Abstract
We newly designed super-elastic biodegradable scaffolds with longitudinally oriented microchannels for repair and regeneration of peripheral nerve defects. Four-armed poly(ε-caprolactone-co-D,L-lactide)s (P(CL-co-DLLA)s) were synthesized by ring-opening copolymerization of CL and DLLA from terminal hydroxyl groups of pentaerythritol, and acryloyl chloride was then reacted with the ends of the chains. The end-functionalized P(CL-co-DLLA) was crosslinked in a cylindrical mold in the presence of longitudinally oriented silica fibers as the templates, which were later dissolved by hydrofluoric acid. The elastic moduli of the crosslinked P(CL-co-DLLA)s were controlled between 10−1 and 102 MPa at 37 °C, depending on the composition. The scaffolds could be elongated to 700% of their original size without fracture or damage (‘super-elasticity’). Scanning electron microscopy images revealed that well-defined and highly aligned multiple channels consistent with the mold design were produced in the scaffolds. Owing to their elastic nature, the microchannels in the scaffolds did not collapse when they were bent to 90°. To evaluate the effect of the channel diameter on Schwann cell migration, microchannels were also fabricated in transparent poly(dimethylsiloxane), allowing observation of cell migration. The migration speed increased with channel size, but the Young's modulus of the scaffold decreased as the channel diameter increased. These findings may serve as the basis for designing tissue-engineering scaffolds for nerve regeneration and investigating the effects of the geometrical and dimensional properties on axonal outgrowth.
1. Introduction
Peripheral nerve injuries caused by trauma and iatrogenic injury are a worldwide problem and can result in a significant, life-long disability [Citation1]. Although nerves can regenerate on their own if injuries are small, effective nerve regeneration and functional recovery subsequent to a larger injury are still a clinical challenge. The standard treatment of peripheral nerve injury involves direct end-to-end surgery of the damaged nerve ends or the use of an autologous nerve graft [Citation2, Citation3]. Autologous nerve graft transplantation is a feasible treatment for the injury across gaps greater than a few millimeters, but limited by donor site morbidity and insufficient donor tissue, impairing a complete functional recovery [Citation4, Citation5]. Thus, bioengineering strategies for the peripheral nervous system are focused on alternatives to the nerve graft. Tissue engineering has introduced innovative approaches to promote and guide peripheral nerve regeneration by using biodegradable materials [Citation6–Citation8]. Synthetic materials are attractive because their chemical and physical properties such as degradation rate, porosity and mechanical strength can be optimized for a particular application [Citation9]. A number of synthetic materials have been explored for use in aiding nerve regeneration [Citation4, Citation6, Citation10]. They are mainly based on the polyesters, such as poly(glycolide) (PGA), poly(L-lactide) (PLLA), poly(L-lactide-co-glycolide) (PLGA), poly(ε-caprolactone) (PCL) and poly(3-hydroxybutyrate) (PHB) because of their availability, biodegradability and approval by the Food and Drug Administration (FDA). Toba et al [Citation11] designed a bioabsorbable PGA tube filled with collagen sponge (PGA–collagen tube) as a nerve connective guide for nerve regeneration. The PGA–collagen conduit supported the nerve repair and functional recovery after grafting into an 80 mm gap in a nerve injury in a dog [Citation12, Citation13]. They have also developed a nerve guide tube created by braiding together PLLA and PGA for repair of long nerve defects. By introducing the slowly decomposing PLLA, better regeneration was achieved compared to that of PLGA alone [Citation14]. On the other hand, the nerve guides composed of a semicrystalline copolymer of ε-caprolactone and L-lactide (P(CL-co-LLA)) were found to degrade slowly, leaving significant amounts of biomaterial around the regenerated nerve after 2 years and causing a chronic foreign body reaction with formation of scar tissue [Citation15–Citation17]. To overcome this problem, copolyester of ε-caprolactone and D,L-lactide (DLLA, replacing LLA) were developed to obtain a more amorphous scaffold with controllable degradation properties [Citation18, Citation19]. Nerve guides constructed of P(CL-co-DLLA) possessed favorable properties for bridging a 10 mm gap in the sciatic nerve of the rat [Citation20, Citation21], and resulted in better functional recovery compared to autologous nerve grafts [Citation22, Citation23]. Despite a plethora of nerve prostheses developed, however, few approaches entered the clinical practice [Citation24, Citation25].
In the case of peripheral nerve regeneration and tissue engineering, evidence obtained from both in vitro and in vivo studies indicates that Schwann cells (SCs) are crucial and play both structural and functional roles [Citation26]. When a peripheral nerve is damaged, SCs alter their behavior to become involved in Wallerian degeneration and Büngner bands [Citation27–Citation30]. In Wallerian degeneration, SCs grow in ordered columns along the endoneurial tube, creating a Büngner band that protects and preserves the endoneurial channel. SCs also elaborate neurotrophic substances that enhance regrowth in conjunction with macrophages after peripheral nerve injury [Citation6]. Additionally, SCs express integrin surface proteins that interact with surface proteins at the growth cone of regenerating axons. When SCs were pre-seeded into nerve guidance channels, the injured peripheral nerves regenerated at a faster rate and over longer distances than without pre-seeding [Citation31, Citation32]. From these perspectives, appropriate synthetic materials for artificial conduits must (1) be readily formed into a conduit with desired dimensions to support SC migration, (2) be pliable and easy to handle and suture, but should maintain their shape and resist collapse during implantation, and (3) protect the regenerating axons in the lumen from the environment, but should be porous and semipermeable to provide a conduit with the diffusion of biomolecules such as growth factors.
