Abstract
We propose a new type of ‘smart’ nanofiber (NF) with dynamically and reversibly tunable properties for the ‘on–off’ controlled release of the polysaccharide dextran. The fibers are produced by electrospinning copolymers of N-isopropylacrylamide (NIPAAm) and N-hydroxymethylacrylamide (HMAAm). The OH groups of HMAAm are subsequently crosslinked by thermal curing. The copolymers were successfully fabricated into a well-defined nanofibrous structure with a diameter of about 600–700 nm, and the fibers preserved their morphology even after thermal curing. The resulting crosslinked NFs showed rapid and reversible volume changes in aqueous media in response to cycles of temperature alternation. The fibrous morphology was maintained for the crosslinked NFs even after the cycles of temperature alternation, while non-crosslinked NFs collapsed and dispersed quickly in the aqueous solution. Dextran-containing NFs were prepared by electrospinning the copolymers blended with fluorescein isothiocyanate (FITC)-dextran, and the ‘on–off’ switchable release of FITC-dextran from the crosslinked NFs was observed. Almost all the FITC-dextran was released from the NFs after six heating cycles, whereas only a negligible amount of FITC-dextran was evolved during the cooling process. The reported incorporation of smart properties into NFs takes advantage of their extremely large surface area and porosity and is expected to provide a simple platform for on–off drug delivery.
1. Introduction
Polymeric nanofibers (NFs) fabricated by electrospinning have gained popularity in the last decade because of their versatile and cost-effective fabrication method. Electrospinning is applicable to almost any soluble or fusible polymers and can yield a variety of continuous fibers with uniform diameters ranging from micrometers to nanometers [Citation1]. Potential applications of electrospinning include filtration membranes [Citation2, Citation3], catalytic fibers [Citation4], fiber-based biochips [Citation5], sensors [Citation6], wound healing [Citation7, Citation8], tissue engineering scaffolds [Citation9, Citation10] and drug delivery systems [Citation11, Citation12]. Recently, functionalized NFs have been developed by electrospinning polymers blended with nanoparticles [Citation13, Citation14], carbon nanotubes [Citation15, Citation16], ceramics [Citation17] or biomolecules [Citation18], or by surface modification with peptides [Citation19] or proteins [Citation20]. Furthermore, functional NFs with a core-shell structure have been prepared through coaxial electrospinning with the aim of realizing drug encapsulating fibers [Citation21, Citation22]. In addition to functional NFs fabricated by the above-mentioned methods, the development of NFs that have the ability to trigger or turn material properties ‘on’ or ‘off’ upon the application of external stimuli is critically important for controlling biological responses in biotechnological and biomedical applications [Citation23, Citation24].
Special types of polymers called ‘stimuli-responsive’ or ‘smart’ polymers have emerged in recent years. The characteristic feature that makes them smart is their ability to respond to very slight changes in the environment such as temperature [Citation25, Citation26], pH [Citation27, Citation28], electric field [Citation29], light or magnetic field [Citation30, Citation31]. Poly(N-isopropylacrylamide) (PNIPAAm) is the smart polymer that has been studied the most extensively. It is soluble in water below its lower critical solution temperature (LCST; 32 °C) and precipitates at higher temperatures [Citation32, Citation33]. NIPAAm does not have functional groups such as carboxyl, amino or hydroxyl groups; however, these moieties have been introduced by copolymerization to assist further conjugation, substitution or functionalization [Citation34–Citation36]. For example, electrospun NFs from the NIPAAm homopolymer are not stable in water and disperse easily; therefore, copolymerization with crosslinkable co-monomers is required to obtain stable NFs in an aqueous medium [Citation37].
