) of PHEMA/PCL. The peaks ascribed to the phosphate group stretching and bending motions were noted at 1091, 1054, 601 and 563 cm−1. The peak at 872 cm−1 due to the carbonate ions was not noted in the nCHA – reinforced hydrogel samples, but a band was seen at 1468 cm−1.
SEM images indicated that there was a nano-dispersion of the nHA/nCHA particles in the polymer matrix. The particles were seen to gather near the ‘grain’ boundaries of the hydrogel. All composites had an even topography (figure ). However, the sample with 10 wt% nCHA had a smaller ‘granular’ appearance when compared with the pure hydrogel and 10 wt% nHA samples.
In vitro bioactivity
Figure shows the decrease in calcium and phosphorus ion concentrations with immersion time for the hydrogel with and without calcium phosphate fillers. There appears to be a slight increase during the initial period of immersion for the samples contained nano-sized CHA. This can be due to the increased solubility and bioactivity of carbonate-substituted hydroxyapatites [Citation14–19]. For all samples containing a bioactive calcium phosphate filler, there was a significant decrease in the amount of calcium and phosphorus ions detected in the SBF. The overall rate of Ca/P ion depletion was seen to be greatest for the hydrogel with 10 wt% CHA nano-inclusions, indicating a faster rate of apatite growth on the surface of this hydrogel composition.
Figure 6 Variation of calcium (Ca2+) and phosphorus (P5+) ion concentrations in SBF with immersion time.
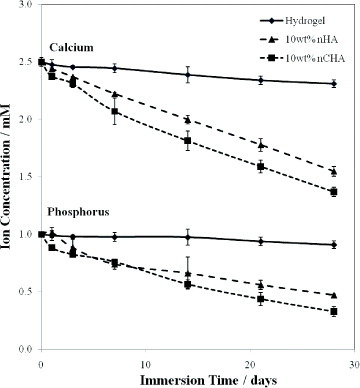
The thin-film XRD and SEM results (figures and ) reinforced the findings of the ICP-OES work. Both techniques indicated calcium phosphate precipitation on the surface of the nHA and nCHA—reinforced PHEMA/PCL composites. The time-point at which an apatite layer formed was reduced when a bioactive filler was added to the hydrogel and when nCHA was used in place of stoichiometric nHA. The 10 wt% nCHA reinforced composite was able to form a continuous apatite layer within a week of immersion in SBF. Performing EDX on the samples demonstrated a good distribution of the nanoparticles. No particle agglomeration was observed. Hence, the new production technique ensured this composite to be truly nano-reinforced.
Figure 8 SEM images of composite surfaces. Only nano-sized crystals of apatite were seen on the hydrogel samples (note the need for higher magnification image), (a) in comparison to the sheet-like coverage of apatite achieved on samples containing nHA (b) and nCHA (c).
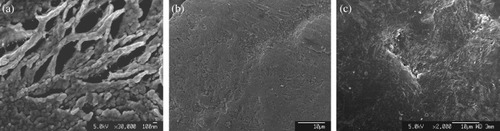
Performing the AlamarBlueTM assay allowed for comparison of the growth of human osteoblast-like cells on the different compositions of hydrogel (figure ). The attachment and growth of these cells occurred on all samples indicating the ability of the hydrogel to support cell development with and without calcium phosphate additions. The samples with nano-sized CHA filler had significantly greater cellular activity than pure hydrogel and nano-sized HA-reinforced hydrogel samples. Hence, these findings indicated that the nano-sized particles (both nHA and nCHA) were sufficiently exposed from within the PHEMA/PCL matrix to be able to present a more favourable surface for cellular attachment and proliferation. The increased ability of CHA to provide a more biocompatible and bioactive surface than HA in vitro and in vivo [Citation14–19] was retained and transferred to this composite scaffold material.
The HOB cells were able to differentiate and proliferate on all materials in this investigation. Figure shows that the HOB cells grew particularly well on the surfaces of the composite containing 10 wt% nCHA. This preliminary cell study shows the biological suitability of the inclusion of nano-sized carbonate-substituted hydroxyapatite in place of stoichiometric hydroxyapatite.
Figure 10 Scanning electron micrographs showing HOB cells growth and attachment to the surface of 10 wt% nCHA-hydrogel composite.
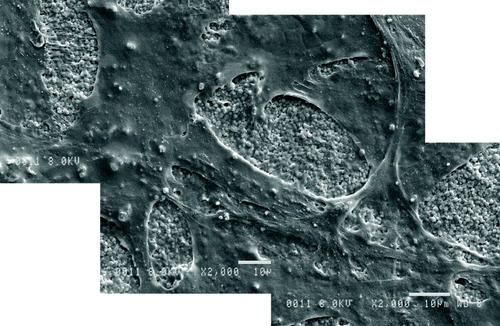
Initial studies of the swelling and mechanical properties of these materials (not shown here) have also illustrated the benefits of including nano-sized particles. These findings are supported by the work of Netti et al [Citation26] and Giordano et al [Citation12] who observed the swelling and mechanical properties of PHEMA and PHEMA/PCL/micro-sized HA, respectively. The present composite of PHEMA/PCL and nano-calcium phosphate particles is able to retain the ability of the hydrogel to swell, allowing the material to be stabilized in vivo and have improved mechanical properties due to the addition of ceramic nanoparticles. The addition of the bioactive nano-filler, especially the carbonate-substituted calcium phosphate particles, allows the PHEMA/PCL to be used in osteo-restorative applications, having suitable resorption, osteo-activity and mechanical strength.