To design promising artificial conduits, here we describe a novel technique for preparing ‘super-elastic’ scaffolds, which are composed of biodegradable P(CL-co-DLLA)s with longitudinal microchannels and have an optimized channel geometry for migration of SCs. We define the term ‘super-elastic’ as follows: (1) the elastic modulus is lower than 5 MPa, and (2) the maximum strain is higher than 500%. First, end-functionalized four-armed P(CL-co-DLLA) macromonomers with various CL/DLLA compositions were synthesized according to the previously reported protocol (scheme ). The macromonomers were then crosslinked in a cylindrical mold containing uniformly spaced silica capillary fibers. The silica fibers were later dissolved with hydrofluoric acid (HF). Their thermal and mechanical properties have been characterized by differential scanning calorimetry (DSC) and tensile tests, respectively. The produced channels within the scaffolds were observed by scanning electron microscopy (SEM). To evaluate the effect of the channel diameters on SC migration, microchannels were also fabricated in transparent poly(dimethylsiloxane) (PDMS). SCs were plated onto poly-L-lysine-coated cell culture dish covered with PDMS microchannels, and the migration distances were evaluated.
Scheme 1 Preparation schemes of pliable biodegradable tubes with microchannels. Four-armed P(CL-co-DLLA)s are synthesized by ring-opening copolymerization of CL and DLLA from terminal hydroxyl groups of pentaerythritol. Acryloyl chloride is then reacted with the ends of the chains. The end-functionalized P(CL-co-DLLA) is crosslinked in a cylindrical mold in the presence of longitudinally oriented silica fibers as the templates, which are later dissolved with HF. SEM images of the silica fibers (a) and the crosslinked P(CL-co-DLLA) before (b) and after etching (c).
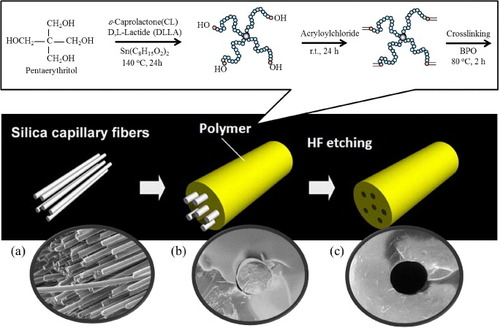
2. Materials and methods
2.1. Materials
ε-Caprolactone was purchased from Tokyo Kasei (Tokyo, Japan) and purified by distillation over calcium hydride, which was purchased from Wako Pure Chemical Industries (Tokyo, Japan), under reduced pressure. Pentaerythritol and acryloyl chloride were also purchased from Tokyo Kasei and used as received. DLLA and LLA were kindly supplied by the Musashino Chemical Laboratory (Tokyo, Japan) and recrystallized twice from ethyl acetate before use. Triethylamine was purchased from Wako Pure Chemical Industries, Ltd, and dehydrated by distillation over potassium hydroxide. Tin octanoate, HF solution (46%) and other chemicals were also purchased from Wako Pure Chemical Industries, Ltd. Benzoyl peroxide (BPO) was purchased from Sigma (St Louis, Missouri, US) and used as received. PDMS prepolymer was purchased from Dow Corning Corporation (Midland, Michigan, US). Negative photoresist SU8-50 was purchased from Microchem Corporation (Massachusetts, US).
2.2. Synthesis of four-armed macromonomers
Four-armed copolymers with different CL and DLLA compositions were synthesized by ring opening copolymerization from terminal hydroxyl groups of pentaerythritol using tin octanoate as a catalyst according to our previous reports [Citation18, Citation33–Citation35]. Acryloyl chloride was then reacted with the ends of the branched chains. Four-armed PLLA macromonomer was also synthesized using the same protocol. The structures and the molecular weights were estimated by proton nuclear magnetic resonance (1H NMR) spectroscopy (JEOL, Tokyo, Japan) and gel permeation chromatography (GPC, JASCO International, Tokyo, Japan).
2.3. Preparation of crosslinked materials with microchannel structures
Silica capillary fibers with various diameters (8, 150, 350 and 660 μm) were inserted in a glass tube of 1.5 mm inner diameter, and a xylene solution containing the four-armed macromonomers (40 wt%) and BPO (1.5 wt%) was injected. The macromonomer solution was then cured at 80 °C for 2 h. After the reaction, the crosslinked samples were removed from the glass tube and immersed in HF solution at room temperature for 24 h to dissolve the silica templates. Films for a tensile test were prepared by crosslinking the macromonomers between glass slides with a 0.2 mm thick Teflon spacer.