When crosslinking NFs, polymers should be crosslinked after they are ejected from the needle, because electrospinning occurs when the electrical forces at the surface of a polymer solution overcome the surface tension. If the jet dries or solidifies before a voltage is applied, polymers are hardly ejected from the syringe needle. In general, polymer-polymer crosslinking can be carried out in different ways. Physical crosslinking can be accomplished by ionic or hydrophobic interactions [Citation38] although this method can avoid the use of crosslinking agents, the resulting bonding is thermally and mechanically unstable [Citation39]. Chemical crosslinks, on the other hand, are more stable. Carbodiimide is one of the most common crosslinking agents used in the condensation reaction between a carboxylic acid and an amine [Citation40, Citation41]. Another widely investigated crosslinking strategy is Michael addition between a nucleophile (i.e. an amine or a thiol) and a vinyl group [Citation42]. Nowadays, ‘click chemistry’, typified by the condensation reaction between an azido group and a triple bond, is extensively used because the reactions occur at low temperatures, give high yields and are tolerant to various media as they can be carried out in the presence of oxygen and even in water [Citation43, Citation44]. In the case of NIPAAm-based NFs, however, post-crosslinking has to be carried out under dry conditions because these NFs dissolve not only in aqueous media but also in various organic solvents.
With this background, in this study, the post-crosslinking of NIPAAm NFs was accomplished by thermal curing in air. First, thermally crosslinkable poly(NIPAAm-co-N-hydroxymethylacrylamide; HMAAm) was polymerized and fabricated into NFs by electrospinning. HMAAm was chosen because its methylol group can be chemically crosslinked by self-condensation upon heating [Citation45, Citation46]. The copolymers were studied in detail by proton nuclear magnetic resonance (1H-NMR), gel permeation chromatography (GPC) and turbidity measurements. The electrospun NFs were subsequently crosslinked by heat treatment at 110 °C. Attenuated total reflection-Fourier transform infrared (ATR-FTIR) spectroscopy was used to monitor the crosslinking of HMAAm. Scanning electron microscopy (SEM) images of the electrospun NFs revealed a continuous and smooth fibrous morphology with an average fiber diameter of 600–700 nm. The resulting crosslinked NFs showed rapid and reversible size changes in aqueous media in response to cycles of temperature alternation; however, their fibrous morphology was preserved even after the cycles of temperature alternation. Finally, dextran-containing NFs were prepared by electrospinning the copolymers blended with fluorescein isothiocyanate (FITC)-dextran, and the on–off switchable release of FITC-dextran from the crosslinked NFs in response to external cycles of temperature alternation was studied (figure ).
2. Materials and methods
2.1. Materials
NIPAAm was kindly supplied by Kohjin Co. Ltd (Japan) and purified by recrystallization from benzene and n-hexane. HMAAm was purchased from TCI (Japan). 2,2'-azodiisobutyronitrile (AIBN) was purchased from Wako (Japan) and purified from methanol. Phosphate-buffered saline (PBS, pH 7.4) was prepared by mixing 40.5 ml of 0.2 M disodium hydrogen phosphate (Na2HPO4) and 9.5 ml of 0.2 M sodium dihydrogen phosphate (NaH2PO4) in distilled water. FITC-dextran (molecular weight: 3000–5000 g mol−1) was purchased from Sigma-Aldrich (USA). All other reagents and commercial products were used as received without further purification.
2.2. Copolymerization of poly(NIPAAm-co-HMAAm)
Poly(NIPAAm-co-HMAAm) with varying monomer ratios was copolymerized by free-radical polymerization (scheme ). NIPAAm and HMAAm were dissolved in 20 ml N,N-dimethylformamide (DMF) and then 0.01 mol% of AIBN was added to the mixture solution. The total monomer molar concentration was 40 mmol. After the solution was completely degassed by four cycles of freezing and thawing, the copolymerization was carried out for 20 h at 62 °C with mechanical stirring. After the polymerization, the AIBN, unreacted monomers, impurities and solvent were removed by dialysis against ethanol and distilled water for 7 days. The dialyzed solutions were lyophilized for 4 days to obtain the copolymers. The resulting poly(NIPAAm-co-HMAAm) samples are abbreviated to PNH_X, where X is the mole percent of HMAAm.
2.3. Characterization of PNHs
The chemical composition of HMAAm in the PNHs was confirmed by 1H-NMR (JNM-GSX400 spectrometer, 400 MHz, JEOL, Japan). The number average molecular weight (Mn) and polydispersity index (PDI) of the PNHs were determined by GPC (co-2065 plus, JASCO International, Japan), using DMF with 10 mM lithium bromide (LiBr) as an eluent, and calculated using poly(ethylene glycol) (PEG) and polystyrene (PS) standards. The LCSTs of the PNHs were measured by UV-visible spectroscopy (V-550, JASCO International, Japan) in PBS solution (0.1%,w/v), at a heating rate of 1.0 °C min−1. The LCST was defined as the temperature at which transmittance decreased to 50%.