Conclusions
Nano-sized carbonate-substituted hydroxyapatite has been successfully produced and incorporated into a PHEMA/PCL matrix alongside nanoHA—reinforced PHEMA/PCL composites. The nano-structure of the calcium phosphates has been retained without agglomeration of particles in the hydrogel matrix. The carbonate-substitution has allowed for improved bioactivity and biocompatibility of the resultant composites when compared with pure PHEMA/PCL and nHA-reinforced hydrogels, hence broadening the use of hydrogels and creating a novel biomaterial for bone tissue engineering applications.
Acknowledgment
This study was supported by the EU-FP6 Project ‘NEWBONE’ (Contract Number 026279-2).
References
- HoffmanA S 2002 Adv. Drug Deliv. Rev. 54 3 http://dx.doi.org/10.1016/S0169-409X(01)00239-3
- RefojoM F 1975 Medicine and Surgery vol 8 R L Kronenenthal ZOser EMartin New York Plenum p 313
- PeppasA N 1984 Recent Advances in Drug Delivery Systems J MAnderson S WKim New York Plenum p 279
- KostJLangerR 1987 Hydrogels Med. Pharm. 95
- SalernitanoEMigliaresiC 2003 J. Appl. Biomater. Biomech. 1 3
- LeeK YMooneyD J 2001 Chem. Rev. 101 1869 http://dx.doi.org/10.1021/cr000108x
- DavisP AHuangS JNicolaisLAmbrosioL 1991 High Performance Biomaterials: A Comprehensive Guide to Medical/Pharmaceutical Applications MSzycher Lancaster, PA Technomic Publishing p 343
- DavisP ANicolaisLAmbrosioLHuangS J 1988 J. Bioactive Compatible Polym. 3 205 http://dx.doi.org/10.1177/088391158800300302
- AmbrosioLNettiP AIannaceSHuangS JNicolaisL 1996 J. Mater. Sci.: Mater. Med. 7 251 http://dx.doi.org/10.1007/BF00058561
- AmbrosioLSantisR DNicolaisL 1998 Proc. Inst. Mech. Eng. 212 93
- KaufmanJ DSongJKlapperichC M 2007 J. Biomed. Mater. Res. A 81 611
- GiordanoFCausaFDi SilvioLAmbrosioL 2007 J. Mater. Sci.: Mater. Med. 18 653 http://dx.doi.org/10.1007/s10856-007-2316-2
- HuangJLinY WFuX WBestS MBrooksR ARushtonNBonfieldW 2007 J. Mater. Sci.: Mater. Med. 18 2151 http://dx.doi.org/10.1007/s10856-007-3201-8
- WangXLiYWeiJde GrootK 2002 Biomaterials 23 4787 http://dx.doi.org/10.1016/S0142-9612(02)00229-6
- WeiGMaP X 2004 Biomaterials 25 4744
- PorterAPatelNBrooksRBestSRushtonNBonfieldW 2005 J. Mater. Sci. Mater. Med. 16 899 http://dx.doi.org/10.1007/s10856-005-4424-1
- GibsonI RBonfieldW 2002 J. Biomed. Mater. Res. 59 697 http://dx.doi.org/10.1002/jbm.10044
- DoiYShibutaniTMoriwakeYKajimotoTIwayamaY 1998 J. Biomed. Mater. Res. 39 603 http://dx.doi.org/10.1002/(SICI)1097-4636(19980315)39:4<603::AID-JBM15>3.0.CO;2-7
- MerryJ 2000 PhD Thesis Materials Department QMW, College University of London
- HingK AMetterJGibsonI RDi SilvioLBestS MBonfieldW 1999 Bioceramics vol 12 HOhgushi W QHastings TYoshikawa Nara, Japan World Scientific p 195
- HankermeyerC ROhashiK LDelaneyD CRossJConstantzB R 2002 Biomaterials 23 743 http://dx.doi.org/10.1016/S0142-9612(01)00179-X
- AokiH 1991 Science and Medical Applications of Hydroxyapatite Tokyo, Japan Takayama Press p 5
- KokuboTKushitaniHSakkaSKitsugiTYamamuroT 1990 J. Biomed. Mater. Res. 24 721 http://dx.doi.org/10.1002/jbm.820240607
- McConnellD 1973 Apatite: Its Crystal Chemistry, Mineralogy, Utilization, and Geological and Biologic Occurrences 1st edn New York Springer p 39
- DriessensF C M 1980 Bull. Soc. Chim. Belg. 89 663
- NettiP ASheltonJ CRevellP APirieCSmithSAmbrosioLNicolaisLBonfieldW 1993 Biomaterials 14 1098 http://dx.doi.org/10.1016/0142-9612(93)90211-J