2.4. Characterizations
The thermal properties of the macromonomers before and after crosslinking were measured by DSC (DSC6100, Seiko Instruments, Chiba, Japan). The measurements were conducted from 0 to 120 °C at a heating rate of 5 °C min−1. The mechanical properties of the crosslinked films were characterized with a tensile tester (EZ-S 500N, Shimadzu, Kyoto, Japan) equipped with a heating chamber (Chromato chamber M-600FN, TAITEC, Saitama, Japan). The tensile tests were carried out at an elongation rate of 10 mm min−1 at various temperatures, and the elastic modulus was calculated from the initial slope of the stress–strain curve. The morphologies of the channels produced in the crosslinked tube were observed by SEM (JCM-5000, JEOL).
2.5. Fabrication of PDMS microchannels
To fabricate microchannels, PDMS prepolymer was cast against the patterned silicon master and cured at 60 °C for 3 h [Citation36, Citation37]. PDMS prepolymer was prepared by mixing PDMS base with a curing agent in a 10:1 ratio by weight and degassing the mixture under vacuum. Patterned silicon master was fabricated by photolithography. Briefly, a silicon wafer was spin-coated with SU8-50 and baked at 95 °C for 1 h. The photoresist was exposed to UV light (SUSS MicroTec MA6) for 15 s thorough transparent masks. After exposure, the masters were baked at 95 °C for 10 min and developed with SU-8 developer (Microchem). Patterned masters were passivated by 10 min exposure to silane under vacuum.
2.6. Migration assay of Schwann cells using PDMS channels
Primary rat Schwann cells were isolated from sciatic nerves of 4- to 5-day-old Wistar rats and cultured in Dulbecco's modified Eagle's medium (DMEM) containing 10% fetal bovine serum (FBS). The following day, 10 μm cytosine arabinoside (AraC) was added to the medium to eliminate dividing fibroblasts for 48 h. The cells were grown in DMEM containing 3% FBS with 3 μm forskolin and 20 ng ml−1 neuregulin. PDMS microchannels were attached to the 6-well-dishes coated with poly-L-lysine. The medium was added to the dish, and air in the gutter on the surface of PDMS microchannels was aspirated. Schwann cells were plated at a density of 1 × 104 cells cm−2. The migration length of Schwann cells into the gutter was observed daily for 12 days.
3. Results and discussion
3.1. Preparation and characterization of P(CL-co-DLLA) scaffolds
PCL is a semicrystalline solid and an important class of biodegradable polymers. The mobility of its chains and the crystallinity markedly change at the melting temperature (Tm, see figure (a)). Crosslinked PCL is relatively elastic above Tm [Citation38, Citation39]; however, the Tm value (∼60 °C) is too high for biological applications. We have previously reported novel techniques to control the Tm of PCL by tailoring the number of branched chains [Citation39, Citation40] or incorporating non-crystalline segments [Citation18, Citation33–Citation35]. In this study, the Tm was adjusted below the human body temperature by copolymerizing CL with DLLA. Four-armed P(CL-co-DLLA) copolymers with various CL/DLLA ratios were synthesized by ring-opening polymerization from pentaerythritol. The obtained copolymers were then reacted with acryloyl chloride to introduce vinyl groups at the end chains. The copolymer properties are summarized in table . All the copolymers possessed relatively narrow molecular weight distributions (Mw/Mn = 1.32–1.57) as determined by GPC. The DLLA contents of the copolymers were determined by 1H-NMR as 0, 9, 19, 29 and 39 mol% DLLA when the feed concentrations were 0, 10, 20, 30 and 40 mol%, respectively. These results indicate that copolymers with the desired branch number, molecular weights and compositions of CL and DLLA have been obtained.
Figure 1 (a) Schematic of crystal–amorphous transition of semicrystalline PCL. (b) Effect of DLLA content in crosslinked P(CL-co-DLLA) on the melting temperature (Tm) determined by DSC.
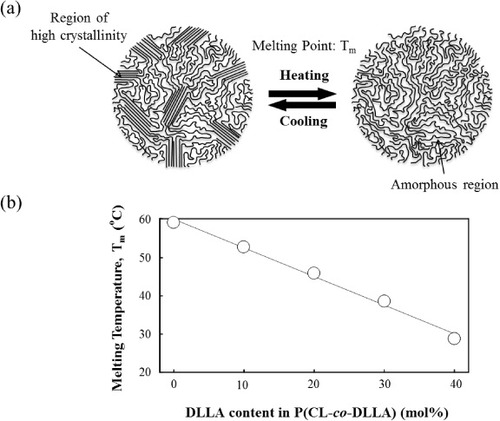
Table S1. Properties of four-armed P(CL-co-DLLA).