2.4. Electrospinning of nanofibers and thermal crosslinking
PNH NFs were fabricated by electrospinning (Imoto IMC-19F5, Japan) as follows: 0.675 g of PNH was dissolved in 5 ml of 1,1,1,3,3,3 hexafluoro-2-propanol (HFIP) and stirred for 24 h at room temperature. The well-mixed PNH solution was poured into a 6 ml plastic syringe with a metal capillary needle (25 gauge), and the filled syringe was set on a syringe pump operated at a fixed rate of 0.5 ml h−1. A DC voltage of 13 kV was applied between the needle and a collector plate wrapped with aluminum foil, which were separated by a distance of 19 cm. After the onset of electrospinning, the polymer solution was transformed into NFs that were deposited onto the collector. The residual solvent in the electrospun NFs was removed in a vacuum oven. The electrospun NFs were punched into a diameter of 2.2 cm and then carefully peeled off the aluminum foil.
For the preparation of FITC-dextran-incorporated NFs, 3 mg of FITC-dextran was dissolved in 0.3 ml of distilled water and stirred for 24 h at room temperature. The well-mixed FITC-dextran solution was added to 3 ml of HFIP solution containing PNH and then stirred for 24 h. The mixture of FITC-dextran and PNHs was electrospun as described above. For crosslinking, the punched PNH NFs were placed in an oven and treated at 110 °C for 0, 1, 3, 5 or 7 h.
2.5. Characterization of nanofibers
The morphologies of the NFs were observed by SEM (Neoscope JCM-5000, JEOL, Japan) at an acceleration voltage of 10 kV. SEM images were taken after the samples were completely dried and coated with platinum (Pt) for 180 s using an ion coater (SC-701 MK-2, Sanyu Electron, Japan). To confirm the success of intermolecular crosslinking after thermal curing, the degree of self-condensation of the methylol groups of HMAAm was calculated from the disappearance of the methylol groups in NFs by ATR-FTIR spectroscopy (IRPrestige-21, Shimadzu, Japan). The number of reacted methylol groups was calculated from the calibration curve of absorbance at 1050 cm−1 as follows: 1 where I1050, t=0 and I1050, t=X are the absorbance of the NFs at 1050 cm−1 before and after heating at 110 °C for X hours, respectively.
The thermal properties of the crosslinked and non-crosslinked NFs were studied by differential scanning calorimetry (DSC, 6100, Sekio Instruments, Japan). The NFs were soaked in distilled water for 5 min and placed in a DSC cell. The cell was sealed to prevent water evaporation during the measurements, which were conducted between 20 and 60 °C at a heating rate of 5 °C min−1. The phase transition temperature was determined from the inflection point of the endothermal peak (see figure S2 available from stacks.iop.org/STAM/13/064203/mmedia).
2.6. Equilibrium swelling ratio of crosslinked nanofibers
To determine the equilibrium swelling ratio of the crosslinked PNH NFs, the dried NFs were equilibrated at 10 °C in PBS for 5 min. The swollen NFs were collected and immersed in PBS at 45 °C for 5 min. This process was repeated eight times and the weights of swollen or shrunk NFs at each change in temperature were measured. The swelling ratio was calculated using the following equation: 2 where Wswell and Wdry are the weights of the swollen and dry NFs, respectively.
2.7. ‘On–off’ dextran release experiments
The FITC-dextran-containing PNH NFs were first swollen in 1 ml of PBS at 10 °C for 5 min. The temperature of PBS was then alternated between 10 and 45 °C for six cycles. The released FITC-dextran during each cycle was collected, and its absorbance at 493 nm was measured by UV-visible spectroscopy. The FITC-dextran release profile was calculated using the following equation: 3 where Mreleased at X is the cumulative amount of FITC-dextran released during the Xth cycle of temperature alternation and Mtotal is the total amount of FITC-dextran incorporated in the NFs.