Figure (b) and table show the effect of DLLA content on the parameters of crosslinked P(CL-co-DLLA). Both the Tm and melting enthalpy (ΔH, table in the supporting information) decrease with the DLLA content. The Tm of the copolymer with 30 mol% of DLLA (70/30), for example, is close to the body temperature (∼39 °C), whereas that of the original PCL homopolymer (100/0) is 59 °C. Even lower Tm (∼29 °C) was achieved for the 60/40 sample. These results indicate that incorporation of amorphous DLLA units prevents crystallization of PCL [Citation41, Citation42]. All samples were chemically crosslinked and therefore retained their original shape even above Tm. Thus the crosslinked PCL copolymers with Tm near or lower than 37 °C can be used as an elastic biodegradable scaffold in the human body.
Figure shows temperature dependences of Young's modulus for the crosslinked copolymers. Young's modulus was determined from the initial slope of the stress–strain curve recorded during the tensile test. The Young's moduli of crosslinked PLLA, which is one of the most promising FDA-approved materials for nerve tissue engineering [Citation43–Citation45], varied between 650 and 830 MPa. Those values were independent of temperature in the range 20–45 °C because PLLA has a higher Tm (around 143 °C). The crosslinked P(CL-co-DLLA) samples, on the other hand, show significantly lower values compared with PLLA. Their Young's moduli markedly decreased as temperature increased. The transition temperatures are consistent well with the Tm obtained from DSC measurements (as shown in figure (b)). For 60/40 samples, Young's moduli of 11.0 ± 0.5 MPa, 370 ± 60 and 80 ± 30 kPa were measured at 20, 30 and 40 °C, respectively; that is, these samples are relatively durable (>10 MPa) at room temperature and are elastic (<0.1 MPa) at body temperature.
3.2. Fabrication and characterization of microchannel structures in the scaffolds
It has been widely accepted that not only biocompatibility, biodegradability and mechanical properties but also the three-dimensional architectures such as porosity and pore interconnectivity in the scaffolds are important for tissue engineering applications. In particular, scaffolds with structural features similar to neural structures can be more effective in the reconstruction process. Valmikinathan et al [Citation46] reported that tubular structures enhanced the Schwann cell attachment and proliferation compared with non-tubular structures. Many other reports have also suggested that in a scaffold with microtubular channel architecture, regenerating axons can extend more efficiently through open longitudinal than randomly oriented channels [Citation47–Citation50]. Those oriented channels are usually created by inserting a needle or wire in a polymer scaffold. After the shape is stabilized, the needle or wire is removed to form the channels. Wang et al [Citation51] developed a molding technique to produce multichannel scaffolds using acupuncture needles as mandrels. Huang et al [Citation52] created longitudinal channels in chitosan scaffolds using nickel–copper wires. Another way to create longitudinal channels is to create a conduit from one polymer with longitudinally embedded fibers from another polymer, and then selectively dissolve the fibers to form longitudinal channels. Flynn et al [Citation53] developed a method of creating longitudinal channels within hydrogels using PCL fibers as the template.
In this study, we fabricated multiple channels in P(CL-co-DLLA) scaffolds using a silica fiber templating technique as shown in scheme 1. This fabrication technique is simple and reproducible. It allows to control dimensions because silica capillary fibers have a well-defined structure with a wide range of diameters and can be easily dissolved in an HF solution. Silica capillary fibers were inserted in a glass tube and the P(CL-co-DLLA) solution was injected. After crosslinking, the fibers were removed by dissolution resulting in longitudinal fiber-free channels uniformly distributed in the scaffold. Figure (a) shows SEM images of microchannels produced in the 70/30 P(CL-co-DLLA) using silica templates. Average diameters of the individual channels were measured from the SEM images (figure (b)). Templates 8, 150, 350 and 660 μm in diameter created channels with diameters of 8.3 ± 0.6, 130.0 ± 18.0, 338.3 ± 7.6 and 605.0 ± 5.0 μm, respectively. Because the scaffolds shrank during drying and crystallization after the crosslinking reaction, the final channel diameters were slightly smaller than those of the templates. The duplicated channel-shapes were observed in cross-sectional images at different positions (data not shown). These results reveal that the silica templating technique yielded well-defined, longitudinally oriented, fiber-free channels in P(CL-co-DLLA) scaffolds.
Figure 3 (a) Representative SEM images of microchannel structures produced in P(CL-co-DLLA) (70/30) scaffolds using silica templates with diameters 8, 150, 350 and 660 μm. (b) Relationship between diameters of silica templates and channels created in the scaffolds.
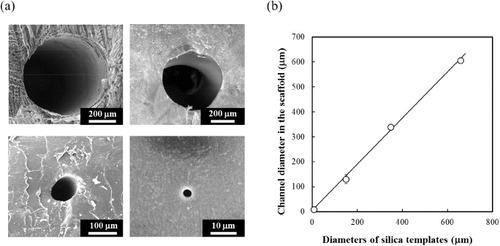
Next, the pliability of crosslinked scaffold was evaluated. Bending tests were performed in cylindrical tubes with 350 μm inner diameter to measure the deformations in the tubes in the bent regions. The optical micrographs of bent P(CL-co-DLLA) (70/30) and PLLA are shown in figure (a). The samples were bent to 90° at 37 °C and sliced at the bending point. Cross-sectional images revealed that the channel structure in P(CL-co-DLLA) tube did not collapse, and the shape and size were consistent before and after bending (figure (b) left). However, the channel in the PLLA tube was significantly deformed by bending the tube (figure (b) right). The deformation of the inner channel was also observed by a replica molding technique. PDMS prepolymer solution was injected into the channel and the tube was bent to 90° at 37 °C. After PDMS was cured, the PDMS replica was removed from the channel. Figure (c) shows the cross-sectional images of the PDMS replicas at the bending point. The deformations of the replicas were almost identical to those of the corresponding channels observed in figure (b). The different pliability between P(CL-co-DLLA) and PLLA does correlate with the elastic modulus for each sample. As shown in figure , the Young's modulus of the 70/30 polymer was several hundred times smaller than that of PLLA at 37 °C. Moreover, P(CL-co-DLLA) has a larger elastic deformation range where the object returns to its original shape, as does rubber. The 70/30 sample recovered its shape after elongation up to 700%, whereas PLLA broke at 50% of strain, which is in the irreversible plastic deformation range for PLLA. These results clearly indicate that the crosslinked P(CL-co-DLLA) has great potential as a new class of pliable scaffold, particularly for regeneration of soft tissues.
Figure 4 Optical (a) and electron (b,c) micrographs of P(CL-co-DLLA) (70/30) (left) and PLLA (right) tubes with inner diameters of 350 μm. (a) The samples were bent to 90° at 37 °C. Cross-sectional images of the tubes (b) and PDMS replica (c) at the bending point.
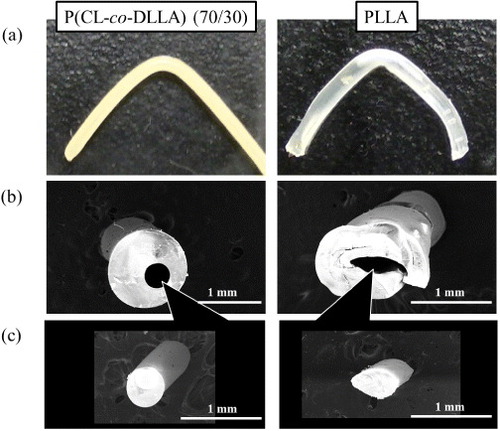
The effect of channel diameter on mechanical properties of P(CL-co-DLLA) tube was also evaluated by tensile test at 37 °C. To eliminate the effect of porosity, the total porosity in the scaffold was fixed at approximately 38% by varying the number of channels. For example, for templates with diameters of 660, 350 and 150 μm, 2, 7 and 39 channels were created in the scaffold, respectively. Figure compares the Young's modulus and strain at break for P(CL-co-DLLA)s (70/30) containing channels of various diameters. At equal porosity, the Young's modulus decreased from 1.6 MPa to 300 kPa with increasing diameter of the individual channel from 150 to 660 μm. The 150 and 350 μm-scaffolds, however, withstood up to 1 N mm−2 of stress, whereas the 660 μm-scaffold broke at 0.41 N mm−2. These results may be attributed to the uniformity of channel distributions, i.e. smaller channels were distributed more uniformly in the scaffold. In addition, larger pores may cause cracks at lower stress, leading to an unstable fracture. Wen et al [Citation54] reported that the Young's modulus of porous magnesium scaffolds decreased with increasing pore size, where the porosity was approximately 45%. Miyoshi et al [Citation55] also reported that the mechanical properties of porous aluminum were related to not only the porosity but also the pore structure. These observations indicate that higher aspect ratios of the wall thickness against the channel edge length result in better energy absorption under applied stress. Therefore, the scaffold with 660 μm channels was the most brittle in this study. Most importantly, these results indicate that the mechanical properties of P(CL-co-DLLA) scaffolds could be controlled not only by Tm but also by the channel structures. From our results, the 350 μm scaffold is the most elastic but reliable material among samples tested in this study.
Figure 5 The effects of channel diameter on Young's modulus and stress at break of P(CL-co-DLLA) (70/30) at 37 °C. All samples possess the same 38% porosity which was adjusted by the number of templates: for 150, 350 and 660 μm diameters, 39, 7 and 2 channels were created in the scaffold, respectively.