3. Results and discussion
3.1. Synthesis and characterizations of PHNs
The PNHs were prepared by free-radical copolymerization with yields exceeding 90%. We prepared copolymers with relatively large molecular weights (>10 000 g mol−1) to obtain uniform NFs [Citation47, Citation48]. The reaction conditions and characterization results are summarized in table .
Table 1. Synthesis details and properties of poly(NIPAAm-co-HMAAm)s.
The composition of HMAAm was determined by 1H-NMR using DMSO-d6 as a solvent (see supplementary information, figure S1 available from stacks.iop.org/STAM/13/064203/mmedia). The methylol and hydroxyl groups of HMAAm were detected at δ = 4.55 ppm and δ = 5.45 ppm, respectively. The HMAAm contents in the PNH_3, 5 and 10 samples were determined to be 3, 5 and 10 mol%, and the Mn values were estimated by GPC to be 12 000, 13 600 and 14 000 g mol−1, respectively. All copolymers had a PDI of approximately 1.9. Figure S2 (supplementary information) shows the temperature dependence of transmittance at 500 nm for the three PNH samples. In this study, the HMAAm content was varied between 3 and 10 mol% to adjust the polymer response to the human body temperature; the corresponding LCST values ranged from 34 to 38 °C.
3.2. Electrospinning of PNHs and thermally induced crosslinking
Generally, the morphology of electrospun fibers (e.g. ultrafine fibers, beads or particles) can be controlled via the electrospinning conditions such as the polymer solution concentration, pumping rate, tip-to-collector distance, solvent and supply voltage [Citation49, Citation50]. Although DMF, THF and ethyl acetate are common electrospinning solvents for various polymers, HFIP was used in this study because it has been suggested in several papers that continuous and smooth NFs can be produced from acrylamide-based copolymers dissolved in HFIP [Citation51]. Indeed, we produced well-defined PNH NFs with average diameters of 600–700 nm, independent of the HMAAm content (figures (a)–(c)). This result is closely related to the electrospinnability of PNHs. Usually, it is very difficult to fabricate continuous, smooth and uniform NFs from polymers having a low molecular weight and a broad distribution of molecular weight [Citation50, Citation52]. Therefore, we synthesized copolymers with relatively large molecular weights (Mn > 10 000 g mol−1) and narrow Mn distributions (PDI < 2), as shown in table . This resulted in uniform NFs with controlled diameters without the formation of beads or particles.
Figure 2 SEM images of NFs electrospun from PNH_3 (a, d), PNH_5 (b, e) and PNH_10 (c, f) solutions before (a–c) and after (d–f) thermal crosslinking at 110 °C for 7 h. Photographs of crosslinked PNH_10 NFs in PBS at 10 °C (g) and 45 °C (h). SEM image of the crosslinked PNH_10 NFs after one cycle of temperature alternation between 10 and 45 °C (i). Scale bars: 5 μm.
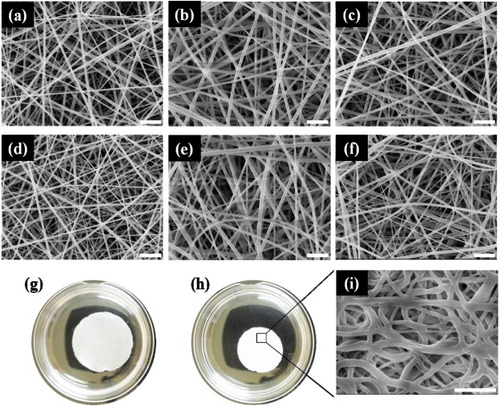
The chemical crosslinking of the electrospun NFs was carried out by thermal curing of the OH groups of HMAAm. The fibers preserved their morphology even after thermal crosslinking at 110 °C for 7 h (figures (d)–(f)). The crosslinked PNH_10 NFs were also stable in aqueous solutions both below and above the LCST (figures (g) and (h)). In figure (i), fibrous structures are still visible in the crosslinked PNH_10 after one cycle of temperature alternation, although the fiber diameters have increased. The crosslinked PNH_5 NFs were also stable in PBS below the LCST (data not shown). The PNH_3 NFs were completely dissolved in PBS after three cycles of temperature alternation. The non-crosslinked NFs dissolved within 30 s in an aqueous medium below the LCST.