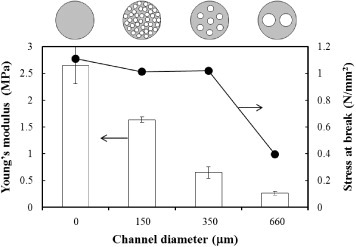
3.3. Effect of channel size on Schwann cells migration
Several studies reported that conduits with longitudinal channels demonstrated better regeneration across long peripheral nerve gaps than hollow conduits in vivo [Citation56, Citation57]. Since longitudinal channels approximate the microarchitecture of native peripheral nerves, the luminal surfaces can promote the adherence and migration of SCs, whose presence is known to play a key role in nerve regeneration. However, there have been few reports on the effect of channel size on SC migration in vitro, and the optimal pore size for SC migration is not fully understood. The optimal pore size usually depends on the cell type. Endothelial cells, for example, show favorable attachment to pores in the range of 20–80 μm, whereas osteoblasts require pores larger than 10 0μm for bone formation [Citation58, Citation59]. A series of PCL copolymers showed good cell compatibility with contact angles in the range of 50–70° depending on the composition [Citation33, Citation34, Citation40]. However, SCs hardly attach on surfaces without coating with poly-L-lysine or laminine. Accordingly, we performed in vitro migration assay for SCs using poly-L-lysine-coated TCPS surfaces covered with microchannels for better understanding of the effect of pore size on the SC migration. The migration assay was carried out in two-dimensional system instead of three-dimensional scaffold to eliminate the effect of curvature (figure (a)). The channels were fabricated in PDMS instead of P(CL-co-DLLA) because the transparency of PDMS enables direct observation of cell migration. A cell culture surface coated with poly-L-lysine was covered with PDMS containing gutters of various widths and heights. Figure (b) shows the effect of channel width on cell migration distance. The channels were 80 μm tall and 30, 70, 150 and 350 μm wide. More SCs migrated away from the edge in wider channels than in narrower ones. Likewise, the migration distance of SCs after 12 days was much longer in the channel with internal width of 350 than 30 μm. This was expected because the number of cells would increase with the channel cross-section. In other words, when the migration distance in each channel is normalized to cross-sectional area, the migration speed may be independent of channel width [Citation60]. Therefore, the effect of channel height on cell migration distance was also observed using PDMS channels 350 μm wide and 80, 200 or 400 μm tall (figure (c)). Faster migration was observed in the taller channels, at constant cross-sectional area. There results indicate that channel with larger diameters can provide not only a larger surface area for cell adherence, but also a more efficient supply of oxygen and nutrients, which is important to maintain cell viability.
Figure 6 (a) Schematic of experimental setup for cell migration assay. Transparent PDMS microchannels enable the microscopic observation of Schwann cells migration in the channel. We measured the migration distances of Schwann cells on the poly-L-lysine-coated TCPS, which was covered with PDMS channels of various widths (b) and heights (c).
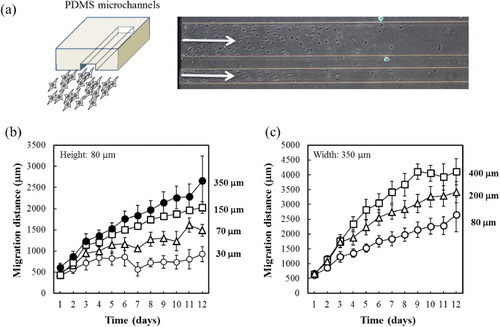
From the reported results, we have developed a concept of scaffold design for peripheral nerve regeneration as shown in figure . Although the surface area available for cell adhesion dramatically increases as the channel size decrease (solid blue line in figure ), no significant migration of SCs was observed for the channel with a width of 30 μm and a height of 80 μm (see figure (b)). This is in part due to the volume decrease for individual channels, which is important for supplying oxygen and nutrients to cells (solid red line). This trend agrees with the general notion that when cells are adhering and growing under a limited supply of oxygen/nutrients, their viability should be reduced. In this study, we used PDMS because of its transparency and capability of microfabrication. PDMS is also known as the most permeable rubbery polymer for oxygen. This can be explained by its chain flexibility, rotational mobility, large free volume and low glass transition temperature [Citation61]. Because the crosslinked P(CL-co-DLLA) scaffolds also exhibited similar characteristics to PDMS in terms of flexibility, crystallinity and hydrophobicity [Citation35, Citation39, Citation62], we expect that the observed trend in PDMS channels can be applied to the crosslinked P(CL-co-DLLA) scaffolds. We also demonstrate that mechanical properties of the scaffold can be controlled by selective sizing of the channel dimensions. The scaffold with larger channel diameter broke at lower stress (dashed black line). From our results, 350 μm is the optimal channel size, which approximates the microarchitecture of native peripheral nerves. Additionally, mechanical tests revealed excellent elasticity and pliability of the crosslinked P(CL-co-DLLA) scaffolds at 37 °C, and the channel structure was maintained when the scaffolds were deformed. These results may help designing scaffolds for peripheral nerve regeneration.
4. Conclusions
We prepared super-elastic biodegradable scaffolds with longitudinal microchannels by crosslinking end-functionalized four-armed P(CL-co-DLLA)s. The elastic modulus was controlled (<1 MPa) by adjusting Tm to the body temperature. Well-defined microchannels consistent with the mold design were produced in the scaffolds using a silica fiber templating technique. The produced microchannels did not collapse upon bending due to the elastic nature of scaffolds. The effect of individual channel diameter on mechanical properties was also evaluated. Scaffolds with wider channels (approximately 600 μm) broke at lower stress. On the other hand, SC migration was faster in wider channels because they provided more oxygen and nutrients to the cells. From these results, 350 μm is the optimal channel width, which also approximates the microarchitecture of native peripheral nerves.
Acknowledgments
This research was partially supported by the Japan Society for the Promotion of Science (JSPS) through the ‘Funding Program for World-Leading Innovative R&D on Science and Technology (FIRST Program),’ initiated by the Council for Science and Technology Policy (CSTP).
References
- LeeS KWolfeS W 2000 J. Am. Acad. Orthop. Surg. 8 243
- BattistonBGeunaSFerreroMTosP 2005 Microsurgery 25 258 10.1002/micr.20127
- BelkasJ SMunroC AShoichetM SMidhaR 2005 Restor. Neurol. Neurosci. 23 19
- PegoA PVleggeert-LankampC LDeenenMLakkeE AGrijpmaD WPootA AMaraniEFeijenJ 2003 J. Biomed. Mater. Res. A 67 876 10.1002/jbm.a.10074
- RodriguezF JVerduECeballosDNavarroX 2000 Exp. Neurol. 161 571 10.1006/exnr.1999.7315
- HuangY-CHuangY-Y 2006 Biomed. Eng.: Appl. Basis Commun. 18 100 10.4015/S101623720600018X
- GuXDingFYangYLiuJ 2011 Prog. Neurobiol. 93 204 10.1016/j.pneurobio.2010.11.002
- RaimondoSFornaroMTosPBattistonBGiacobini-RobecchiM GGeunaS 2011 Ann. Anat. 193 334 10.1016/j.aanat.2011.03.001
- LangerRVacantiJ P 1993 Science 260 920 10.1126/science.8493529
- SangsanohPWaleetorncheepsawatSSuwantongOWutticharoenmongkolPWeeranantanapanOChuenjitbuntawornBCheepsunthornPPavasantPSupapholP 2007 Biomacromolecules 8 1587 10.1021/bm061152a
- TobaTNakamuraTShimizuYMatsumotoKOhnishiKFukudaSYoshitaniMUedaHHoriYEndoK 2001 J. Biomed. Mater. Res. 58 622 10.1002/jbm.1061
- TobaTNakamuraTLynnA KMatsumotoKFukudaSYoshitaniMHoriYShimizuY 2002 Int. J. Artif. Organs 25 230
- MatsumotoKOhnishiKKiyotaniTSekineTUedaHNakamuraTEndoKShimizuY 2000 Brain Res. 868 315 10.1016/S0006-8993(00)02207-1
- IchiharaSInadaYNakadaAEndoKAzumaTNakaiRTsutsumiSKurosawaHNakamuraT 2009 Tissue Eng. C: Methods 15 387 10.1089/ten.tec.2008.0508
- GrijpmaD WZondervanG JPenningsA J 1991 Polym. Bull. 25 327 10.1007/BF00316902
- Den DunnenW F ASchakenraadJ MZondervanG JPenningsA JVanderleiBRobinsonP H 1993 J. Mater. Sci.: Mater. Med. 4 521 10.1007/BF00120133
- Den DunnenW F AVanderleiBSchakenraadJ MBlaauwE HStokroosIPenningsA JRobinsonP H 1993 Microsurgery 14 508 10.1002/micr.1920140808
- MuroyaTYamamotoKAoyagiT 2009 Polym. Degrad. Stab. 94 285 10.1016/j.polymdegradstab.2008.12.014
- MeekM FJansenKSteendamRvan OeverenWvan WachemP Bvan LuynM J A 2004 J. Biomed. Mater. Res. Part A 68 43 10.1002/jbm.a.10157
- Den DunnenW Fvan der LeiBRobinsonP HHolwerdaAPenningsA JSchakenraadJ M 1995 J. Biomed. Mater. Res. 29 757 10.1002/jbm.820290612
- Den DunnenW FStokroosIBlaauwE HHolwerdaAPenningsA JRobinsonP HSchakenraadJ M 1996 J. Biomed. Mater. Res. 31 105 10.1002/(SICI)1097-4636(199605)31:1%3C105::AID-JBM13%3E3.0.CO;2-M
- Den DunnenW F AVanderLeiBSchakenraadJ MStokroosIBlaauwEBartelsHPenningsA JRobinsonP H 1996 Microsurgery 17 348 10.1002/(SICI)1098-2752(1996)17:7%3C348::AID-MICR2%3E3.0.CO;2-C
- MeekM FVan der WerffJ F ANicolaiJ P AGramsbergenA 2001 Muscle Nerve 24 753 10.1002/mus.1066
- MackinnonS EDellonA L 1990 Plast. Reconstr. Surg. 85 419 10.1097/00006534-199003000-00015
- VacantiC ABonassarL JVacantiM PShufflebargerJ 2001 New Engl. J. Med. 344 1511 10.1056/NEJM200105173442004
- MosahebiAWoodwardBWibergMMartinRTerenghiG 2001 Glia 34 8 10.1002/glia.1035
- NathanielE J 1963 J. Ultrastruct. Res. 52 511 10.1016/S0022-5320(63)80082-9
- ThomasP K 1964 J. Anat. 98 175
- GianniniCDyckP J 1990 J. Neuropathol. Exp. Neurol. 49 550 10.1097/00005072-199011000-00002
- BradleyJ LAbernethyD AKingR HMuddleJ RThomasP K 1998 J. Anat. 192 (Pt 4) 529 10.1046/j.1469-7580.1998.19240529.x