The extent of intermolecular crosslinking was calculated from the disappearance of the peaks corresponding to methylol groups in the ATR-FTIR spectra of the NFs. Figure shows the spectra of PNH NFs before crosslinking. The peaks at 1650 and 1550 cm−1 were assigned to amide I (C = O stretching) and amide II (N–H bending) of the copolymer, respectively. The broad absorption band observed around 3430 cm−1 was assigned to the N–H stretching of amide groups in the copolymer. The peaks at 1370–1390 and 2980 cm−1 were assigned to the stretching modes of the –CH(CH3)2 and –(CH3)2 groups in NIPAAm, respectively. The peaks at 1050, 1230 and 3300 cm−1 were assigned to the C–O–H stretching, C–O–H bending and O–H stretching modes in HMAAm, respectively. Among these peaks, the C–O–H stretching peak at 1050 cm−1 was chosen to monitor the crosslinking reaction of HMAAm because the other peaks were either too weak or overlapped with the amide peaks. Figure shows the ATR-FTIR spectra of PNH NFs at different times during the thermal treatment. The signal intensity of methylol groups (1050 cm−1) gradually decreased with increasing thermal treatment time, indicating the formation of bis(methylene ether) and methylene bridges. To evaluate the crosslinking efficiency, we compared the 1050 cm−1 peak for all samples after 7 h of treatment. Approximately 1.5, 2.5 and 5 mol% of methylol groups were involved in the crosslinking process in the PNH_3, PNH_5 and PNH_10 samples, respectively. About 50% of the methylol groups still remained after 7 h of treatment. This is because the mobility of the methylol side groups is restricted as they are tethered onto the polymer side chains, which limits the crosslinking reaction.
Figure 3 ATR-FTIR spectra of PNIPAAm (a), PNH_3 (b), PNH_5 (c) and PNH_10 (d) NFs before crosslinking. The shaded areas around 1050 and 3300 cm−1 are assigned to the stretching of C–O–H and –OH groups in HMAAm, respectively.
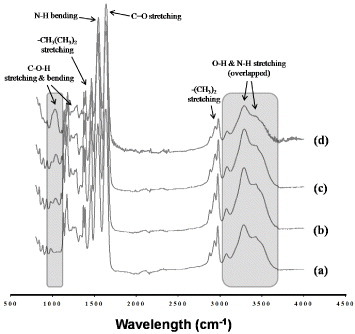
Figure 4 ATR-FTIR spectra of PNH_3 (a), PNH_5 (b) and PNH_10 (c) NFs at different times (0, 1, 3, 5 and 7 h) during the thermal treatment at 110 °C. The shaded areas around 1050 and 3300 cm−1 are assigned to the stretching of C–O–H and –OH groups in HMAAm, respectively.
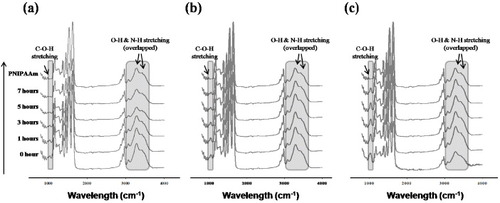
It is difficult to determine the LCSTs for NFs by UV-vis spectroscopy once the fibers are crosslinked; therefore, we measured the thermal properties of the PNHs by DSC. Figures and S3 (available from stacks.iop.org/STAM/13/064203/mmedia) show the LCSTs of the PNHs before and after crosslinking. The LCST increases with increasing HMAAm content, although the obtained values are slightly higher than those obtained by UV-vis spectroscopy. The LCST decreased after crosslinking because fewer hydrophilic hydroxyl groups remained in the PNHs.