- HokeA 2006 Nat. Clin. Pract. Neurol. 2 448 10.1038/ncpneuro0262
- HadlockT ASundbackC AHunterD AVacantiJ PCheneyM L 2001 Microsurgery 21 96 10.1002/micr.1016
- MiyasakoHYamamotoKAoyagiK 2008 Polym. J. 40 806 10.1295/polymj.PJ2008036
- MiyasakoHYamamotoKNakaoAAoyagiT 2007 Macromol. Biosci. 7 76 10.1002/mabi.200600188
- AoyagiTMiyataFNagaseY 1994 J. Control. Release 32 87 10.1016/0168-3659(94)90228-3
- EbaraMHoffmanJ MHoffmanA SStaytonP S 2006 Lab Chip 6 843 10.1039/b515128g
- HoffmanJ MEbaraMLaiJ JHoffmanA SFolchAStaytonP S 2010 Lab Chip 10 3130 10.1039/c004978f
- LendleinASchmidtA MLangerR 2001 Proc. Natl Acad. Sci. USA 98 842 10.1073/pnas.98.3.842
- UtoKYamamotoKHiraseSAoyagiT 2006 J. Control. Release 110 408 10.1016/j.jconrel.2005.10.024
- EbaraMUtoKIdotaNHoffmanJ MAoyagiT 2012 Adv. Mater. 24 273 10.1002/adma.201102181
- NijenhuisA JGrijpmaD WPenningsA J 1996 Polymer 37 2783 10.1016/0032-3861(96)87642-7
- HelminenA OKorhonenHSeppalaJ V 2002 Macromol. Chem. Phys. 203 2630 10.1002/macp.200290039
- LimJ IKimJ HParkH-K 2012 Microelectron. Eng. 96 6 10.1016/j.mee.2012.02.046
- EvansG R DBrandtKKatzSChauvinPOttoLBogleMWangBMeszlenyiR KLuL CMikosA GPatrickC W 2002 Biomaterials 23 841 10.1016/S0142-9612(01)00190-9
- YangFMuruganRRamakrishnaSWangXMaY XWangS 2004 Biomaterials 25 1891 10.1016/j.biomaterials.2003.08.062
- ValmikinathanC MHoffmanJYuX 2011 Mater. Sci. Eng. C: Mater. Biol. Appl. 31 22 10.1016/j.msec.2010.04.001
- BellamkondaR V 2006 Biomaterials 27 3515 10.1016/j.biomaterials.2006.02.030
- LietzMDreesmannLHossMOberhoffnerSSchlosshauerB 2006 Biomaterials 27 1425 10.1016/j.biomaterials.2005.08.007
- KimY THaftelV KKumarSBellamkondaR V 2008 Biomaterials 29 3117 10.1016/j.biomaterials.2008.03.042
- HuXHuangJYeZXiaLLiMLvBShenXLuoZ 2009 Tissue Eng. A 15 3297 10.1089/ten.tea.2009.0017
- WangAAoQCaoWYuMHeQKongLZhangLGongYZhangX 2006 J. Biomed. Mater. Res. A 79 36 10.1002/jbm.a.30683
- HuangY CHuangY YHuangC CLiuH C 2005 J. Biomed. Mater. Res. B Appl. Biomater 74 659 10.1002/jbm.b.30267
- FlynnLDaltonP DShoichetM S 2003 Biomaterials 24 4265 10.1016/S0142-9612(03)00334-X
- WenC EYamadaYShimojimaKChinoYHosokawaHMabuchiM 2004 Mater. Lett. 58 357 10.1016/S0167-577X(03)00500-7
- MiyoshiTItohMAkiyamaSKitaharaA 2000 Adv. Eng. Mater. 2 179 10.1002/(SICI)1527-2648(200004)2:4%3C179::AID-ADEM179%3E3.0.CO;2-G
- AoQWangA JCaoW LZhaoCGongY DZhaoN MZhangX F 2005 Key Eng. Mater. 288–289 27 10.4028/www.scientific.net/KEM.288-289.27
- ZhuY QWangA JPatelSKurpinskiKDiaoEBaoXKwongGYoungW LLiS 2011 Tissue Eng. C: Med. 17 705 10.1089/ten.tec.2010.0565
- TsurugaETakitaHItohHWakisakaYKubokiY 1997 J. Biochem. 121 317 10.1093/oxfordjournals.jbchem.a021589
- ByrneE MFarrellEMcMahonL AHaughM GO'BrienF JCampbellV APrendergastP JO'ConnellB C 2008 J. Mater. Sci.: Mater. Med. 19 3455 10.1007/s10856-008-3506-2
- PearsonR GMolinoYWilliamsP MTendlerS J BDaviesM CRobertsC JShakesheffK M 2003 Tissue Eng. 9 201 10.1089/107632703764664675
- PolmanteerK E 1981 Rubber Chem. Technol. 54 1051 10.5254/1.3535846
- AndradyA LSefcikM D 1984 J. Appl. Polym. Sci. 29 3561 10.1002/app.1984.070291129