3.3. Swelling and shrinking of the crosslinked nanofibers
Generally, it is difficult to observe the temperature-responsive dynamic behavior of nanoscale objects. In this study, however, the NFs were stably crosslinked and could be manipulated as a bulk material. Therefore, the temperature-responsive behavior of the NFs was easily characterized by observing their swelling and shrinking in response to temperature cycling between 10 and 45 °C (figure ). The NFs were incubated at each temperature for 5 min, because they shrank completely within 60 s (figure S5 available from stacks.iop.org/STAM/13/064203/mmedia), and this process was repeated for eight cycles. The swelling ratios for the crosslinked PNH_3 NFs at 10 °C were smaller than those for the other NFs even though the PNH_3 NFs had the lowest crosslinking density. This is because the PNH_3 NFs gradually came apart and dissolved in water after three cycles of temperature alternation owing to insufficient crosslinking. In contrast, the PNH_5 and PNH_10 NFs demonstrated temperature-responsive swelling and shrinking for at least eight cycles without weight loss. This result suggests that more than 1.5 mol% of methylol groups is needed for the stable crosslinking of NFs. The PNH_5 and PNH_10 NFs responded quickly to changes in temperature, and their swelling ratio recovered within each cycle. In general, the application of bulk materials such as hydrogels is limited by their slow response, and several techniques have been proposed to increase the rate of response, such as reducing the gel size and constructing an interconnected pore structure within the gel [Citation53]. Because of the high specific surface area of NFs, they are more sensitive to external stimuli than the corresponding bulk materials. From these results, the PNH_5 and PNH_10 NFs were chosen to investigate the possibility of ‘on–off’ dextran release because they are stable and sensitive to temperature changes in aqueous media.
3.4. Release of FITC-dextran from NFs
An ‘on–off’ drug-delivery system offers rapid and transient drug release, and delivers the drug at an appropriate time and dosage. Various technologies have been used to demonstrate the ‘on–off’ controlled release of drugs using stimuli-responsive polymers [Citation27, Citation54]. The fabrication of stimuli-responsive polymers in NFs is attractive because of the resulting large and tunable surface area and porosity [Citation55, Citation56]. Moreover, crosslinked NFs can be shaped into bulk materials. Therefore, we investigated the possibility of the ‘on–off’ drug release using the crosslinked temperature-responsive NFs. As a model drug, we used FITC-dextran, which has good water solubility and is becoming increasingly important for drug delivery [Citation57–Citation59]. FITC-dextran-incorporated PNH NFs were electrospun by simply blending FITC-dextran and PNHs in HFIP as a solvent.
Figure S4 (supplementary information) shows representative SEM images of the FITC-dextran-incorporated PNH_10 NFs before and after crosslinking. A nanosized fibrous morphology was clearly observed even though a mixture of HFIP and distilled water was used as the solvent for electrospinning. The crosslinked PNH NFs were first swollen in PBS at 10 °C for 5 min. The temperature was then alternated between 10 and 45 °C, and the amount of FITC-dextran released during each cycle was measured by UV-visible spectroscopy. The results are shown in figure . After the first heating, approximately 30% of the loaded dextran was released from the PNH_10 NFs. The release stopped after cooling to 10 °C but restarted upon the second heating. In this system, the dextran is released by it being squeezed out of the collapsing polymer network. The release of dextran stops upon cooling because of the suppressed diffusion of the FITC-dextran molecules, which have higher molecular weights. Almost all the dextran was released after six temperature cycles for the PNH_5 and PNH_10 NFs. Judging from the obtained dextran release profiles, the proposed system can release a certain amount of a drug within a short time after an off period that can be programmed according to the circadian rhythm of the disease being treated. Such a system can be used for the optimal therapy of chronic diseases such as asthma, hypertension, myocardial infarction and arthritis, which show circadian variation.
4. Conclusions
Thermally crosslinkable and temperature-responsive poly(NIPAAm-co-HMAAm) NFs have been fabricated by electrospinning to realize the ‘on–off’ controllable release of dextran. Approximately 50% of the methylol groups of HMAAm in the polymer were crosslinked by thermal curing without altering the fiber morphology. The LCSTs of the fabricated NFs were close to human body temperature. The crosslinked NFs exhibited rapid swelling and shrinking in response to cycles of temperature alternation across the LCST. Dextran-loaded NFs with a continuous and smooth fibrous structure were fabricated, and the ‘on–off’ switchable release of dextran from the NFs was observed. Almost all the dextran was released from the NFs after six heating cycles, whereas a negligible amount of dextran was evolved during the cooling process. The reported incorporation of smart properties into NFs takes advantage of their extremely large surface area and porosity and is expected to provide a simple platform for ‘on–off’ drug delivery.
Acknowledgments
The authors would like to express their gratitude for a Grant-in-Aid for Young Scientists (A) (10013481) from the Ministry of Education, Culture, Sports, Science and Technology (MEXT), Japan. The authors are grateful to Professor Allan S. Hoffman (University of Washington) and Dr. John M. Hoffman (Stratos Genomics Inc. in USA) for continued and valuable discussions.
References
- HuangZ-MZhangY-ZKotakiMRamakrishnaS 2003 Compos. Sci. Technol. 63 2223 10.1016/S0266-3538(03)00178-7
- BarhateR SRamakrishnaS 2007 J. Membr. Sci. 296 1 10.1016/j.memsci.2007.03.038
- GopalRKaurSFengC YChanCRamakrishnaSTabeSMatsuuraT 2007 J. Membr. Sci. 289 210 10.1016/j.memsci.2006.11.056
- JiaHZhuGVugrinovichBKataphinanWRenekerD HWangP 2002 Biotechnol. Prog. 18 1027 10.1021/bp020042m
- Vo-DinhTCullumB MStokesD L 2001 Sensors Actuators B 74 2 10.1016/S0925-4005(00)00705-X
- WangXDrewCLeeS-HSenecalK JKumarJSamuelsonL A 2002 Nano Lett. 2 1273 10.1021/nl020216u
- RhoK SJeongLLeeGSeoB-MParkY JHongS-DRohSChoJ JWonW HMinB-M 2006 Biomaterials 27 1452 10.1016/j.biomaterials.2005.08.004
- MinB-MLeeGKimS HNamY SLeeT SParkW H 2004 Biomaterials 25 1289 10.1016/j.biomaterials.2003.08.045
- YoshimotoHShinY MTeraiHVacantiJ P 2003 Biomaterials 24 2077 10.1016/S0142-9612(02)00635-X
- KuS HParkC B 2010 Biomaterials 31 9431 10.1016/j.biomaterials.2010.08.071
- MaretschekSGreinerAKisselT 2008 J. Controll. Release 127 180 10.1016/j.jconrel.2008.01.011
- TungprapaSJangchudISupapholP 2007 Polymer 48 5030 10.1016/j.polymer.2007.06.061
- TsengR JHuangJOuyangJKanerR BYangY 2005 Nano Lett. 5 1077 10.1021/nl050587l
- KongHJangJ 2008 Langmuir 24 2051 10.1021/la703085e
- SungJ HKimH SJinH-JChoiH JChinI-J 2004 Macromolecules 37 9899 10.1021/ma048355g
- McCullenS DStevensD RRobertsW AOjhaS SClarkeL IGorgaR E 2007 Macromolecules 40 997 10.1021/ma061735c
- LiDWangYXiaY 2003 Nano Lett. 3 1167 10.1021/nl0344256
- KimB JChoiY SChaH J 2012 Angew. Chem. Int. Ed. 51 675 10.1002/anie.201105789
- KimT GParkT G 2006 Tissue Eng. 12 221 10.1089/ten.2006.12.221
- YePXuZ-KWuJInnocentCSetaP 2006 Biomaterials 27 4169 10.1016/j.biomaterials.2006.03.027
- McCannJ TLiDXiaY 2005 J. Mater. Chem. 15 735 10.1039/b415094e
- HuangZ-MHeC-LYangAZhangYHanX-JYinJWuQ 2006 J. Biomed. Mater. Res. A 77 169
- LohX JPehPLiaoSSngCLiJ 2010 J. Controll. Release 143 175 10.1016/j.jconrel.2009.12.030
- SongMGuoDPanCJiangHChenCZhangRGuZWangX 2008 Nanotechnology 19 165102 10.1088/0957-4484/19/16/165102
- StaytonP SShimobojiTLongCChilkotiAChenGHarrisJ MHoffmanA S 1995 Nature 378 472 10.1038/378472a0
- YoshidaRUchidaKKanekoYSakaiKKikuchiASakuraiYOkanoT 1995 Nature 374 240 10.1038/374240a0
- GuptaPVermaniKGargS 2002 Drug Discov. Today 7 569 10.1016/S1359-6446(02)02255-9
- TechawanitchaiPEbaraMIdotaNAsohT-AKikuchiAAoyagiT 2012 Soft Matter 8 2844 10.1039/c2sm07277g
- KwonI CBaeY HKimS W 1991 Nature 354 291 10.1038/354291a0
- SuzukiATanakaT 1990 Nature 346 345 10.1038/346345a0
- LaiJ JHoffmanJ MEbaraMHoffmanA SEstournesCWattiauxAStaytonP S 2007 Langmuir 23 7385 10.1021/la062527g
- SchildH G 1992 Prog. Polym. Sci. 17 163 10.1016/0079-6700(92)90023-R
- EbaraMYamamotoMAoyagiTKikuchiASakaiKOkanoT 2008 Adv. Mater. 20 3034 10.1002/adma.200702308
- EbaraMAoyagiTSakaiKOkanoT 2000 Macromolecules 33 8312 10.1021/ma000121j
- MaedaTTakenouchiMYamamotoKAoyagiT 2006 Biomacromolecules 7 2230 10.1021/bm060261m
- KotsuchibashiYEbaraMIdotaNNarainRAoyagiT 2012 Polym. Chem. 3 1150 10.1039/c2py00589a
- KimY-JEbaraMAoyagiT 2012 Angew. Chem. Int. Ed. at press. DOI: 10.1002/anie.201204139
- BhattaraiNZhangM 2007 Nanotechnology 18 455601 10.1088/0957-4484/18/45/455601
- OkuzakiHKobayashiKYanH 2009 Macromolecules 42 5916 10.1021/ma9014356
- KimH-WSongJ-HKimH-E 2005 Adv. Funct. Mater. 15 1988 10.1002/adfm.200500116
- ZhangSHuangYYangXMeiFMaQChenGRyuSDengX 2009 J. Biomed. Mater. Res. A 90 671
- MatherB DViswanathanKMillerK MLongT E 2006 Prog. Polym. Sci. 31 487 10.1016/j.progpolymsci.2006.03.001
- FuG DXuL QYaoFZhangKWangX FZhuM FNieS Z 2009 Appl. Mater. Interfaces 1 239 10.1021/am800143u
- CrownoverEDuvallC LConvertineAHoffmanA SStaytonP S 2011 J. Controll. Release 155 167 10.1016/j.jconrel.2011.06.013
- YocumR HNyquistE B 1973 Functional Monomers New York Marcel Dekker
- KrishnanSKleinAEl-AasserM SSudolE D 2003 Macromolecules 36 3511 10.1021/ma021121h
- ShenoyS LBatesW DFrischH LWnekG E 2005 Polymer 46 3372 10.1016/j.polymer.2005.03.011
- BuchkoC JChenL CShenYMartinD C 1999 Polymer 40 7397 10.1016/S0032-3861(98)00866-0
- TheronS AZussmanEYarinA L 2004 Polymer 45 2017 10.1016/j.polymer.2004.01.024
- TanS-HInaiRKotakiMRamakrishnaS 2005 Polymer 46 6128 10.1016/j.polymer.2005.05.068
- KonosuYMatsumotoHTsuboiKMinagawaMTaniokaA 2011 Langmuir 27 14716 10.1021/la203396y
- KoskiAYimKShivkumarS 2004 Mater. Lett. 58 493 10.1016/S0167-577X(03)00532-9
- WangJSuttiAWangXLinT 2011 Soft Matter 7 4364 10.1039/c1sm00010a
- ChungJ EYokoyamaMYamatoMAoyagiTSakuraiYOkanoT 1999 J. Controll. Release 62 115 10.1016/S0168-3659(99)00029-2
- FuG-DXuL-QYaoFLiG-LKangE-T 2009 Appl. Mater. Interfaces 1 2424 10.1021/am900526u
- ChenMDongMHavelundRReginaV RMeyerR LBesenbacherFKingshottP 2010 Chem. Mater. 22 4214 10.1021/cm100753r
- JiangHFangDHsiaoB SChuBChenW 2004 Biomacromolecules 5 326 10.1021/bm034345w
- MengFZhongZFeijenJ 2009 Biomacromolecules 10 197 10.1021/bm801127d
- KikuchiAKawabuchiMWatanabeASugiharaMSakuraiYOkanoT 1999 J. Controll. Release 58 21 10.1016/S0168-3659(98)00141-2